Objectives
Be sure to check out the bonus material, including free self-assessment exercises, on the Evolve web site at
http://evolve.elsevier.com/Urden/priorities/.
• Identify the components of a cardiovascular history.
• Outline the steps to interpret a change in Scvo2/Svo2 values.
• Outline the steps in analyzing an ECG rhythm strip.
• Explain the significance of normal and abnormal ECG findings.
• Discuss the different purposes of three cardiovascular diagnostic procedures.
Physical assessment of the cardiovascular patient is a skill that must not be lost amid the technology of the critical care setting. Data collected from a thorough, thoughtful history and examination contribute to both the nursing and the medical decisions for therapeutic interventions.
History
The patient history is important because it provides data that contribute to the cardiovascular diagnosis and treatment plan. For a patient in acute distress, the history is curtailed to just a few questions about the patient’s chief complaint, the precipitating events, and current medications. For a patient without obvious distress, the history focuses on the following four areas:
1. Review of the patient’s present illness.
4. Survey of the patient’s lifestyle, including risk factors for CAD.
One of the unique challenges in cardiovascular assessment is identifying when “chest pain” is of cardiac origin and when it is not. The following safety information should always be considered:
• There is not always a correlation between the location of chest discomfort and its source because of referred pain. For example, in patients with gastroesophageal reflux disease (GERD), esophageal spasm can cause visceral substernal chest pain that radiates to the left arm and jaw, described by patients as “heartburn.”1,2
In a meta-analysis of the evaluation of stable, intermittent chest pain, a patient’s description of chest pain was found to be the most important predictor of underlying coronary disease.3 In the evaluation of acute chest pain, the 12-lead electrocardiogram was the most useful bedside predictor for a diagnosis of ST-elevation myocardial infarction (STEMI).3
Physical Examination
A comprehensive physical assessment is fundamental to the achievement of an accurate diagnosis. The nurse who has developed the skills of inspection, palpation, and auscultation can be confident when assessing patients with cardiovascular disease. Percussion is not employed when assessing the cardiovascular system.
Inspection
The priorities for inspection of the patient with cardiovascular dysfunction are: (1) assessing the general appearance; (2) examining the extremities; (3) estimating jugular venous distention; and (4) observing the apical impulse.
Assessing General Appearance
The face is observed for the color of the skin (i.e., cyanotic, pale, or jaundiced) and for apprehensive or painful expressions. The skin, lips, tongue, and mucous membranes are inspected for pallor or cyanosis. Central cyanosis is a bluish discoloration of the tongue and sublingual area. Multiracial studies indicate that the tongue is the most sensitive site for observation of central cyanosis, which must be recognized and treated as a medical emergency. Pulse oximetry, arterial blood gas analysis, and treatment with 100% oxygen must be instituted immediately.
The anterior thorax and posterior thorax are inspected for skeletal deformities that may displace the heart and cause cardiac compromise. The skin on the chest wall and abdomen is inspected for scars, bruises, wounds, and bulges associated with pacemaker or defibrillator implants. Respiratory rate, pattern, and effort are also observed and recorded. The abdomen is assessed for signs of distention or ascites that may be associated with right-sided heart failure. Abdominal adiposity is a known risk factor for CAD.
Body posture can indicate the amount of effort it takes to breathe. For example, sitting upright to breathe may be necessary for the patient with acute heart failure, and leaning forward may be the least painful position for the patient with pericarditis. The patient is observed for signs of confusion or lethargy that may indicate hypotension, low cardiac output (CO), or hypoxemia.
Examining the Extremities
The legs are inspected for signs of peripheral arterial or venous vascular disease. The visible signs of arterial vascular disease include pale, shiny legs with sparse hair growth. Venous disease creates an edematous limb with deep red rubor, brown discoloration, and, frequently, leg ulceration. A comparison of arterial and venous disease is presented in Table 11-1.
TABLE 11-1
INSPECTION AND PALPATION OF EXTREMITIES: COMPARISON OF ARTERIAL AND VENOUS DISEASE
CHARACTERISTIC | ARTERIAL DISEASE | VENOUS DISEASE |
Hair loss | Present | Absent |
Skin texture | Thin, shiny, dry | Flaking, stasis, dermatitis, mottled |
Ulceration | Located at pressure points; painful, pale, dry with little drainage; well-demarcated with eschar or dried; surrounded by fibrous tissue; granulation tissue scant and pale | Usually at the ankles; painless, pink, moist with large amount of drainage; irregular, dry, and scaly; surrounded by dermatitis; granulation tissue healthy |
Skin color | Elevational pallor, dependent rubor | Brown patches, rubor, mottled cyanotic color when dependent |
Nails | Thick, brittle | Normal |
Varicose veins | Absent | Present |
Temperature | Cool | Warm |
Capillary refill | Greater than 3 seconds | Less than 3 seconds |
Edema | None or mild, usually unilateral | Usually present foot to calf, unilateral or bilateral |
Pulses | Weak or absent (0 to 1+) | Normal, strong, and symmetric |
Modified from Krenzer ME: Peripheral vascular assessment: finding your way through arteries and veins, AACN Clin Issues 6(4):631, 1995.
The nail beds are inspected for signs of discoloration or cyanosis. Clubbing in the nail bed is a sign associated with long-standing central cyanotic heart disease or pulmonary disease with hypoxemia.4 Clubbing describes a nail that has lost the normal angle between the finger and the nail root; the nail becomes wide and convex. The terminal phalanx of the finger also becomes bulbous and swollen, sometimes described as drumstick fingers.5 Clubbing is rare. It denotes long-standing severe central cyanosis (Figure 11-1). Platelet-derived vascular endothelial growth factor is thought to play a key role in the development of clubbing.6
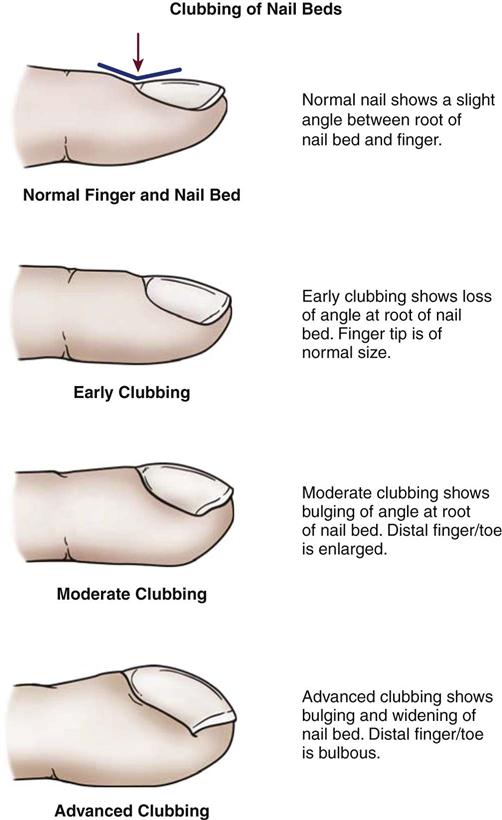
Peripheral cyanosis, a bluish discoloration of the nail bed, is more commonly seen. Peripheral cyanosis results from a reduction in the quantity of oxygen in the peripheral extremities from arterial disease or decreased CO. Clubbing never occurs as a result of peripheral cyanosis.
