Cardiovascular System
This chapter follows the development of the heart from a simple tubular structure to the four-chambered organ that can assume the full burden of maintaining an independent circulation at birth. Similarly, the pattern of blood vessels is traced from their first appearance to an integrated system that carries blood to all parts of the embryo and the placenta. (The early stages in the establishment of the heart and blood vessels are described in Chapter 6 [see Figs. 6.14 to 6.19], and the general plan of the embryonic circulation is summarized in Figure 6.26.) Cellular aspects of blood formation are also briefly described. Clinical Correlations 17.1 and 17.2 at the end of the chapter discuss malformations of the heart and the blood vessels. Table 17.6, also at the end of the chapter, summarizes the timelines in cardiac development.
Development of Blood and the Vascular System
Development of the vascular system begins in the wall of the yolk sac during the third week of gestation (18 days) with the formation of blood islands (see Fig. 6.19). At this time, the embryo has attained a size that is too large for the distribution of oxygen to all tissues by diffusion alone. This situation necessitates the very early development of the heart and the vascular system. Because the tissues that normally produce blood cells in an adult have not yet begun to form, yolk sac hematopoiesis serves as a temporary adaptation for accommodating the immediate needs of the embryo.
Embryonic Hematopoiesis
Hemangiogenic precursor cells first arise in the posterolateral mesoderm during gastrulation and from there migrate to the earliest blood-forming organs (Fig. 17.1). Under the influence of Runx-1, some of their progeny follow the hematopoietic lineage, whereas others, responding to Hoxa3, enter the endothelial lineage. Still other progeny will enter a third lineage and eventually form vascular smooth muscle cells. Although blood cell formation (hematopoiesis) begins in the yolk sac, the yolk sac–derived cells are soon replaced by blood cells that are independently derived from other sites of hematopoiesis (Fig. 17.2).
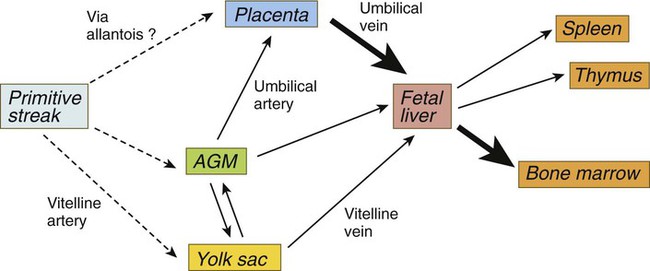
AGM, aorta/genital ridge/mesonephros region. (Adapted from Mikkola HKA, Orkin SH: Development 133:3733-3744, 2006.)
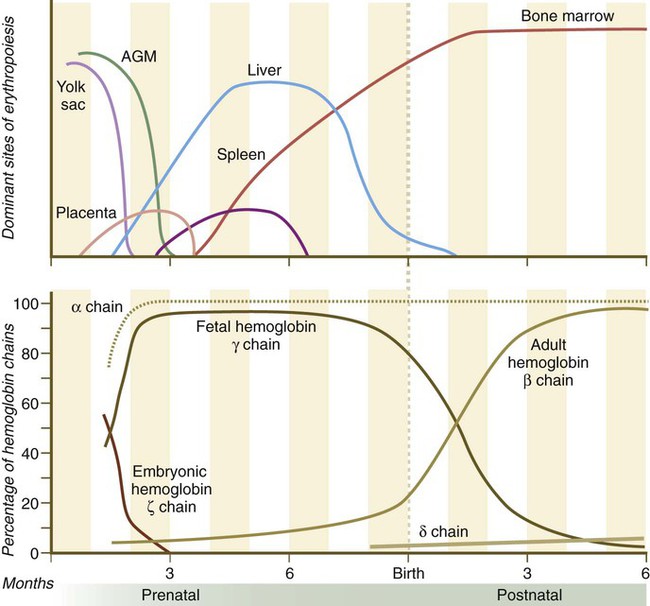
The upper graph highlights the relative importance of the various sites of hematopoiesis. The lower graph shows the percentages of the various hemoglobin polypeptide chains present in the blood at any given time. The α chain is treated separately from the others. AGM, aorta/genital ridge/mesonephros region. (Based on Carlson B: Patten’s foundations of embryology, ed 6, New York, 1996, McGraw-Hill).
Analysis of human embryos has shown that, starting at 28 days, definitive intraembryonic hematopoiesis begins in small clusters of cells (para-aortic clusters) in the splanchnopleuric mesoderm associated with the ventral wall of the dorsal aorta and shortly thereafter in the aorta/genital ridge/mesonephros (AGM) region. Precursor cells from the AGM region make their way via the blood to blood-forming sites in the liver, the yolk sac, and the placenta. Hematopoietic stem cells formed in the AGM, the yolk sac, and the placenta become transported to the liver via the circulation to the liver (see Fig. 17.1). By 5 to 6 weeks of gestation, sites of hematopoiesis become prominent in the liver. In both the yolk sac and the early sites of embryonic hematopoiesis, the endothelial cells themselves briefly retain the capacity for producing blood-forming cells. There is now evidence that in the AGM region, nitric oxide gas signaling, resulting from shear stress caused by blood flow on the endothelial cells, can induce their transformation into hematopoietic stem cells.
Cellular Aspects of Hematopoiesis
The first hematopoietic stem cells that arise in the embryo are truly pluripotential in that they can give rise to all the cell types found in the blood (Fig. 17.3). These pluripotent stem cells, sometimes called hemocytoblasts, have great proliferative ability. They produce vast numbers of progeny, most of which are cells at the next stage of differentiation, but they also produce small numbers of their original stem cell type, which act as a reserve capable of replenishing individual lines of cells should the need arise. Very early in development, the line of active blood-forming cells subdivides into two separate lineages. Lymphoid stem cells ultimately form the two lines of lymphocytes: B lymphocytes (which are responsible for antibody production) and T lymphocytes (which are responsible for cellular immune reactions). Myeloid stem cells are precursors to the other lines of blood cells: erythrocytes, the granulocytes (neutrophils, eosinophils, and basophils), monocytes, and platelets. The second-generation stem cells (lymphoid and myeloid) are still pluripotent, although their developmental potency is restricted because neither lymphoid cells nor myeloid cells can form the progeny of the other type.
Erythropoiesis
The erythrocyte lineage represents one line of descent from the CFU-S cells. Although the erythroid progenitor cells are restricted to forming only red blood cells, there are many generations of precursor cells (Fig. 17.4). The earliest stages of erythropoiesis are recognized by the behavior of the precursor cells in culture, rather than by morphological or biochemical differences. These are called erythroid burst-forming units (BFU-E) and erythroid CFUs (CFU-E). Each responds to different stimulatory factors. The pluripotent CFU-S precursors (see Fig. 17.3) respond to interleukin-3, a product of macrophages in adult bone marrow. A hormone designated as burst-promoting activity stimulates mitosis of the BFU-E precursors (see Fig. 17.4). A CFU-E cell, which has a lesser proliferative capacity than a BFU-E cell, requires the presence of erythropoietin as a stimulatory factor.
One or two generations after the CFU-E stage, successive generations of erythrocyte precursor cells can be recognized by their morphology. The first recognizable stage is the proerythroblast (Fig. 17.5), a large, highly basophilic cell that has not yet produced sufficient hemoglobin to be detected by cytochemical analysis. Such a cell has a large nucleolus, much uncondensed nuclear chromatin, numerous ribosomes, and a high concentration of globin mRNAs. These are classic cytological characteristics of an undifferentiated cell.
Hemoglobin Synthesis and its Control
Both the red blood cells and the hemoglobin within them undergo isoform transitions during embryonic development. The adult hemoglobin molecule is a complex composed of heme and four globin chains: two α and two β chains. The α and β subunits are products of genes located on chromosomes 16 and 11 (Fig. 17.6). Different isoforms of the subunits are encoded linearly on these chromosomes.