Estimating Jugular Venous Distention
The jugular veins of the neck are inspected for a noninvasive estimate of intravascular volume and pressure. The internal jugular veins are observed for jugular vein distention (JVD) (Figure 11-2 and Box 11-1). JVD is caused by an elevated central venous pressure (CVP).7 This occurs with fluid volume overload and right ventricular dysfunction, which elevates right atrial pressure.8 The right internal jugular vein can be used for measurement of CVP in centimeters of water (Figure 11-3 and Box 11-2).9–11
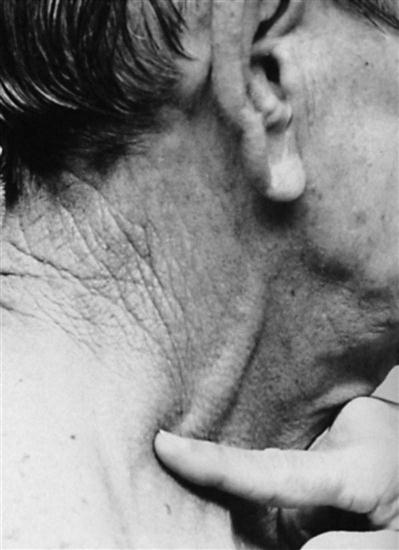
Applying light finger pressure over the sternocleidomastoid muscle, parallel to the clavicle, helps identify the external jugular vein by occluding flow and distending it. The finger pressure is released, and the patient is observed for true distention. If the patient’s trunk is elevated to 30 degrees or more, JVD should not be present.
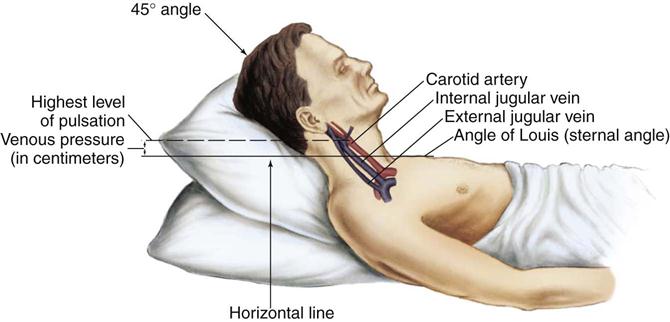
Pulsation in the internal jugular vein can be used to estimate central venous pressure. (Modified from Thompson JM, et al: Mosby’s clinical nursing, ed 5, St Louis, 2002, Mosby.)
The abdominojugular reflux sign can assist with the diagnosis of right ventricular failure. This noninvasive test is used in conjunction with measurement of JVD. The procedure for assessing abdominojugular reflux is described in Box 11-3. A positive abdominojugular reflux sign is an increase in the jugular venous pressure (CVP equivalent) of 4 cm or more sustained for at least 15 seconds.12
Observing the Apical Impulse
The thoracic cage is divided with imaginary vertical lines (sternal, midclavicular, axillary, vertebral, and scapular), and the intercostal spaces are divided with horizontal lines to serve as reference points in locating or describing cardiac findings (Figure 11-4). The anterior thorax is inspected for the apical impulse, sometimes referred to as the point of maximal impulse (PMI). The apical impulse occurs as the left ventricle contracts during systole and rotates forward, causing the left ventricular apex of the heart to hit the chest wall. The apical impulse is a quick, localized, outward movement normally located just lateral to the left midclavicular line at the fifth intercostal space in the adult patient (Figure 11-5). The apical impulse is the only normal pulsation visualized on the chest wall. In the patient without cardiac disease, PMI may not be noticeable (see Figure 11-5).
Palpation
The priorities for palpation of the patient with cardiovascular dysfunction are: (1) assessing arterial pulses; (2) evaluating capillary refill; (3) estimating edema; and (4) assessing for signs of deep vein thrombosis.
Assessing Arterial Pulses
Seven pairs of bilateral arterial pulses are palpated. The examination incorporates bilateral assessment of the carotid, brachial, radial, ulnar, popliteal, dorsalis pedis, and posterior tibial arteries. The pulses are palpated separately and compared bilaterally to check for consistency. Pulse volume is graded on a scale of 0 to 3+ (Box 11-4). The abdominal aortic pulse can also be palpated. If a distal pulse cannot be palpated using light finger pressure, a Doppler ultrasound stethoscope can increase diagnostic accuracy.13 It is important to mark the location of the audible signal with an indelible ink marker pen for future evaluation of pulse quality. The radial and ulnar arterial pulses must be evaluated for collateral flow before an arterial line is inserted; this test, known as the Allen test, is described in Box 11-5.
Evaluating Capillary Refill
Capillary refill assessment is a maneuver that uses the patient’s nail beds to evaluate arterial circulation to the extremity and overall perfusion. The nail bed is compressed to produce blanching, after which release of the pressure should result in a return of blood flow and baseline nail color within 2 seconds.14 The severity of arterial insufficiency is directly proportional to the amount of time required to reestablish baseline flow and color.
Estimating Edema
Edema is fluid accumulation in the extravascular spaces of the body. The dependent tissues within the legs and sacrum are particularly susceptible. The nurse should observe whether the edema is dependent, unilateral or bilateral, pitting or nonpitting. The amount of edema is quantified by measuring the circumference of the limb or by pressing the skin of the feet, ankles, and shins against the underlying bone. Edema is a symptom associated with several diseases, and further diagnostic evaluation is required to determine the cause. Although no universal scale for pitting edema exists, one example is a 0 to 4+ system (Table 11-2).
TABLE 11-2
PITTING EDEMA SCALE INDENTATION DEPTH
SCALE | EDEMA | ENGLISH UNITS | METRIC UNITS | TIME TO BASELINE |
0 | None | 0 | 0 | |
1+ | Trace | 0-0.25 inch | <6.5 mm | Rapid |
2+ | Mild | 0.25-0.5 inch | 6.5-12.5 mm | 6.5-12.5 mm |
3+ | Moderate | 0.5-1 inch | 12.5 mm-2.5 cm | 1-2 min |
4+ | Severe | >1 inch | >2.5 cm | 2-5 min |
Auscultation
The priorities for auscultation of the patient with cardiovascular dysfunction are: (1) measuring blood pressure; (2) detecting orthostatic hypotension; (3) measuring pulse pressure (4) detecting pulsus paradoxus; (5) assessing normal heart sounds; and (6) identifying abnormal heart sounds, murmurs, and pericardial rubs.
Measuring Blood Pressure
Blood pressure measurement is an essential component of every complete physical examination. Hypertension is diagnosed as a systolic blood pressure (SBP) of 140 mm Hg or higher, or a diastolic blood pressure (DBP) of 90 mm Hg or above.15 Prehypertension is defined as an SBP in the range of 120 to 139 mm Hg in association with a DBP between 80 to 89 mm Hg.15,16 The incidence of hypertension in the United States has increased dramatically as a result of an aging population and an increasing prevalence of obesity. During the period from 1999 to 2000, 65 million adults in the United States were hypertensive, compared with 50 million in 1988 through 1994—an increase of 30%.17 Risk of hypertension increases with older age. More than 90% of people who have a normal blood pressure at 55 years of age eventually develop hypertension, according to findings from the Framingham Heart Study.16
In the critical care setting, systemic blood pressure can be measured directly or indirectly. Arterial monitoring devices that directly measure arterial pressure by means of an invasive catheter technique are considered the gold standard.18 Accurate use of a stethoscope and sphygmomanometer or electronic measuring devices can produce indirect blood pressure values that closely reflect direct measurements.19
Detecting Orthostatic Hypotension
When a healthy person stands, 10% to 15% of the blood volume is pooled in the legs; this reduces venous return to the right side of the heart, which decreases CO and lowers arterial blood pressure.20 The fall in blood pressure activates baroreceptors; the subsequent reflex increase in sympathetic outflow and parasympathetic inhibition leads to peripheral vasoconstriction, with increased heart rate and contractility.20 Postural (orthostatic) hypotension occurs when the SBP drops 10 to 20 mm Hg or the diastolic BP drops 5 mm Hg after a change from the supine to the upright posture.20,21 It is usually accompanied by complaints of dizziness, lightheadedness, or syncope. If a patient experiences these symptoms, it is important to complete a full set of postural vital signs before increasing the patient’s activity level (Box 11-6). Orthostatic hypotension can have many causes. The three most common causes of orthostatic vital sign changes (i.e., drop in blood pressure and rise in heart rate) observed in critical care are:
Measuring Pulse Pressure
Pulse pressure describes the difference between the systolic and diastolic blood pressure values. The normal pulse pressure is 40 mm Hg (i.e., the difference between an SBP of 120 mm Hg and a DBP of 80 mm Hg). In the critically ill patient, a low blood pressure is frequently associated with a narrow pulse pressure. For example, a patient with a blood pressure of 90/72 mm Hg has a pulse pressure of 18 mm Hg. The narrowed pulse pressure is a temporary compensatory mechanism caused by arterial vasoconstriction resulting from volume depletion or heart failure. The narrow pulse pressure ensures that the MAP (78 mm Hg in this example) remains in a therapeutic range to provide adequate organ perfusion.
In contrast, a hypotensive septic patient who exhibits vasodilation will have a wide pulse pressure and inadequate organ perfusion. If the blood pressure is 90/36 mm Hg, the pulse pressure is 54 mm Hg, and the MAP calculates to an inadequate 54 mm Hg. In both of these examples, the SBP is the same (90 mm Hg); the difference in pulse pressure is a function of intravascular volume and vascular tone.
Detecting Pulsus Paradoxus
In normal physiology, the strength of the pulse fluctuates throughout the respiratory cycle. When the “pulse” is measured using the SBP, the pressure is observed to decrease slightly during inspiration and to rise slightly during respiratory exhalation. The normal difference is 2 to 4 mm Hg.8 One exception is cardiac tamponade, where the blood pressure decline is abnormally large during inspiration. In general, an inspiratory decline of SBP greater than 10 mm Hg is considered diagnostic of pulsus paradoxus.22–24 The traditional technique for measuring pulsus paradoxus using a sphygmomanometer and a blood pressure cuff18 and pulse oximetry22–24 is described in Box 11-7.18 If the patient is hypotensive, pulsus paradoxus is more accurately assessed in the critical care unit by monitoring a pulse oximetry waveform or an indwelling arterial catheter waveform.22–24
Assessing Normal Heart Sounds
Auscultation of the heart is the most challenging part of the cardiac physical examination, and, in an era of increasing technological demands, it is daunting to new clinicians.25 To summarize the advice given by most experts, the examiner must do the following:
1. Auscultate systematically across the precordium.26
2. Visualize the cardiac anatomy under each point of auscultation, expecting to hear the physiologically-associated sounds.26
3. Memorize the cardiac cycle to enhance the ability to hear abnormal sounds.26
First and Second Heart Sounds.
Normal heart sounds are referred to as the first heart sound (S1) and the second heart sound (S2). S1 is the sound associated with mitral and tricuspid valve closure and is heard most clearly in the mitral and tricuspid areas. S2 (aortic and pulmonic closure) can be heard best at the second intercostal space to the right and left of the sternum (see Figure 11-5). Both sounds are high-pitched and heard best with the diaphragm of the stethoscope (Box 11-8). Each sound is loudest in an auscultation area located downstream from the actual valvular component of the sound, as shown in Figure 11-6.
Pathological Splitting of S1 and S2.
A variety of abnormalities can alter the intensity and timing of split heart sounds. For example, during auscultation in the pulmonic area, a pathological split is audible with a stethoscope if the pulmonic valve closure occurs after the aortic valve closure. Pathological splitting of S1 and S2 is associated with specific cardiovascular conditions such as pulmonary hypertension, pulmonic stenosis, right ventricular failure, and with electrical conduction disturbances such as right bundle branch block and premature ventricular contractions.
Identifying Abnormal Heart Sounds, Murmurs, and Pericardial Rubs
Third and Fourth Heart Sounds.
The abnormal heart sounds are known as the third heart sound (S3) and the fourth heart sound (S4); they are referred to as gallops when auscultated during an episode of tachycardia. These low-pitched sounds occur during diastole and are best heard with the bell of the stethoscope positioned lightly over the apical impulse. The characteristics of S3 and S4 are detailed in Box 11-9. The presence of S3 may be normal in children, young adults, and pregnant women because of rapid filling of the ventricle in a young, healthy heart.28 However, an S3 in the presence of cardiac symptoms is an indicator of heart failure in a noncompliant ventricle with fluid overload.29 Not unexpectedly, the development of an S3 heart sound is strongly associated with elevated levels of brain natriuretic peptide (BNP).29,30
Auscultation of an S4 also leads the examiner to suspect heart failure and decreased ventricular compliance. An S4, also referred to as an atrial gallop, occurs at the end of diastole (just before S1), when the ventricle is full. The sound is associated with atrial contraction, also called atrial kick.
Heart Murmurs.
Heart valve murmurs are prolonged extra sounds that occur during systole or diastole. Murmurs are produced by turbulent blood flow through the chambers of the heart, which results in vibrations that occur during systole or diastole. Most murmurs are caused by structural cardiac changes. The steps to effectively and accurately auscultate for cardiac murmurs are listed in Box 11-10. Murmurs are characterized by specific criteria:
Pericardial Friction Rub.
A pericardial friction rub is a sound that can occur within 2 to 7 days after a myocardial infarction. The friction rub results from pericardial inflammation (pericarditis). Classically, a pericardia friction rub is a grating or scratching sound that is both systolic and diastolic, corresponding with cardiac motion within the pericardial sac. It is often associated with chest pain, which can be aggravated by deep inspiration, coughing, swallowing, and changing position. It is important to differentiate pericarditis from acute myocardial ischemia, and the detection of a pericardial friction rub through auscultation can assist in this differentiation, leading to effective diagnosis and treatment.
Bedside Hemodynamic Monitoring
Hemodynamic monitoring is at a critical juncture. The technology that launched invasive hemodynamic monitoring is more than 30 years old, and the search to find viable replacement monitoring technologies that are minimal or noninvasive is intense. This has created a new challenge in critical care. Although the use of invasive therapies is declining, they are still employed for hemodynamically unstable patients. Critical care nurses must be knowledgeable about traditional hemodynamic monitoring methods and be able to apply established physiological principles in new situations. As the technology evolves, the critical care nurse will apply the same physiological principles to the new methods to ensure safety and optimal outcomes for each patient.