During the period of yolk sac hematopoiesis, embryonic globin isoforms are produced. The earliest embryonic hemoglobin, sometimes called Gower 1, is composed of two ζ (α-type) and two ε (β-type) chains. After passing through a couple of transitional forms (Table 17.1), hemoglobin synthesis enters a fetal stage by 12 weeks, which corresponds to the shift in the site of erythropoiesis from the yolk sac to the liver. Fetal hemoglobin consists of two adult-type α chains, which form very early in embryogenesis, and two γ chains, the major fetal isoform of the β chain. Fetal hemoglobin is the predominant form during the remainder of pregnancy. The main adaptive value of the fetal isoform of hemoglobin is that it has a higher affinity for oxygen than the adult form. This is advantageous to the fetus, which depends on the oxygen concentration of the maternal blood. Starting at about 30 weeks’ gestation, there is a gradual switch from the fetal to the adult type of hemoglobin, with α2β2 being the predominant type. A minor but functionally similar variant is α2δ2.
Table 17.1
Developmental Isoforms of Human Hemoglobin
Developmental Stage | Hemoglobin Type | Globin Chain Composition |
Embryo | Gower 1 | ζ2ε2 |
Embryo | Gower 2 | α2ε2 |
Embryo | Portland | ζ2γ2 |
Embryo to fetus | Fetal | α2γ2 |
Fetus to adult | A (adult) | α2β2 |
Adult | A2 | α2δ2 |
Adult | Fetal | α2γ2* |
*The fetal hemoglobin expressed in adults differs from true fetal hemoglobin by an amino acid substitution at the 136 position of the γ chain.
Adapted from Brown MS: In Stockman J, Pochedly C, eds: Developmental and neonatal hematology, New York, 1988, Raven Press.
Formation of Embryonic Blood Vessels
The early embryo is devoid of blood vessels. Although blood islands appear in the wall of the yolk sac, and extraembryonic vascular channels form in association with them (see Fig. 6.19), much of the vasculature of the embryonic body is derived from intraembryonic sources. During the early period of somite formation, networks of small vessels rapidly appear in many regions of the embryonic body.
The formation of blood vessels in the embryo consists of several phases (Fig. 17.7). The first is the specification of a population of vascular precursors, called angioblasts. These cells become organized into a primary capillary plexus through a process known as vasculogenesis. To keep pace with the rapidly growing embryo, the primary capillary plexus must rapidly undergo reorganization through the resorption of existing vessels and the sprouting of new branches to support the expanding vascular network. This latter process is called angiogenesis. Angiogenesis continues not only in the prenatal period, but also throughout adult life, as tissues and organs continually adapt to changing conditions of life, whether normal or pathological.
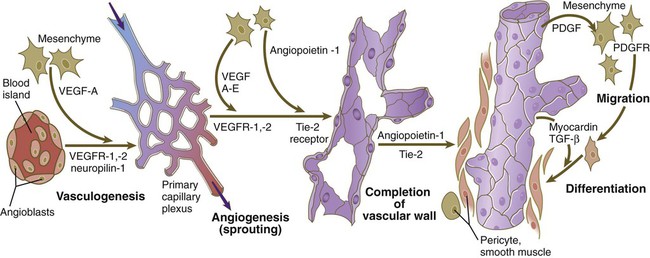
Angioblasts, initially expressing vascular endothelial growth factor receptor (VEGFR-2), are stimulated by vascular endothelial growth factor (VEGF-A), secreted by the surrounding mesenchyme, to form the primary capillary plexus by the process of vasculogenesis. Under additional stimulation by growth factors, competent endothelial cells of the primary capillary plexus form vascular sprouts in the earliest stages of angiogenesis. This is followed by the recruitment of surrounding mesenchymal cells to form the cellular elements of the vascular wall. PDGF, platelet-derived growth factor; PDGFR, platelet-derived growth factor receptor; TGF-β, transforming growth factor-β.
Detailed descriptive studies and transplantation experiments involving intrinsic cellular labels or graft-specific monoclonal antibody labels have shown that angioblasts arise from most mesodermal tissues of the body, except notochord and prechordal mesoderm (Table 17.2). Embryonic blood vessels form from angioblasts by three main mechanisms. Many of the larger blood vessels, such as the dorsal aortae, are formed by the coalescence of angioblasts in situ. Other equally large channels, such as the endocardium, are formed by angioblasts migrating into the region from other sites. Other vessels, especially the intersegmental vessels of the main body axis and vessels of the central nervous system, arise as vascular sprouts from existing larger vessels. Many of the angioblasts of the trunk are originally associated with the splanchnic mesoderm.
Table 17.2
Distribution of Endogenous Angioblasts in Embryonic Tissues
Tissues | Angioblasts |
Cephalic | |
Paraxial mesoderm | + |
Lateral mesoderm | + |
Prechordal mesoderm | − |
Notochord | − |
Brain | − |
Neural crest | − |
Trunk | |
Whole somites | + |
Dorsal half somites | + |
Segmental plate mesoderm | + |
Lateral somatic mesoderm | + |
Lateral splanchnic mesoderm | + |
Spinal cord | − |
From Noden DM: Ann NY Acad Sci 588:236-249, 1990.
All stages in the formation of the vascular system occur in response to the influence of powerful growth factors and their receptors. The initial phase of recruitment of a population of angioblasts from the mesoderm is characterized by the appearance of a transmembrane vascular endothelial growth factor receptor (VEGFR-2) on their surfaces (see Fig. 17.7). Soon, in response to the production of vascular endothelial growth factor (VEGF-A) by the surrounding mesenchyme, the phase of vasculogenesis occurs, and the angioblasts form the cellular tubes that become the basis for the primary capillary plexus.
Research has shed considerable light on the differentiation of the arterial versus venous system. The arterial or venous identity of endothelial cells is established very early in their development, before angiogenesis and before the onset of circulation. The endothelial cells of developing arteries express the membrane-bound ligand Ephrin-B2, whereas the endothelial cells of developing veins express the receptor Eph-B4 on their surface membranes. These characteristic phenotypes are the results of different signaling cascades. Arterial vessels are the first to differentiate from generic endothelial background, and a set of signals, starting with sonic hedgehog (shh), finally leads to the acquisition of an arterial phenotype (Fig. 17.8). Notch, one of the links in that cascade, not only causes progression of the sequence of arterial differentiation leading to Ephrin-B2 expression, but it inhibits the expression of Eph-4 and the pathway leading to the venous phenotype. In what was earlier assumed to be a purely default mode, venous differentiation occurs under the influence of COUP-TFII (Chicken ovalbumin upstream promoter-transcription factor II), which suppresses the arterial pathway by inhibiting Notch signaling, but is a determinant of venous differentiation by acting upstream of Eph-4. Under the influence of Sox-18 and Prox-1, lymphatic vessels form and branch off from the veins. Prox-1 is a master regulator of venous identity. Later, physiological and local factors play a role in the differentiation of blood vessels. When the flow of blood to the yolk sac is greatly reduced, vessels slated to become arteries develop venous characteristics; correspondingly, developing veins exposed to high blood pressure transform into arteries.
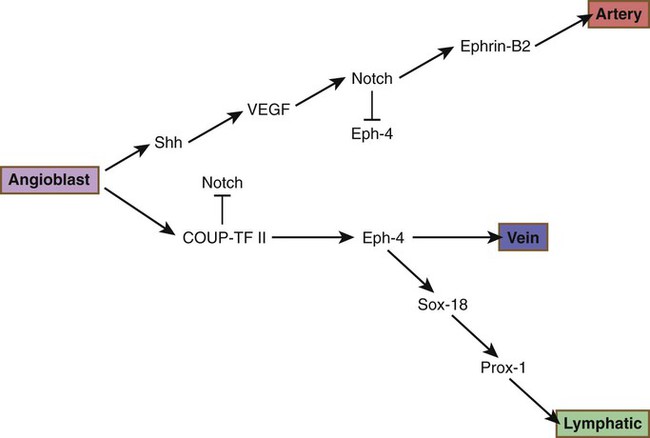
COUP-TF II, chicken ovalbumin upstream promoter-transcription factor II; Shh, sonic hedgehog; VEGF, vascular endothelial growth factor.