Equipment
A traditional hemodynamic monitoring system has four component parts, as shown in Figure 11-7 and described in the following list:
1. An invasive catheter and high-pressure tubing connect the patient to the transducer.
3. The flush system maintains patency of the fluid-filled system and catheter.
Although many different types of invasive catheters can be inserted to monitor hemodynamic pressures, all such catheters are connected to similar equipment (see Figure 11-7). Even so, there remains considerable variation in the way different hospitals configure their hemodynamic systems. The basic setup consists of the following:
Heparin
The use of the anticoagulant heparin added to the normal saline (NS) flush setup to maintain catheter patency remains controversial. A systematic review of the literature showed that a heparinized flush solution is associated with a longer duration of catheter patency.31 Other units do not use heparin because of concern about development of the autoimmune condition known as heparin-induced thrombocytopenia (HIT). This is sometimes described as a “heparin allergy” and, when present, is associated with a dramatic drop in platelet count, and thrombus formation. Some trials have not found platelet counts or catheter duration to be influenced by heparin.32,33 If heparin is used in the flush infusion, monitoring the trend in the platelet count is recommended.34
The flush solutions and tubing are usually changed every 72 to 96 hours. There is variety; some hospitals change flush solutions every 24 hours. For this reason, it is essential to be familiar with the specific written procedures that concern hemodynamic monitoring equipment in each critical care unit.
Calibrating Hemodynamic Monitoring Equipment
To ensure accuracy of hemodynamic pressure readings, two baseline measurements are necessary:
1. Calibration of the system to atmospheric pressure, also known as zeroing the transducer
2. Determination of the phlebostatic axis for transducer height placement, also called leveling the transducer35
Zeroing the Transducer.
To calibrate the equipment to atmospheric pressure, referred to as zeroing the transducer, the three-way stopcock nearest to the transducer is turned simultaneously to open the transducer to air (atmospheric pressure) and to close it to the patient and the flush system. The monitor is adjusted so that “0” is displayed, which equals atmospheric pressure. Atmospheric pressure is not zero; it is 760 mm Hg at sea level. Using zero to represent current atmospheric pressure provides a convenient baseline for hemodynamic measurement purposes.
Some monitors also require calibration of the upper scale limit while the system remains open to air. At the end of the calibration procedure, the stopcock is returned to the closed position and a closed cap is placed over the open port. At this point, the patient’s waveform and hemodynamic pressures are displayed.
Disposable transducers are very accurate, and after they are calibrated to atmospheric pressure, drift from the zero baseline is minimal. Although in theory this means that repeated calibration is unnecessary, clinical protocols in most units require the nurse to calibrate the transducer at the beginning of each shift for quality assurance.
Phlebostatic Axis.
The phlebostatic axis is an external physical reference point on the chest that is used to ensure consistent transducer height placement. To obtain the axis, a theoretic line is drawn from the fourth intercostal space (ICS), where it joins the sternum to a midaxillary line on the side of the chest. The midaxillary line is one half of the anteroposterior (AP) depth of the lateral chest wall.35 This line approximates the level of the atria, as shown in Figure 11-7. The phlebostatic axis is used as the reference mark for central venous pressure (CVP) and pulmonary artery catheter transducers. The level of the transducer approximates the level of the tip of an invasive hemodynamic monitoring catheter within the chest.
Leveling the Transducer.
Leveling the transducer is different from zeroing. This process aligns the transducer with the level of the left atrium. The purpose is to line up the air-fluid interface with the left atrium to correct for changes in hydrostatic pressure in blood vessels above and below the level of the heart.35
A carpenter’s level or laser-light level can be used to ensure that the transducer is parallel with the phlebostatic axis reference point. When there is a change in the patient’s position, the transducer must be leveled again to ensure accurate hemodynamic pressure measurements are obtained.35 Errors in measurement can occur if the transducer is placed above or below the phlebostatic axis.36 If the transducer is placed below this level, the fluid in the system weighs on the transducer, creating additional hydrostatic pressure, to produce a falsely high reading. For every inch the transducer is below the tip of the catheter, the fluid pressure in the system increases the measurement by 1.87 mm Hg. For example, if the transducer is positioned 6 inches below the tip of the catheter, this falsely elevates the displayed pressure by 11 mm Hg.
If the transducer is placed above this atrial level, gravity and lack of fluid pressure will give an erroneously low reading. For every inch the transducer is positioned above the catheter tip, the measurement is 1.87 mm Hg less than the true value. If several clinicians are taking measurements, the reference point can be marked on the side of the patient’s chest to ensure accurate measurements.35 The American Association of Critical-Care Nurses (AACN) has an audit tool on their website that can be downloaded to assess whether all appropriate quality assurance measures for accurate hemodynamic monitoring have been followed.37
Recognizing Normal Hemodynamic Values
Once the system is correctly calibrated, the clinical team uses the known normal values as a reference when evaluating the patient’s hemodynamic response to therapeutic interventions.
Accommodating Changes in Patient Position
Position of the hemodynamically monitored patient would not be an issue if critical care patients only lay flat in the bed. However, lying flat is not always a comfortable position, especially if the patient is alert or if the head of the bed needs to be elevated to decrease the work of breathing.
Head-of-Bed Position.
Nurse researchers have determined that the CVP, pulmonary artery pressure (PAP), and pulmonary artery occlusion pressure (PAOP, also called pulmonary artery wedge pressure [PAWP]) can be reliably measured at head-of-bed positions from 0 (flat) to 60 degrees if the patient is lying on his or her back (supine).35 If the patient is normovolemic and hemodynamically stable, raising the head of the bed usually does not affect hemodynamic pressure measurements. If the patient is so hemodynamically unstable or hypovolemic that raising the angle of the head of the bed negatively affects intravascular volume distribution, the first priority is to correct the hemodynamic instability and leave the patient in a supine position. In summary, most patients do not need the head of the bed to be lowered to 0 degrees (flat) to obtain accurate CVP, PAP, or PAOP readings.
Lateral Position.
The landmarks for leveling the transducer are different if the patient is turned to the side. Researchers have evaluated hemodynamic pressure measurement readings with patients positioned in 30- and 90-degree lateral positions, with the head of the bed flat. The following transducer landmarks are recommended to achieve reliable measurements:35
• 30-degree lateral position: level the transducer at one half of the distance from the surface of the bed to the left sternal border.35
• 90-degree right-lateral position: level the transducer at the fourth ICS at the mid-sternum.35
• 90-degree left-lateral position: level the transducer at the fourth ICS at the left parasternal border (beside the sternum).35
It is important to know that measurements can be recorded in lateral positions, because critically ill patients must be turned to prevent development of pressure ulcers and other complications of immobility.