As with myoblasts, angioblasts seem to react to local environmental cues that determine the specific morphological pattern of a blood vessel. An unexpected finding is that the pattern of the peripheral innervation often determines the pattern of the smaller arteries. The growing tips of endothelial cell buds and axonal growth cones of outgrowing nerve fibers contain receptors that respond remarkably similarly to the major families of environmental ligands (Table 17.3). VEGF, secreted by the nerve fibers, acts as an effective patterning agent for the blood vessels. The smooth muscle cells of the developing arteries secrete a factor, artemin, that guides the extension of sympathetic nerve fibers along the vessel wall.
Table 17.3
Responsiveness of Axonal Growth Cones and Endothelial Cell Tips to Environmental Ligands
Ligand | Growth Cones | Endothelial Tips |
Attractants | ||
Semaphorin | + | − |
VEGF | − | + |
Netrin | + | + |
Slit | + | + |
Repellants | ||
Semaphorin | + | + |
Ephrin | + | + |
Netrin | + | + |
Slit | + | + |
Development of the Arteries
Aorta, Aortic Arches, and Their Derivatives
The dorsal aorta forms from the direct aggregation of endothelial precursor cells derived from the lateral plate mesoderm. These cells form a vessel directly by a vasculogenesis mechanism. Vasculogenesis is stimulated by VEGF and other factors produced by the endoderm and BMP in the lateral mesoderm. When first formed, the cranial part of the dorsal aorta is a paired, with each member located lateral to the midline. The reason is that in the midline the notochord secretes the BMP antagonists noggin and chordin, which inhibit the activity of BMP and also inactivate the vasculogenic influences from the endoderm. Late in the fourth week, hematopoietic stem cells form in the lining of the ventral part of the aorta (see p. 409).
The system of aortic arches in early human embryos is organized along the same principles as the system of arteries supplying blood to the gills of many aquatic lower vertebrates. Blood exits from a common ventricle in the heart into a ventral aortic root, from which it is distributed through the branchial arches by pairs of aortic arches (Fig. 17.9A). In gilled vertebrates, the aortic arch arteries branch into capillary beds, where the blood becomes reoxygenated as it passes through the gills. In mammalian embryos, the aortic arches remain continuous vessels because gas exchange occurs in the placenta and not in the pharyngeal arches. The aortic arches empty into paired dorsal aortae where the blood enters the regular systemic circulation. In human embryos, all aortic arches are never present at the same time. Their formation and remodeling show a pronounced craniocaudal gradient. Blood from the outflow tract of the heart (the truncoconal region) flows into an aortic sac, which differs from the truncoconal region in the construction of its wall. The aortic arches branch off from the aortic sac.
The developmental anatomy of the aortic arch system illustrates well the principle of morphological adaptation of the vascular bed during different stages of embryogenesis (Table 17.4). Continued development of the cranial and cervical regions causes components of the first three arches and associated aortic roots to be remodeled into the carotid artery system (see Fig. 17.9). With the remodeling of the heart tube and the internal division of the outflow tract into aortic and pulmonary components, the fourth arches undergo an asymmetrical adaptation to the early asymmetry of the heart. The left fourth aortic arch is retained as a major channel (arch of the aorta), which carries the entire output from the left ventricle of the heart. The right fourth arch is incorporated into the right subclavian artery.
Table 17.4
Adult Derivatives of the Aortic Arch System
Right Side | Left Side | |
Aortic Arches | ||
1 | Disappearance of most of structure | Disappearance of most of structure |
Part of maxillary artery | Part of maxillary artery | |
2 | Disappearance of most of structure | Disappearance of most of structure |
Hyoid and stapedial arteries | Hyoid and stapedial arteries | |
3 | Ventral part—common carotid artery | Ventral part—common carotid artery |
Dorsal part—internal carotid artery | Dorsal part—internal carotid artery | |
4 | Proximal part of right subclavian artery | Part of arch of aorta |
5 | Rarely recognizable, even in early embryo | Rarely recognizable, even in early embryo |
6 (pulmonary) | Part of right pulmonary artery | Ductus arteriosus |
Part of left pulmonary artery | ||
Ventral Aortic Roots | ||
Cranial to third arch | External carotid artery | External carotid artery |
Between third and fourth arches | Common carotid artery | Common carotid artery |
Between fourth and sixth arches | Right brachiocephalic artery | Ascending part of aorta |
Dorsal Aortic Roots | ||
Cranial to third arch | Internal carotid artery | Internal carotid artery |
Between third and fourth arches | Disappearance of structure | Disappearance of structure |
Between fourth and pulmonary arches | Central part of right subclavian artery | Descending aorta |
Caudal to pulmonary arch | Disappearance of structure | Descending aorta |
Embryology textbooks traditionally depict the aortic arch system as consisting of six pairs of vascular arches, but the fifth and sixth arches never appear as discrete vascular channels similar to the first through fourth arches. The fifth aortic arch, if it exists at all, is represented by no more than a few capillary loops. The sixth (pulmonary arch) arises as a capillary plexus associated with the early trachea and lung buds. The capillary plexus is supplied by ventral segmental arteries arising from the paired dorsal aortae in that region (Fig. 17.10). The equivalent of the sixth arch is represented by a discrete distal segment (ventral segmental artery) connected to the dorsal aorta and a plexuslike proximal segment that establishes a connection between the aortic sac at the base of the fourth arch and the distal segmental component. As the respiratory diverticulum and early lung buds elongate, parts of the pulmonary capillary network consolidate to form a pair of discrete pulmonary arteries that connect to the putative sixth arch. Although the term sixth aortic arch is frequently used in anatomical and clinical literature, pulmonary arch is a more appropriate term because it does not imply equivalence to the other aortic arches.
Similar to the fourth aortic arch, the pulmonary arch develops asymmetrically. On the left side, it becomes a large channel. Its distal segment, which was derived from a ventral segmental artery, persists as a major channel (ductus arteriosus) that shunts blood from the left pulmonary artery to the aorta (see Fig. 17.9C). The lungs are protected by this shunt from a flow of blood that is greater than what their vasculature can handle during most of the intrauterine period. On the right side, the distal segment of the pulmonary arch regresses, and the proximal segment (the base of the right pulmonary artery) branches off from the pulmonary trunk.
The asymmetry of the derivatives of the pulmonary arch accounts for the difference between the course of the right and left recurrent laryngeal nerves, which are branches of the vagus nerve (cranial nerve X). These nerves, which supply the larynx, hook around the pulmonary arches. As the heart descends into the thoracic cavity from the cervical region, the branch point from the vagus of each recurrent laryngeal nerve is correspondingly moved. On the left side, the nerve is associated with the ductus arteriosus (see Fig. 17.9C), which persists throughout the fetal period, so it is pulled deep into the thoracic cavity. On the right side, with the regression of much of the right pulmonary arch, the nerve moves to the level of the fourth arch, which constitutes an anatomical barrier. The positions of the right and left recurrent laryngeal nerves in an adult reflect this asymmetry, with the right nerve curving under the right subclavian artery (fourth arch) and the left nerve hooking around the ligamentum arteriosum (the adult derivative of the ductus arteriosus, the distal segment of the left pulmonary arch).
Major Branches of the Aorta
In the early embryo, when the dorsal aortae are still paired vessels, three sets of arterial branches arise from them—dorsal intersegmental, lateral segmental, and ventral segmental (Fig. 17.11). These branches undergo a variety of modifications in form before assuming their adult configurations (Table 17.5). The ventral segmental arteries arise as paired vessels that course over the dorsal and lateral walls of the gut and yolk sac. With the closure of the gut and the narrowing of the dorsal mesentery, certain branches fuse in the midline to form the celiac, superior, and inferior mesenteric arteries.
Table 17.5
Major Arterial Branches of the Aorta
Embryonic Vessels | Adult Derivatives |
Dorsal Intersegmental Branches (Paired) | |
Cervical intersegmental (1-16) | Lateral branches joining to become vertebral arteries |
Seventh intersegmentals | Subclavian arteries |
Thoracic intersegmentals | Intercostal arteries |
Lumbar intersegmentals | Iliac arteries |
Lateral Segmental Branches | |
Up to 20 pairs of vessels supplying the mesonephros | Adrenal arteries, renal arteries, gonadal (ovarian or spermatic) arteries |
Ventral Segmental Branches* | |
Vitelline vessels | Celiac artery, superior and inferior mesenteric arteries |
Allantoic vessels | Umbilical arteries |
*Originally paired in areas where the embryonic aorta itself consists of paired components.
Arteries of the Head
The arteries supplying the head arise from two sources. Ventrally, the aortic arch system (first to third arches and corresponding roots) gives rise to the arteries supplying the face (external carotid arteries) and the frontal part of the base of the brain (internal carotid arteries) (see Fig. 17.9).
At the level of the spinal cord, the vertebral arteries, which form through connections of lateral branches of dorsal intersegmental arteries, grow toward the brain. Soon, they veer toward the midline and fuse with the basilar artery, which has already formed earlier from the fusion of the bilateral longitudinal neural arteries (Fig. 17.12). The longitudinal neural arteries, which run along the ventral sides of the brain from the midbrain through the caudal end of the hindbrain, represent the major arterial supply to the brain during the fourth week of development. The basilar artery runs along the ventral surface of the brainstem and supplies the brainstem with a series of paired arteries. As the basilar artery approaches the level of the diencephalon and the internal carotid arteries, sets of branches from each of these major vessels grow out and fuse, to form posterior communicating arteries, which join the circulations of the basilar and internal carotid arteries. Two other small branches off the internal carotid system fuse in the midline to complete a vascular ring (circle of Willis), which underlies the base of the diencephalon and encircles the optic chiasm and pituitary stalk. The circle of Willis is a structural adaptation that ensures a continuous blood supply in the event of occlusion of some major arteries supplying the brain. In addition, it is a structural landmark that marks the transition between mesodermally derived blood vessels of the trunk and those of the head, which are primarily of neural crest origin.
Coronary Arteries
Although intuitively one would expect the coronary arteries to arise as branches growing out from the aorta, experimental studies have shown instead that cellular precursors of the coronary arteries, arising in the same cellular primordium as the future epicardium, migrate toward the aorta and invade its wall (see Fig. 6.18B). The smooth muscle cells of the coronary vessels have a purely mesodermal origin, instead of the mixed neural crest and mesodermal origin seen in the aortic arch derivatives. Studies involving the tagging of cells with retroviral markers confirm that progenitor cells of the coronary vessels penetrate the wall of the already beating heart as the epicardial layer envelops the myocardium, and that after forming in situ, the coronary arteries secondarily enter the aorta.
Development of Veins
Veins follow a morphologically complex pattern of development characterized by the formation of highly irregular networks of capillaries and the ultimate expansion of certain channels into definitive veins. Because of the multichanneled beginnings and the number of options (Fig. 17.13), the adult venous system is characterized by a higher incidence of anatomical variations than the arterial system. A detailed description of the development of venous channels is beyond the scope of this text.
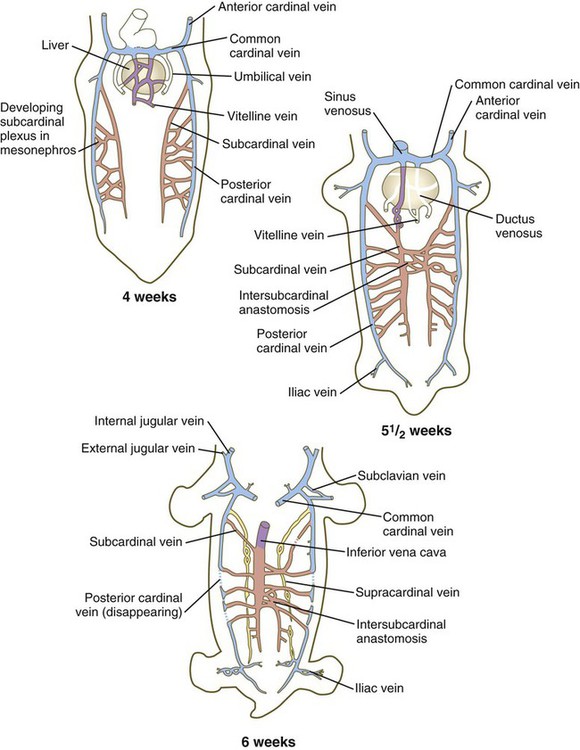
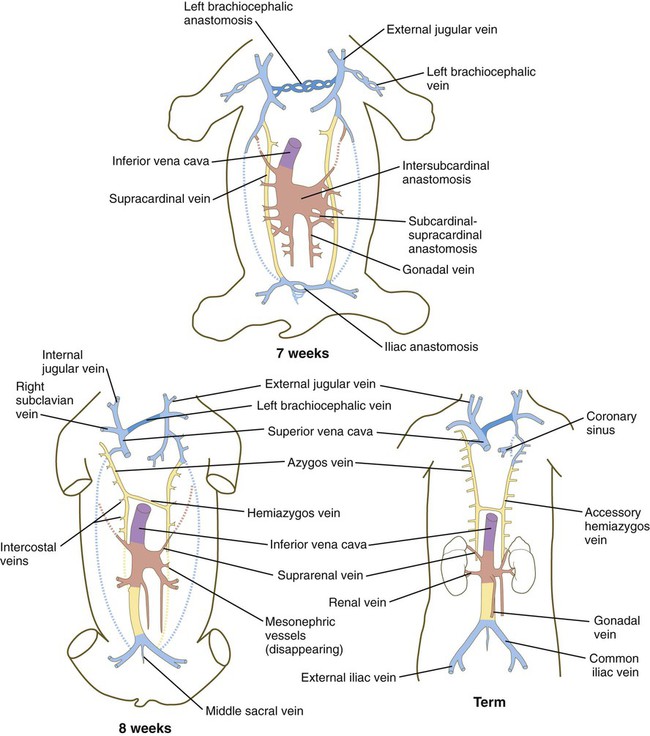
The colors of the original embryonic cardinal veins are carried through in all drawings to facilitate an understanding of the derivations of the adult veins. (Based on McClure CFW, Butler EG: Am J Anat 35:331-383, 1925.)
Cardinal Veins
The earliest pattern of cardinal veins consists of paired anterior and posterior cardinal veins, which drain blood from the head and body into a pair of short common cardinal veins (see Fig. 17.13). The common cardinal veins, in turn, empty their blood into the sinus venosus of the primitive heart (see Fig. 17.15).
In the cranial region, the originally symmetrical anterior cardinal veins are transformed into the internal jugular veins (Fig. 17.14). As the heart rotates to the right, the base of the left internal jugular vein is attenuated. At the same time, a new anastomotic channel, which ultimately forms the left brachiocephalic vein, connects the left internal jugular vein to the right one. Through this anastomosis, the blood from the left side of the head is drained into the original right anterior cardinal vein, which ultimately becomes the superior vena cava, emptying into the right atrium of the heart. Meanwhile, the proximal part of the left common cardinal vein persists as a small channel, the coronary sinus, which is the final drainage pathway of many of the coronary veins, also into the right atrium of the heart.
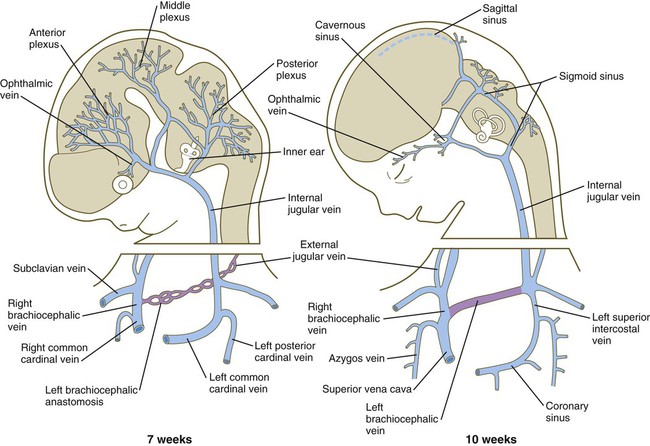
At 7 weeks, an anastomosis (purple) between the left and right jugular vein forms the basis for establishment of the left brachiocephalic vein.