Establishing Safe Monitor Alarm Limits
All bedside hemodynamic monitoring systems have alarm limits that are preset to ensure patient safety. The alarms must be sufficiently distinctive and audible to be heard over the noise of a typical critical care unit. Patient safety guidelines are designed to promote clinical alarm goals. Some clinical situations create special challenges with respect to alarm safety. Nursing care actions that cause the patient to move in the bed will often trigger the alarms. Temporarily silencing the sound for 1 to 3 minutes while continuing to observe the bedside monitor is appropriate. The real challenge occurs when a patient is restless or fidgeting with IV tubing or electrodes, resulting in the alarms bring constantly triggered because the monitor is unable to evaluate the ECG rhythms and hemodynamic waveforms effectively. It is tempting to silence these “nuisance alarms” permanently. The alarms should not be turned off, however, because the patient is left in a vulnerable position if a dysrhythmia or hemodynamic complication arises. Clinical interventions to ameliorate the root cause of the problem (e.g., restlessness) are more appropriate. Education of nurses about the risk of becoming desensitized to the sound of beside alarms is also important.38 Key issues concerning monitor alarms are presented in the Patient Safety Priorities box on Clinical Alarms.
Solving Hemodynamic Equipment Problems
Typical problems with bedside monitoring equipment and nursing measures to ensure patient safety and troubleshoot equipment problems are addressed in Table 11-3.
TABLE 11-3
PROBLEM | PREVENTION | RATIONALE | TROUBLESHOOTING |
Overdamping of waveform | Provide continuous infusion of solution containing heparin through an in-line flush device (1 unit of heparin for each 1 mL of flush solution). | Ensure that recorded pressures and waveform are accurate because a damped waveform gives inaccurate readings. | Before insertion, completely flush the line and/or catheter. In a line attached to a patient, back flush through the system to clear bubbles from tubing or transducer. |
Underdamping (“overshoot” or “fling”) | Use short lengths of noncompliant tubing. Use fast-flush square wave test to demonstrate optimal system damping. Verify arterial waveform accuracy with the cuff blood pressure. | If the monitoring system is underdamped, the systolic and diastolic values will be overestimated by the waveform and the digital values. False high systolic values may lead to clinical decisions based on erroneous data. | Perform the fast-flush square wave test to verify optimal damping of the monitoring system. |
Clot formation at end of the catheter | Provide continuous infusion of solution containing heparin through an in-line flush device (1 unit of heparin for each 1 mL of flush solution). | Any foreign object placed in the body can cause local activation of the patient’s coagulation system as a normal defense mechanism. The clots that are formed may be dangerous if they break off and travel to other parts of the body. | If a clot in the catheter is suspected because of a damped waveform or resistance to forward flush of the system, gently aspirate the line using a small syringe inserted into the proximal stopcock. Flush the line again after the clot is removed, and inspect the waveform. It should return to a normal pattern. |
Hemorrhage | Use Luer-lock (screw) connections in line setup. Close and cap stopcocks when not in use. Ensure that the catheter is sutured or securely taped in position. |
A loose connection or open stopcock creates a low-pressure sump effect, causing blood to back into the line and into the open air. If a catheter is accidentally removed, the vessel can bleed profusely, especially with an arterial line or if the patient has abnormal coagulation factors (resulting from heparin in the line) or has hypertension. |
After a blood leak is recognized, tighten all connections, flush the line, and estimate blood loss. If the catheter has been inadvertently removed, put pressure on the cannulation site. When bleeding has stopped, apply a sterile dressing, estimate blood loss, and inform the physician. If the patient is restless, an arm board may protect lines inserted in the arm. |
Air emboli | Ensure that all air bubbles are purged from a new line setup before attachment to an indwelling catheter. Ensure that the drip chamber from the bag of flush solution is more than one-half full before using the in-line, fast-flush system. Some sources recommend removing all air from the bag of flush solution before assembling the system. |
Air can be introduced at several times, including when central venous pressure (CVP) tubing comes apart, when a new line setup is attached, or when a new CVP or pulmonary artery (PA) line is inserted. During insertion of a CVP or PA line, the patient may be asked to hold his or her breath at specific times to prevent drawing air into the chest during inhalation. The in-line, fast-flush devices are designed to permit clearing of blood from the line after withdrawal of blood samples. If the chamber of the intravenous tubing is too low or empty, the rapid flow of fluid will create turbulence and cause flushing of air bubbles into the system and into the bloodstream. |
Because it is impossible to get the air back after it has been introduced into the bloodstream, prevention is the best cure. If air bubbles occur, they must be vented through the in-line stopcocks and the drip chamber must be filled. The left atrial pressure (LAP) line setup is the only system that includes an air filter specifically to prevent air emboli. |
Normal waveform with low digital pressure | Ensure that the system is calibrated to atmospheric pressure. Ensure that the transducer is placed at the level of the phlebostatic axis. |
To provide a 0 baseline relative to atmospheric pressure. If the transducer has been placed higher than the phlebostatic level, gravity and the lack of hydrostatic pressure will produce a false low reading. |
Recalibrate the equipment if transducer drift has occurred. Reposition the transducer at the level of the phlebostatic axis. Misplacement can occur if the patient moves from the bed to the chair or if the bed is placed in a Trendelenburg position. |
Normal waveform with high digital pressure | Ensure that the system is calibrated to atmospheric pressure. Ensure that the transducer is placed at the level of the phlebostatic axis. |
To provide a 0 baseline relative to atmospheric pressure. If the transducer has been placed lower than the phlebostatic level, the weight of hydrostatic pressure on the transducer will produce a false high reading. |
Recalibrate the equipment if transducer drift has occurred. Reposition the transducer at the level of the phlebostatic axis. This situation can occur if the head of the bed was raised and the transducer was not repositioned. Some centers require attachment of the transducer to the patient’s chest to avoid this problem. |
Loss of waveform | Always have the hemodynamic waveform monitored so that changes or loss can be quickly noted. | The catheter may be kinked, or a stopcock may be turned off. | Check the line setup to ensure that all stopcocks are turned in the correct position and that the tubing is not kinked. Sometimes, the catheter migrates against a vessel wall, and having the patient change position restores the waveform. |
Intraarterial Blood Pressure Monitoring
Indications
Intraarterial blood pressure monitoring is indicated for any major medical or surgical condition that compromises cardiac output (CO), tissue perfusion, or fluid volume status. The system is designed for continuous measurement of three blood pressure parameters: systole, diastole, and mean arterial blood pressure (MAP). The direct arterial access is helpful in the management of patients with acute respiratory failure who require frequent arterial blood gas measurements.
Catheters
The size of the catheter used is proportionate to the diameter of the cannulated artery. In small arteries—such as the radial and dorsalis pedis—a 20-gauge, 3.8-cm to 5.1-cm, nontapered catheter is used most often. If the larger femoral or axillary arteries are used, a 19- or 20-gauge, 16-cm catheter is used.
The catheter insertion is usually percutaneous, although the technique varies with vessel size. Catheters are most often inserted in the smaller arteries, using a “catheter-over-needle” unit in which the needle is used as a temporary guide for catheter placement. With this method, after the unit has been inserted into the artery, the needle is withdrawn, leaving the supple plastic catheter in place. Insertion of a catheter into a larger artery typically uses the Seldinger technique, which involves the following steps:
1. Entry into the artery using a needle
2. Passage of a supple guidewire through the needle into the artery
4. Passage of the catheter over the guidewire
5. Removal of the guidewire, leaving the catheter in the artery
Insertion and Allen Test.