In the trunk, a pair of subcardinal veins arises in association with the developing mesonephros. The subcardinal veins are connected with the posterior cardinal veins and to each other through numerous anastomoses. Both the postcardinal and the subcardinal veins drain the mesonephric kidneys through numerous small side branches. As the mesonephric kidneys start to regress, the veins draining them also begin to break up. At this point, a pair of supracardinal veins appears in the body wall dorsal to the subcardinal veins. Over time, all three sets of cardinal veins in the body break up to varying degrees, with surviving remnants incorporated into the inferior vena cava. The inferior vena cava forms as a single asymmetrical vessel that runs parallel to the aorta on the right (see Fig. 17.13). Most of the named veins of the thoracic and abdominal cavities are derived from persisting segments of the cardinal vein system.
Vitelline and Umbilical Veins
The extraembryonic vitelline and umbilical veins begin as pairs of symmetrical vessels that drain separately into the sinus venosus of the heart (Fig. 17.15). Over time, these vessels become intimately associated with the rapidly growing liver. The vitelline veins, which drain the yolk sac, develop sets of anastomosing channels within and outside the liver. Outside the liver, the two vitelline veins and their side-to-side anastomotic channels become closely associated with the duodenum. Through the persistence of some channels and the disappearance of others, the hepatic portal vein, which drains the intestines, takes shape. Within the liver, the vitelline plexus becomes transformed into a capillary bed that allows the broad distribution of food materials absorbed from the gut through the functional parts of the liver. From the hepatic capillary bed, the blood that arrives from the hepatic portal vein passes into a set of hepatic veins, which empty the blood into the sinus venosus.
Pulmonary Veins
The pulmonary veins are phylogenetically recent structures that form independently, rather than taking over portions of the older cardinal vein systems. From each lung, venous drainage channels converge until they ultimately form a single large common pulmonary vein, which empties into the left atrium of the heart. As the atrium expands, the common pulmonary vein becomes incorporated into its wall (Fig. 17.16). Ultimately, the absorption passes the first and second branch points of the original pulmonary veins, with resulting entrance of four independent pulmonary veins into the left atrium.
Development of Lymphatic Channels
The lymphatic system arises by cells budding off from the lateral surfaces of the anterior and posterior cardinal veins (see Fig. 17.8). As they are preparing to bud off from the veins, the prelymphatic endothelial cells express the homeobox genes Sox18 and Prox-1. This is followed by the expression of VEGFR-3 and their specification to become lymphatic endothelial cells. These cells respond to two specific isoforms of VEGF: VEGF-C and VEGF-D. The emigrating lymphatic endothelial cells aggregate in areas where the lateral plate mesoderm produces VEGF-C to form primary lymph sacs. The first of these are the two jugular lymph sacs arising from the anterior cardinal veins and abdominal lymph sacs, which arise from the endothelium of the posterior cardinal veins (Fig. 17.17). In the abdomen, a retroperitoneal lymph sac forms on the posterior body wall at the root of the mesentery during the eighth week. Later, a cisterna chyli forms at the same level, but dorsal to the aorta. At about the same time, a pair of posterior lymph sacs arises at the bifurcation of the femoral and sciatic veins. By the end of the ninth week, lymphatic vessels connect these lymph sacs.
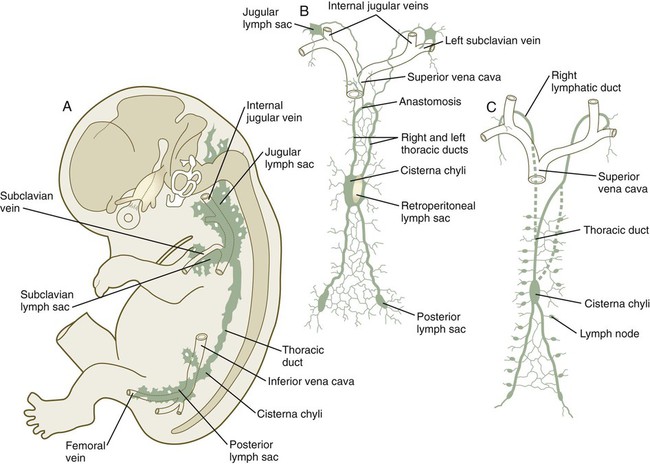
A and B show 9-week-old embryos. C shows the fetal period. Between B and C, the transformation between the reasonably symmetrical disposition of the main lymphatic channels and the asymmetrical condition that is characteristic of an adult can be seen.
Development and Partitioning of the Heart
Early Development of the Heart
Cellular Origins
The heart arises from splanchnic mesoderm. The first part of the heart to develop is the primary heart field (cardiac crescent; see Fig. 6.14B), which forms the left ventricle and the atria, the phylogenetically most primitive components of the mammalian heart. Exposure of the most posterior cells of the cardiac crescent to a retinoic acid gradient arising in the posterior mesoderm conditions these cells to adopt an atrial identity, whereas the more anterior cells not exposed to retinoic acid by default assume a ventricular identity (Fig. 17.18). The appearance of the secondary heart field provides the cellular material for formation of the evolutionarily more recent right ventricle and outflow tract. Cells of the secondary heart field also form the proepicardium (see p. 105) and contribute myocardial cells to the inflow (atrial) areas of the heart.
Several sets of molecules (MEF2, NKX2, GATA, Tbx, and Hand) form what has been called the core regulatory network that guides the differentiation of cardiac tissue. These sets of molecules are differentially regulated, however, by upstream activators that are specific to either the primary or the secondary heart fields (see Fig. 17.18). Even within the core regulatory network, molecular variants are unique to derivatives of the primary or secondary heart fields. Hand-1 is expressed in cells derived from the primary heart field, and in its absence left ventricular anomalies are seen. Hand-2 is expressed in cells derived from the secondary heart field, and if it is not expressed, the right ventricle does not form. Research has shown that the actions of microRNAs are tightly integrated into the overall regulatory programs that guide development of the heart.
As the outflow tract develops, it receives additional cellular contributions from various sources. Endothelial components arise from cephalic paraxial and lateral mesoderm in the region of the otic placode. A major cellular component of the wall of the outflow tract is derived from cardiac neural crest, specifically from the level between the midotic placode to the caudal end of the third somite (see Fig. 12.11). These cranial components integrate with the bilateral cardiac primordia while in the cervical region. As the heart descends into the thoracic cavity, the cranially derived cells of the outflow tract accompany it.
Another source of cells for the developing heart is the proepicardium (see Fig. 6.18). The proepicardium, which consists of an aggregate of mesothelial cells in the pericardium near the inflow area of the early heart, gives rise to the epicardium, most of the interstitial cells of the heart, and the coronary vasculature. Through an epitheliomesenchymal transformation mechanism, some of the epicardial cells transform into the fibroblasts, which invade the heart musculature and constitute most of the interstitial cells of the heart, as well as the smooth muscle cells of the coronary vessels.
Looping of the Heart
The cardiac tube, which takes shape late in the third week, is bilaterally symmetrical (see Fig. 6.17) and is composed principally of cells derived from the primary heart field. As it develops, the heart tube incorporates cells from the secondary heart field. Soon, it undergoes a characteristic dextral looping, making it the first asymmetrical structure to appear in the embryonic body. (The molecular basis for asymmetry of the embryonic body is discussed on p. 87.)
Research has revealed that the outpocketing (ballooning) of the future ventricular and atrial chambers is accompanied by the development of important structural and functional properties. The early symmetrical heart tube (called the primary myocardium) is characterized by slow growth, slow conduction of impulses, slow contraction, and the ability to undergo spontaneous depolarization. As the heart begins to loop, bulges that represent the incipient ventricular and atrial chambers appear on the outer surfaces of the loop and at the inflow end of the heart tube (Fig. 17.19).
The result of cardiac looping is an S-shaped heart in which the originally caudal inflow part of the heart (atrium) becomes positioned dorsal to the outflow tract. In the early heart, the outflow tract is commonly called the bulbus cordis, and it leads directly into the aortic sac and the incipient aortic arch system (Fig. 17.20). In early looping, the cranial limb of the S represents the bulbus cordis, and the middle limb represents the ventricular part of the heart, with the primordium of the right ventricle closest to the outflow tract, and that of the left ventricle next to the caudal limb. The caudal limb represents a common atrium. Later, the common atrium bulges on either side (Fig. 17.21), and an internal septum begins to divide the common ventricle into right and left chambers. The outflow tract (bulbus cordis of the early heart) retains its gross tubular appearance. Its distal part, which leads directly into the aortic arch system, is called the truncus arteriosus. The shorter transitional segment between the truncus and the ventricle is called the conus arteriosus. The conus is separated from the ventricles by faint grooves.
Early Atrioventricular Partitioning of the Heart
Early in heart development, the atrium becomes partially separated from the ventricle by the formation of thickened atrioventricular cushions. A similar but less pronounced thickening forms at the junction between the ventricle and the outflow tract (Fig. 17.22). In these areas, the cardiac jelly, which is organized like a thick basement membrane, protrudes into the atrioventricular canal. The endocardial cushions function as primitive valves that assist in the forward propulsion of blood.
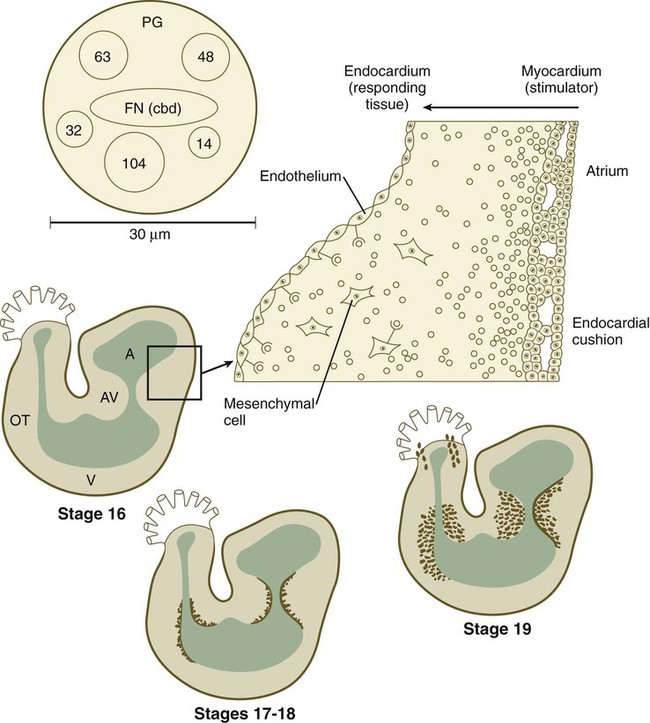
The higher power drawing in the upper right shows details of the transformation of endocardial to mesenchymal cells. Also shown is a hypothetical model of the 30-nm adheronlike particle that seems to induce this transformation in the endocardial cells. Numbered circles indicate other proteins. A, atrium; AV, atrioventricular canal; FN (cbd), fibronectin (cell-binding domain); OT, outlet tract; PG, proteoglycan; V, ventricle. (Based on Bolender DL, Markwald RR: In Feinberg RN, Sherer GK, eds: The development of the vascular system, Basel, 1991, Karger, pp 109-124.)
In response to an inductive action by the underlying primary myocardium, certain antigenically distinct cells from the endocardium of the endocardial cushions lose their epithelial character and become transformed into mesenchymal cells, which migrate into the cardiac jelly. The inductive stimulus, which emanates from primary, but not chamber, myocardial cells, was initially described as being packaged as 20- to 50-nm particles, called adherons (see Fig. 17.22). Current data suggest that TGF-β3 and BMP-2, which act upstream of Notch and Tbx-2, are important components of the overall inductive signal, as a result of which certain endothelial cells express Snail-1/2, Msx-1, and Twist-1. Endocardial cells express the neural cell adhesion molecule (N-CAM) on their surfaces. Those cells that transform into mesenchymal cells downregulate the production of N-CAM, which facilitates their transformation into motile cells. The transformed mesenchymal cells secrete proteases, which destroy inductively active adherons and restore morphogenetic stability to the endocardial cushion regions.
Later Partitioning of the Heart
Separation of the Atria from the Ventricles
The endocardial cushions (see Fig. 17.22), which ultimately become transformed into dense connective tissue, form on the dorsal and ventral walls of the atrioventricular canal. As they grow into the canal, the two cushions meet and separate the atrioventricular canal into right and left channels (Figs. 17.23 and 17.24). The early endocardial cushions serve as primitive valves that assist in the forward propulsion of blood through the heart. Later in development, thin leaflets of anatomical valves take shape in the atrioventricular canal. The definitive valve leaflets do not seem to come from endocardial cushion tissue as much as from invagination of superficial epicardially derived tissues of the atrioventricular groove. The valve that protects the right atrioventricular canal develops three leaflets (tricuspid valve), but the valve in the left canal (mitral, or bicuspid, valve) develops only two. At the molecular level, inhibition of expression of Tbx-2 on the atrial and the ventricular sides of the atrioventricular canal effects the separation between the atria and ventricles.
Partitioning of the Atria
While the atrioventricular canals are forming, a series of structural changes divides the common atrium into separate left and right chambers. Partitioning begins in the fifth week with the downgrowth of a crescentic interatrial septum primum from the cephalic wall between the bulging atrial chambers (see Fig. 17.24). The apices of the crescent of the septum primum extend toward the atrioventricular canal and merge with the endocardial cushions. The space between the leading edge of the septum primum and the endocardial cushions is called the interatrial foramen primum. This space serves as a shunt, permitting blood to pass directly from the right to the left atrium.
When the interatrial septum primum is almost ready to fuse with the endocardial cushions, an area of genetically programmed cell death causes the appearance of multiple perforations near its cephalic end (see Fig. 17.24C). As the leading edge of the septum primum fuses with the endocardial cushions, thus obliterating the foramen primum, the cephalic perforations in the septum primum coalesce and give rise to the interatrial foramen secundum. This new foramen preserves the direct connection between the right and left atria.
Repositioning of the Sinus Venosus and the Venous Inflow into the Right Atrium
During the stage of the straight tubular heart, the sinus venosus is a bilaterally symmetrical chamber into which the major veins of the body empty (see Fig. 17.15). As the heart undergoes looping and the interatrial septa form, the entrance of the sinus venosus shifts completely to the right atrium (see Figs. 17.21 and 17.24). As this occurs, the right horn of the sinus venosus becomes increasingly incorporated into the wall of the right atrium, so the much reduced left horn, the coronary sinus (which is the common drainage channel for the coronary veins), opens directly into the right atrium (see Fig. 17.16). Also in the right atrium, valvelike flaps of tissue (valvulae venosae) form around the entrances of the superior and inferior venae cavae. Because of the orientation of the orifice and its pressure, blood entering the right atrium from the inferior vena cava passes mostly through the interatrial shunt and into the left atrium, whereas blood entering from the superior vena cava and the coronary sinus flows through the tricuspid valve into the right ventricle.
Partitioning of the Ventricles
When the interatrial septa are first forming, a muscular interventricular septum begins to grow from the apex of the ventricular loop between the ballooning right and left ventricular chambers toward the atrioventricular endocardial cushions (see Fig. 17.24C). The early division of the common ventricle is also reflected by the presence of a groove on the outer surface of the heart (Fig. 17.25). Although an interventricular foramen is initially present, it is ultimately obliterated by (1) further growth of the muscular interventricular septum, (2) a contribution by truncoconal ridge tissue that divides the outflow tract of the heart, and (3) a membranous component derived from endocardial cushion connective tissue.