Several major peripheral arteries are suitable for receiving a catheter and for long-term hemodynamic monitoring. The most frequently used site is the radial artery. The femoral artery is a larger vessel that is also frequently cannulated. Other smaller arterials such as the dorsalis-pedis, axillary, or brachial arteries are avoided if possible, and only used when other arterial access is unavailable.
The major advantage of the radial artery is the supply of collateral circulation to the hand provided by the ulnar artery through the palmar arch, in most of the population. Before radial artery cannulation, collateral circulation must be assessed by using Doppler flow or by the Allen test, according to institutional protocol.39–41 In the Allen test the radial and ulnar arteries are compressed simultaneously. The patient is asked to clench and unclench the hand until it blanches. One of the arteries is then released, and the hand should immediately flush from that side. The same procedure is repeated for the remaining artery. Bedside ultrasound is increasingly used to increase the accuracy of catheter placement. If the catheter is placed on the first pass, this is both safer and more comfortable for the patient. The use of ultrasound to identify the radial artery prior to cannulation has been shown to increase first-pass placement accuracy.42
Nursing Management
Nursing priorities for the patient with intraarterial monitoring focus on (1) assessing arterial perfusion pressures, (2) interpreting the accuracy of the arterial pressure waveform, and (3) troubleshooting arterial waveform problems.
Intraarterial blood pressure monitoring is designed for continuous assessment of arterial perfusion to the major organ systems of the body. MAP is the clinical parameter most often used to assess perfusion, because MAP represents perfusion pressure throughout the cardiac cycle. Because one third of the cardiac cycle is spent in systole and two thirds in diastole, the MAP calculation must reflect the greater amount of time spent in diastole. This MAP formula can be calculated by hand or with a calculator, where diastole times 2 plus systole is divided by 3 as shown in the formula below:
< ?xml:namespace prefix = "mml" />

A blood pressure of 120/60 mm Hg produces a MAP of 80 mm Hg. However, the bedside hemodynamic monitor may show a slightly different digital number because most computers calculate the area under the curve of the arterial line tracing (normal values listed in Table 11-4). The MAP represents an estimate of organ perfusion pressure.43
TABLE 11-4
HEMODYNAMIC PRESSURES AND CALCULATED HEMODYNAMIC VALUES
HEMODYNAMIC PRESSURE | DEFINITION AND EXPLANATION | NORMAL RANGE |
Mean arterial pressure (MAP) | Average perfusion pressure created by arterial blood pressure during the cardiac cycle. The normal cardiac cycle is one third systole and two thirds diastole. These three components are divided by 3 to obtain the average perfusion pressure for the whole cardiac cycle. | 70-100 mm Hg |
Central venous pressure (CVP) | Pressure created by volume in the right side of the heart. When the tricuspid valve is open, the CVP reflects filling pressures in the right ventricle. Clinically, the CVP is often used as a guide to overall fluid balance. | 2-5 mm Hg 3-8 cm water (H2O) |
Left atrial pressure (LAP) | Pressure created by the volume in the left side of the heart. When the mitral valve is open, the LAP reflects filling pressures in the left ventricle. Clinically, the LAP is used after cardiac surgery to determine how well the left ventricle is ejecting its volume. In general, the higher the LAP, the lower the ejection fraction from the left ventricle. | 5-12 mm Hg |
Pulmonary artery pressure (PAP) PA systolic (PAS) PA diastolic (PAD) PAP mean (PAPM) |
Pulsatile pressure in the pulmonary artery measured by an indwelling catheter. | PAS 20-30 mm Hg PAD 5-10 mm Hg PAPM 10-15 mm Hg |
Pulmonary artery occlusion pressure (PAOP)* | Pressure created by the volume in the left side of the heart. When the mitral valve is open, the PAOP reflects filling pressures in the pulmonary vasculature, and pressures in the left side of the heart are transmitted back to the catheter “wedged” into a small pulmonary arteriole. | 5-12 mm Hg |
Cardiac output (CO) | Amount of blood pumped out by a ventricle. Clinically, it can be measured using the thermodilution CO method, which calculates CO in liters per minute (L/min). | 4-6 L/min (at rest) |
Cardiac index (CI) | CO divided by the body surface area (BSA), with tailoring of CO to individual body size. A BSA conversion chart is necessary to calculate CI, which is considered more accurate than CO because it is individualized to height and weight. CI is measured in liters per minute per square meter of BSA (L/min/m2). | 2.2-4.0 L/min/m2 |
Stroke volume (SV) | Amount of blood ejected by the ventricle with each heartbeat. Hemodynamic monitoring systems calculate SV by dividing cardiac output (CO in L/min) by the heart rate (HR) and then multiplying the answer by 1000 to change liters to milliliters (mL). | 60-70 mL |
Stroke volume index (SI) | SV indexed to the BSA. | 40-50 mL/m2 |
Systemic vascular resistance (SVR) | Mean pressure difference across the systemic vascular bed divided by blood flow. Clinically, SVR represents the resistance against which the left ventricle must pump to eject its volume. This resistance is created by the systemic arteries and arterioles. As SVR increases, CO falls. SVR is measured in Wood units or dyn·sec·cm−5. If the number of Wood units is multiplied by 80, the value is converted to dyn·sec·cm−5. | 10-18 Wood units or 100-250 dyn·sec·cm−5 |
Systemic vascular resistance index (SVRI) | SVR indexed to BSA. | 2000-2400 dyn·sec·cm−5 |
Pulmonary vascular resistance (PVR) | Mean pressure difference across pulmonary vascular bed divided by blood flow. Clinically, PVR represents the resistance against which the right ventricle must pump to eject its volume. This resistance is created by the pulmonary arteries and arterioles. As PVR increases, the output from the right ventricle decreases. PVR is measured in Wood units or dyn·sec·cm−5. PVR is normally one sixth of SVR. | 1.2-3.0 Wood units or 100-250 dyn·sec·cm−5 |
Pulmonary vascular resistance index (PVRI) | PVR indexed to BSA. | 225-315 dyn·sec·cm−5/m2 |
*Pulmonary artery occlusion pressure (PAOP) was formerly called pulmonary capillary wedge pressure (PCW or PCWP) or pulmonary arterial wedge pressure (PAWP).
Assessing Arterial Perfusion Pressures.
A MAP greater than 60 mm Hg is necessary to perfuse the coronary arteries. A higher MAP may be required to perfuse the brain and the kidneys. A MAP between 70 and 90 mm Hg is ideal for the cardiac patient to decrease left ventricular (LV) workload. After a carotid endarterectomy or neurosurgery, a MAP of 90 to 110 mm Hg may be more appropriate to increase cerebral perfusion pressure. Systolic and diastolic pressures are monitored in conjunction with the MAP as a further guide to the accuracy of perfusion. If CO decreases, the body compensates by constricting peripheral vessels to maintain the blood pressure. In this situation, the MAP may remain constant but the pulse pressure (difference between systolic and diastolic pressures) narrows. The following examples explain this point:
Both patients have a perfusion pressure of 76 mm Hg, but they are clinically very different. Mr. A is peripherally vasoconstricted, as is demonstrated by the narrow pulse pressure (90/70 mm Hg). His skin is cool to the touch, and he has weak peripheral pulses. Mr. B has a wide pulse pressure (150/40 mm Hg), warm skin, and palpable peripheral pulses. Nursing assessment of the patient with an arterial line includes comparison of clinical findings with arterial line readings, including perfusion pressure and MAP.
Interpreting Arterial Pressure Waveforms.
As the aortic valve opens, blood is ejected from the left ventricle and is recorded as an increase of pressure in the arterial system. The highest point recorded is called systole. After peak ejection (systole), the ejection force decreases, and the pressure decreases as a result, as seen on the waveform. A notch (dicrotic notch) may be visible on the downstroke of the arterial waveform, representing closure of the aortic valve. The dicrotic notch signifies the beginning of diastole. The remainder of the downstroke represents diastolic runoff of blood flow into the arterial tree. The lowest point recorded is called diastole. A normal arterial pressure tracing is shown in Figure 11-8. Time is measured from left to right on the waveform tracing. Notice that electrical stimulation (QRS) always comes first, and that the arterial pressure tracing follows the initiating QRS. If the arterial line becomes unreliable or dislodged, a cuff pressure can be used as a reserve system.44
Troubleshooting Arterial Pressure Monitoring Problems.
Major complications associated with arterial pressure monitoring are rare. The most life-threatening risk is exsanguination if the Luer-Lock connections are not tight or if an in-line stopcock is inadvertently opened to air. Pressure monitor alarms must always be on, with alarm limits (high and low) set at a safe, audible warning range for each patient. When the arterial monitor displays a low blood pressure digital reading, it is a nursing responsibility to determine whether this is a true patient problem, or a problem with the monitoring equipment. A damped waveform occurs when communication from the artery to the transducer is interrupted and produces false values on the monitor and oscilloscope. Troubleshooting techniques are used to find the origin of the problem and to remove the cause of damping (see Table 11-3).
Fast-Flush Square Waveform Test.
The monitoring system’s dynamic response can be verified for accuracy at the bedside by the fast-flush square waveform test, also called the dynamic frequency response test.35 The nurse performs this test to ensure that the patient pressures and waveform shown on the bedside monitor are accurate.35 The test makes use of the manual flush system on the transducer. Normally, the flush device allows only 3 mL of fluid/hr. With the normal waveform displayed, the manual fast-flush procedure is used to generate a rapid increase in pressure, which is displayed on the monitor oscilloscope. As shown in Figure 11-9, the normal dynamic response waveform shows a square pattern with one or two oscillations before the return of the arterial waveform. If the system is overdamped, a sloped (rather than square) pattern is seen. If the system is underdamped, additional oscillations—or vibrations—are seen on the fast-flush square wave test. This test can be performed with any hemodynamic monitoring system. If air bubbles, clots, or kinks are in the system, the waveform becomes damped, or flattened, and this is reflected in the square waveform result.
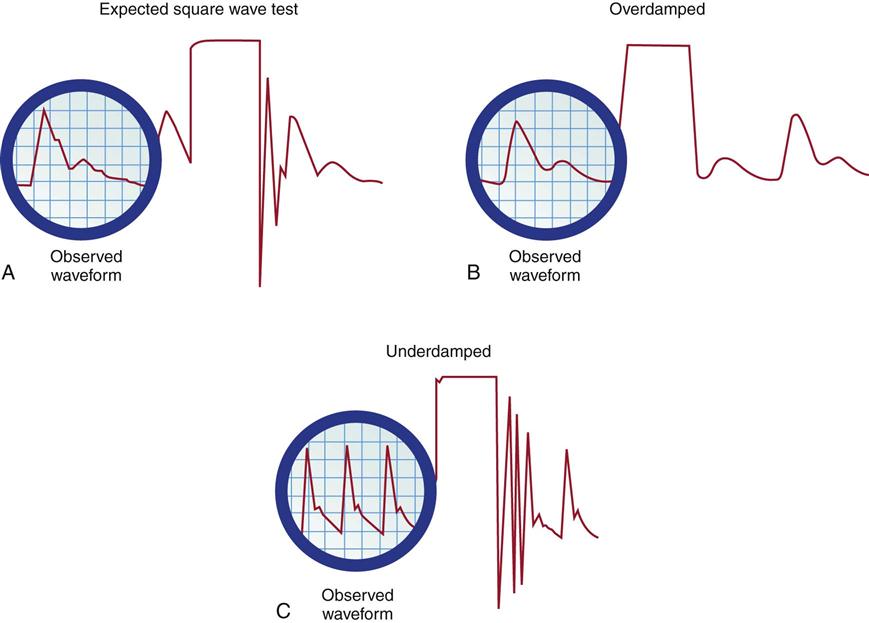
A, Expected square wave test result. B, Overdamped. C, Underdamped. (From Darovic GO: Hemodynamic monitoring: invasive and noninvasive clinical application, ed 3, Philadelphia, 2002, WB Saunders.)
This is an easy test to perform, and it should be incorporated into nursing care procedures at the bedside when the hemodynamic system is first set up, at least once per shift, after opening the system for any reason, and when there is concern about the accuracy of the waveform.35 If the pressure waveform is distorted or the digital display is inaccurate, the troubleshooting methods described in Table 11-3 can be implemented. The nurse caring for the patient with an arterial line must be able to assess whether a low MAP or narrowed perfusion pressure represents decreased arterial perfusion or equipment malfunction. Assessment of the arterial waveform on the oscilloscope, in combination with clinical assessment and use of the square waveform test, will yield the answer.
Central Venous Pressure Monitoring
CVP monitoring is indicated whenever a patient has significant alteration in fluid volume. The CVP can be used as a guide in fluid volume replacement in hypovolemia and to assess the impact of diuresis after diuretic administration in the case of fluid overload. When a major intravenous line is required for volume replacement, a central venous catheter (CVC) is a good choice because large volumes of fluid can easily be delivered.
A range of CVC options are available as single-, double-, triple-, and quad-lumen infusion catheters, depending on the specific needs of the patient. CVCs are made from a variety of materials ranging from polyurethane to silicone; most are soft and flexible. Many catheters incorporate an antimicrobial coating to reduce the risk of bloodstream infections.45
Insertion
The large veins of the upper thorax—subclavian (SC) and internal jugular (IJ)—are most commonly used for percutaneous CVC line insertion. The femoral vein in the groin is used when the thoracic veins are not accessible. All three major sites have advantages and disadvantages.
Internal Jugular Vein.
The IJ vein is the most frequently used access site for CVC insertion. Compared with the other thoracic veins, it is the easiest to canalize. If the IJ vein is not available, the external jugular (EJ) vein may be accessed, although blood flow is significantly higher in the IJ vein, making it the preferred site. Another advantage of the IJ vein is that the risk of creating an iatrogenic pneumothorax is small. Disadvantages to the IJ vein are patient discomfort from the indwelling catheter when moving the head or neck and contamination of the IJ vein site from oral or tracheal secretions, especially if the patient is intubated or has a tracheostomy. This may be the reason why catheter-related infections are higher in the IJ than the SC position for indwelling catheters left in place for more than 4 days.46,47
Subclavian Vein.
If the anticipated CVC dwelling time is prolonged more than 5 days, the SC site is preferred. The SC position has the lowest infection rate and produces the least patient discomfort from the catheter. The disadvantages are that the SC vein is more difficult to access and carries a higher risk of iatrogenic pneumothorax or hemothorax, although the risk varies greatly, depending on the experience and skill of the physician inserting the catheter.