Partitioning of the Outflow Tract of the Heart
In the very early tubular heart, the outflow tract is a single tube, the bulbus cordis. By the time the interventricular septum begins to form, the bulbus has elongated and can be divided into a proximal conus arteriosus and a distal truncus arteriosus (see Fig. 17.20). Closest to the heart, the wall of the outflow tract is composed largely of cells derived from the secondary heart field; more distally, cells derived from the neural crest predominate. Although initially a single channel, the outflow tract is partitioned into separate aortic and pulmonary channels through the appearance of two spiral truncoconal ridges, which are derived largely from neural crest mesenchyme. These ridges bulge into the lumen and finally meet, thus separating the lumen into two channels. The aortic sac, which is located distal to the truncoconal region, does not contain ridges. Partitioning of the outflow tract begins near the ventral aortic root between the fourth and sixth arches and extends toward the ventricles, spiraling as it goes (Fig. 17.26). This accounts for the partial spiraling of the aorta and the pulmonary artery in the adult heart.
At the base of the conus, where endocardial cushion tissue is formed in the same manner as in the atrioventricular canal, two new sets of semilunar valves form (Fig. 17.27). These valves, each of which has three leaflets, prevent ejected blood from washing back into the ventricles. Cranial neural crest cells and cardiac mesoderm contribute to the formation of the semilunar valves. As previously stated, the most proximal extensions of the truncoconal ridges contribute to the formation of the interventricular septum. Just past the aortic side of the aortic semilunar valve, the two coronary arteries join the aorta to supply the heart with blood.
Innervation of the Heart
Although initial heart development occurs independently of nerves, three sets of nerve fibers ultimately innervate the heart (Fig. 17.28). Sympathetic (adrenergic) nerve fibers, which act to speed up the heart beat, arrive as outgrowths from sympathetic ganglia of the trunk. These nerve fibers are derived from trunk neural crest. Parasympathetic (cholinergic) innervation is derived from the cardiac neural crest. Neurons of the cardiac ganglia, which are the second-order parasympathetic neurons, migrate directly to the heart from the cardiac neural crest. These synapse with axons of first-order parasympathetic neurons that gain access to the heart via the vagus nerve. Sensory innervation of the heart is also supplied via the vagus nerve, but the sensory neurons originate from placodal ectoderm (nodose placode) (see Fig. 13.1). The direct innervation of the heart thus has three separate origins.
Initiation of Cardiac Function and the Conducting System of the Heart
The human heart begins to beat 22 to 23 days after fertilization, when it is still in the stage represented by the primary heart myocardium (see p. 107). The beat is slow (<40 beats/minute), and pacemaking activity begins near the inflow region of the heart and spreads toward the outflow tract through spontaneous depolarization of the cells. At this stage, the heart functions like a simple peristaltic pump.
The sinoatrial node is the pacemaker of the mature heart (Fig. 17.29), and it is a direct descendant of the cells in the primary myocardium that initiate the first coordinated heart beats. Terminals of sympathetic and parasympathetic nerve fibers grow into the area to modulate the heart beat. The contractile stimulus then passes to the atrioventricular node through mechanisms still not well understood. From early development, the atrioventricular node, which is also a direct derivative of the primary myocardium, functions to slow down the conductive impulse to separate the contractions of the atrial and ventricular chambers. Activity of the transcriptional repressor Tbx-3 prevents the primary myocardial cells destined to form the sinoatrial and atrioventricular nodes from differentiating into the more highly contractile and more poorly conducting cells that characterize the ventricular chambers. From the atrioventricular node, the pacemaking impulse then passes with increasing velocity down the atrioventricular bundle and into left and right bundle branches before spreading out over the ventricular myocardium as the Purkinje fibers.
The atrioventricular node and bundle arise from a segment of a ring of myocardial cells that initially surround the interventricular foramen and later in some species become translocated to form a figure eight–shaped ring at the atrioventricular junction (see Fig. 17.29). Branches from this ring ultimately pass along either side of the interventricular septum and then arborize along the ventricular walls as Purkinje fibers.
Fetal Circulation
Highly oxygenated blood from the placenta enters the umbilical vein in a large stream that is sometimes under increased pressure because of uterine contractions. Within the substance of the liver, blood from the umbilical vein under higher pressure passes directly into the ductus venosus, which allows it to bypass the small circulatory channels of the liver and flow directly into the inferior vena cava (Fig. 17.30). When in the vena cava, blood has immediate access to the heart. Poorly oxygenated blood flowing in the inferior vena cava can be backed up because of the strength of the umbilical blood flow.
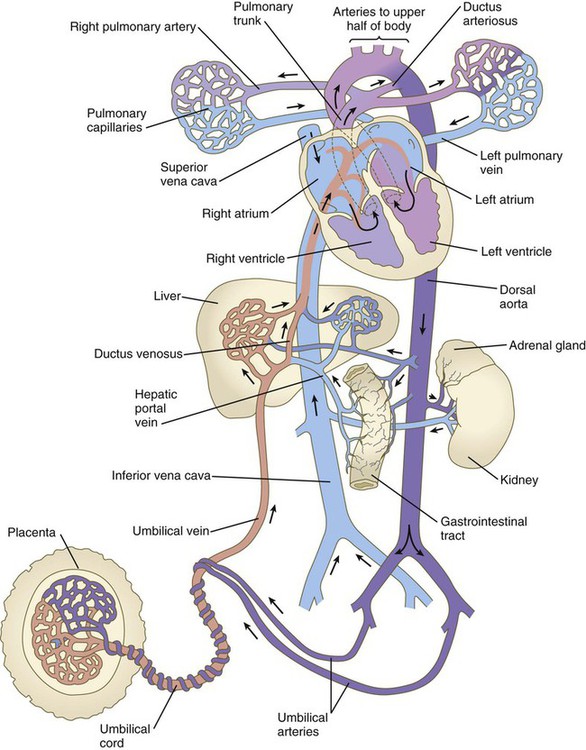
In the right atrium, the orientation of the entrance of the inferior vena cava allows a stream of blood under slightly increased pressure to pass directly through the foramen ovale and foramen secundum into the left atrium (see Fig. 17.30). This is the route normally taken by highly oxygenated umbilical blood entering the body under increased pressure. Because the interatrial shunt of the fetus is smaller than the opening of the inferior vena cava, some of the highly oxygenated caval blood eddies in the right atrium and enters the right ventricle. When low-pressure blood (typically poorly oxygenated systemic blood) enters the right atrium, it joins with the venous blood draining the head through the superior vena cava and the heart through the coronary sinus and is mostly directed through the tricuspid valve into the right ventricle.
Clinical Correlation 17.1 presents malformations of the heart, and Clinical Correlation 17.2 presents malformations of the blood vessels. Table 17.6 summarizes the timelines in cardiac development.
Table 17.6
Timelines in Normal and Abnormal Cardiac Development
Normal Time | Developmental Events | Malformations Arising during Period |
18 days | Horseshoe-shaped cardiac primordium appears | Lethal mutants |
20 days | Bilateral cardiac primordia fuse | Cardia bifida (experimental) |
Cardiac jelly appears | — | |
Aortic arch is forming | — | |
22 days | Heart is looping into S shape | Dextrocardia |
Heart begins to beat | — | |
Dorsal mesocardium is breaking down | — | |
Aortic arches I and II are forming | — | |
24 days | Atria are beginning to bulge | — |
Right and left ventricles act like two pumps in series | — | |
Outflow tract is distinguishable from right ventricle | — | |
Late fourth week | Sinus venosus is becoming incorporated into right atrium | Venous inflow malformations |
Endocardial cushions appear | Persistent atrioventricular canal | |
Early septum I appears between left and right atria | Common atrium | |
Muscular interventricular septum is forming | Common ventricle | |
Truncoconal ridges are forming | Persistent truncus arteriosus | |
Aortic arch I is regressing | — | |
Aortic arch III is formed | — | |
Aortic arch IV is forming | Missing fourth aortic arch segment | |
Early fifth week | Endocardial cushions are coming together, forming right and left atrioventricular canals | Persistent atrioventricular canal |
Further growth of interatrial septum I and muscular interventricular septum occurs | Muscular ventricular septal defects | |
Truncus arteriosus is dividing into aorta and pulmonary artery | Transposition of great vessels; aortic and pulmonary stenosis or atresia | |
Atrioventricular bundle is forming; possible neurogenic control of heart beat | — | |
Pulmonary veins are becoming incorporated into left atrium | Aberrant pulmonary drainage | |
Aortic arches I and II have regressed | — | |
Aortic arches III and IV have formed | — | |
Aortic arch VI is forming | — | |
Conduction system forms | — | |
Late fifth to early sixth week | Endocardial cushions fuse | — |
Interatrial foramen II is forming | — | |
Interatrial septum I is almost contacting endocardial cushion | Low atrial septal defects | |
Membranous part of interventricular septum starts to form | Membranous interventricular septal defects | |
Semilunar valves begin to form | Aortic and pulmonary valvular stenosis | |
Late sixth week | Interatrial foramen II is large | High atrial septal defects |
Interatrial septum II starts to form | — | |
Atrioventricular valves and papillary muscles are forming | Tricuspid or mitral valvular stenosis or atresia | |
Interventricular septum is almost complete | Membranous interventricular septal defects | |
Coronary circulation is becoming established | — | |
Eighth to ninth week | Membranous part of interventricular septum is completed | Membranous interventricular septal defects |
Summary
The vascular system arises from mesodermal blood islands in the wall of the yolk sac. Hemangioblasts give rise to either blood cells or vascular endothelial cells. Nucleated red blood cells produced in the blood islands are the first blood cells found in the embryo. Later, hematopoiesis shifts to the embryonic body, beginning in the para-aortic bodies, and then to the liver, and finally to the bone marrow.
During hematopoiesis, hemocytoblasts give rise to lymphoid and myeloid stem cells. Each of these stem cells further differentiates into the definitive lines of blood cells. Erythropoiesis involves the passage of precursor cells of red blood cells through several stages. The earliest stages are defined by behavioral, rather than morphological, characteristics. During later stages of differentiation, the precursor cells of erythrocytes gradually lose their RNA-producing machinery and accumulate increasing amounts of hemoglobin in their cytoplasm; at the same time, the nucleus becomes more condensed and is eventually lost. Hemoglobin also undergoes isoform transitions during embryonic development.
The earliest blood and associated extraembryonic blood vessels arise from blood islands in the mesodermal wall of the yolk sac. Much of the vasculature of the embryonic body is derived from intraembryonic sources. Endothelial cell precursors (angioblasts) arise from most mesodermal tissues of the body except the notochord and prechordal mesoderm. Embryonic blood vessels form by three main mechanisms: (1) coalescence in situ (vasculogenesis), (2) migration of angioblasts into organs, and (3) sprouting from existing vessels (angiogenesis). Ingrowth of blood vessels into some organ primordia is stimulated by VEGF or other angiogenic factors.
The first three pairs of aortic arches form arteries that supply the head. The fourth pair of arches develops asymmetrically, with the left arch forming part of the aortic arch of adults. The fifth pair of arches never forms. A sixth pair of arches arises as a capillary plexus that connects with the fourth arch. The distal part of the left sixth arch forms the ductus arteriosus, a shunt that allows blood to bypass the immature lungs and enter the aorta directly. Many of the larger arteries of adults arise from three sets of aortic branches: the dorsal intersegmental, the lateral segmental, and the ventral segmental. The coronary arteries arise from capillary plexuses associated with the epicardium. These plexuses are secondarily connected with the aorta.
The venous system arises from very complex capillary networks that initially develop into components of the cardinal vein system. Anterior and posterior cardinal veins drain the head and trunk. They then empty into the paired common cardinal veins and ultimately into the sinus venosus of the heart. Paired subcardinal veins are associated with the developing mesonephros. Paired extraembryonic umbilical and vitelline veins pass through the developing liver and directly into the sinus venosus. The pulmonary veins arise as separate structures and empty into the left atrium. The lymphatic system first appears as six primary lymph sacs. These become connected by lymphatic channels. Lymphatics from most of the body collect into the thoracic duct, which empties into the venous system at the base of the left internal jugular vein.
The heart arises from splanchnic mesoderm as a horseshoe-shaped primordium consisting of primary and secondary heart fields. Originally, bilateral endocardial tubes fuse in the midline. The fused cardiac tube then undergoes an S-shaped looping, and, soon, specific regions of the heart can be identified. Starting with the inflow tract, these regions are the sinus venosus, the atria, the ventricles, and the outflow tract (bulbus cordis). The outflow tract later divides into the conus arteriosus and the truncus arteriosus.
Atrial endocardial cushions are thickenings between the atria and ventricles. The underlying myocardium induces cells from the endothelial lining of the endocardial cushion to leave the endocardial layer and transform into mesenchymal cells that invade the cardiac jelly. These events serve as the basis for the formation of the atrioventricular valves.
Internal partitioning of the heart begins with the separation of the atria from the ventricles and formation of the mitral and tricuspid valves. The left and right atria become separated by growth of the septum primum and septum secundum, but throughout embryonic life a shunt remains from the right to the left atrium via the foramen secundum and foramen ovale. The sinus venosus and the venae cavae empty into the right atrium, and the pulmonary veins drain into the left atrium. The ventricles are divided by the interventricular septum. Spiral truncoconal ridges partition the common outflow tract into pulmonary and aortic trunks. Semilunar valves prevent the reflux of blood in these vessels into the heart.
In addition to sensory innervation, the heart receives sympathetic and parasympathetic innervation. The conduction system distributes the contractile stimulus throughout the heart. The conduction system is derived from modified cardiac muscle cells. The heart begins to beat early in the fourth week of gestation. Physiological maturation of the heart beat follows maturation of the pacemaker system and the innervation of the heart.
The fetal circulation brings oxygenated blood from the placenta through the umbilical vein and into the right atrium, where much of it is shunted into the left atrium. Other blood entering the right atrium passes into the right ventricle. Blood leaving the right ventricle enters the pulmonary trunk, which supplies some blood to the lungs and most to the aorta via the ductus arteriosus. Blood in the left atrium empties into the left ventricle and aorta, where it supplies the body. Poorly oxygenated blood enters the umbilical arteries and is carried to the placenta for renewal.
Common malformations of the heart consist of atrial septal defects, which in postnatal life allow blood to pass from the left to the right atrium. Ventricular septal defects, which also result in a left-to-right shunting of blood, are more serious. Defects that block a channel for blood flow (e.g., tricuspid atresia) must be accompanied by secondary shunt defects to be compatible with life. A persistent atrioventricular canal can be attributed to a defect in the formation or further development of the atrioventricular endocardial cushions. Most malformations of the outflow tract of the heart seem to be related to inappropriate partitioning by the truncoconal ridges. The basis for this is frequently found in neural crest abnormalities.
Malformations of the major arteries often result from the inappropriate appearance or disappearance of specific components of the aortic arch system. Some malformations, such as double aortic arch or right aortic arch, can interfere with swallowing or breathing because of pressure. Patent ductus arteriosus is caused by the failure of the ductus arteriosus to close properly after birth. Coarctation of the aorta must be compensated by either a patent ductus arteriosus or the opening of collateral vascular channels that allows blood to bypass the site of coarctation.
Because of their complex mode of origin, veins are commonly subject to considerable variation, but these malformations are frequently asymptomatic. Anomalous pulmonary return, which brings oxygenated blood into the right atrium, must be accompanied by a right-to-left shunt to be compatible with life. Malformations of the lymphatic system can cause local swellings such as cystic hygroma, which results in a collarlike swelling in the neck.