Femoral Vein.
The femoral vein is considered the easiest cannulation site because there are no curves in the insertion route. The large diameter of the femoral vein carries a high blood flow that is advantageous for specialized procedures such as continuous renal replacement therapy (CRRT) or plasmapheresis. Disadvantages are that the patient cannot bend at the hip, because this interrupts blood flow through the catheter and may lead to thrombus formation; risk of retroperitoneal bleed; and a higher rate of nosocomial infections, probably due to site location near the groin area.46
During insertion of a catheter in the SC or IJ vein, the patient may be placed in a Trendelenburg position. Placing the head in a dependent position causes the IJ veins in the neck to become more prominent, facilitating line placement. To minimize the risk of air embolus during the procedure, the patient may be asked to “take a deep breath and hold it” any time the needle or catheter is open to air. The tip of the catheter is designed to remain in the vena cava and should not migrate into the right atrium. If the IJ or SC veins are not available, the femoral veins can be used for CVC access. The femoral veins are farther away from the heart; for accurate CVP measurements, the tip of the catheter must be advanced into the inferior vena cava near the right atrium. Because many patients are awake and alert when a CVC is inserted, a brief explanation about the procedure can minimize patient anxiety and result in cooperation during the insertion. This cooperation is important, because CVC insertion is a sterile procedure and because the supine or Trendelenburg position may not be comfortable for many patients. The electrocardiogram (ECG) should be monitored during CVC insertion because of the associated risk of dysrhythmias.
All central catheters are designed for placement by percutaneous injection after skin preparation and administration of a local anesthetic. A prepackaged CVC kit typically is used for the procedure. The standard CVC kit contains sterile towels, chlorhexidine and alcohol for skin preparation, a needle introducer, a syringe, guidewire, and a catheter. The Seldinger technique, in which the vein is located by using a “seeking” needle and syringe, is the preferred method of placement. A guidewire is passed through the needle, the needle is removed, and the catheter is passed over the guidewire. After the catheter is correctly placed in the vena cava, the guidewire is removed. A sterile intravenous tubing and solution is attached, and the catheter is sutured in place.
An important development in central venous catheter management is adherence to the Centers for Disease Control (CDC) and Institute for Healthcare Improvement (IHI) bundle approach to prevent blood stream infections. The CVC bundle places a strong emphasis upon infection control during insertion, to protect the patient. The IHI recommends meticulous hand hygiene, 2% chlorhexidine gluconate in 70% isopropyl patient skin preparation, full-barrier precautions for each CVC insertion, and optimal catheter site selection. In many hospitals the nurse is authorized to stop the procedure if these insertion infection control guidelines are not followed. A daily review to determine whether the catheter is still required is recommended to ensure CVCs are removed promptly when no longer needed.
After thoracic CVC placement, a chest radiograph is obtained to verify placement and the absence of an iatrogenic hemothorax or pneumothorax, especially if the SC vein was accessed. The use of Doppler ultrasound guidance to find the vein and guide insertion may reduce the incidence of iatrogenic complications.46 In the very rare case when it is not possible to insert a CVC percutaneously, a surgical cutdown may be performed.
Nursing Management
Nursing priorities for the patient with CVP monitoring focus on (1) preventing central venous catheter-associated complications, (2) assessing fluid volume status, (3) accommodating changes in patient position, and (4) accurately interpreting the CVP waveforms and pressures.
Preventing Central Venous Catheter-Associated Complications.
The CVC is an essential tool in care of the critically ill patient, but it is associated with some risks, and it is the responsibility of all clinicians to be informed about these hazards and to follow hospital procedures to avoid iatrogenic complications. CVC complications include air embolus, catheter-associated thrombus formation, and infection.
Air Embolus.
The risk of air embolus, although uncommon, is always present for the patient with a central venous line in place. Air can enter during insertion48 through a disconnected or broken catheter by means of an open stopcock,49 or air can enter along the path of a removed CVC.50 This is more likely if the patient is in an upright position, because air can be pulled into the venous system with the increase in negative intrathoracic pressure during inhalation. If a large volume of air is infused rapidly, it may become trapped in the right ventricular outflow tract, stopping blood flow from the right side of the heart to the lungs. Based on animal studies, this volume is approximately 4 mL/kg.51 If the air embolus is large, the patient will experience respiratory distress and cardiovascular collapse. An auscultatory clinical sign specifically associated with a large venous air embolism is a mill wheel murmur.49–51 A mill wheel murmur is a loud, churning sound heard over the middle chest, caused by the obstruction to right ventricular outflow. Treatment involves administering 100% oxygen and placing the patient on the left side with the head downward (left lateral Trendelenburg position).49 This position displaces the air from the right ventricular outflow tract to the apex of the heart, where the air may be aspirated by catheter intervention or gradually absorbed by the bloodstream as the patient remains in the left lateral Trendelenburg position. Precautions to prevent an air embolism in a CVP line include using only screw (Luer-Lock) connections, avoiding long loops of intravenous tubing, and using closed-top screw caps on the three-way stopcock.
Thrombus Formation.
Clot formation (thrombus) at the CVC site is unfortunately common. Ultrasound studies have found asymptomatic thrombus formation to be in the range of 33% to 67% when the catheter is in place for more than 7 days.46 Symptomatic thrombi are reported in 0% to 5% of those cases.46,47 Thrombus formation is not uniform; it may involve development of a fibrin sleeve around the catheter, or the thrombus may be attached directly to the vessel wall. Other factors that promote clot formation include rupture of vascular endothelium, interruption of laminar blood flow, and physical presence of the catheter, all of which activate the coagulation cascade. The risk of thrombus formation is higher if insertion was difficult or if there were multiple needlesticks.46 Gradual thrombus formation may lead to “sudden” CVC occlusion. Usually, the CVC becomes more difficult to withdraw blood from, or the CVP waveform becomes intermittently damped over a period of hours or even 1 to 2 days and is reported as “needing frequent flushes” to remain patent. This situation is caused by the continued lengthening of a fibrin sleeve that extends along the catheter length from the insertion site past the catheter tip.46,47 Some catheters are heparin coated to reduce the risk of thrombus formation, although the risk of HIT, reported to be 0.4% with indwelling CVC, does not make this a benign option.47 Sometimes, CVC complications are additive; for example, the risk of catheter-related infection is increased in the presence of thrombi. The thrombus likely serves as a culture medium for bacterial growth.47
Infection.
Infection related to the use of CVCs is a major problem. Risk factors for catheter-related infections include extremes of age, impaired host defense mechanisms, severe illness, malnutrition, and presence of other invasive lines. It is estimated that more than 50,000 infections related to CVC use occur annually in the United States, with associated mortality rates between 10% and 20%.47
The incidence of infection strongly correlates with the length of time the CVC has been inserted.52 Catheters that are in place up to 3 days rarely lead to infection, provided that standard insertion and management procedures are followed. If the CVC remains between 3 and 7 days, the infection rate is 3% to 5%. Catheters remaining in one site more than 7 days have an infection rate of 5% to 10%.47
CVC-related infection is identified at the catheter insertion site or as a bloodstream infection (septicemia). Systemic manifestations of infection can be present without inflammation at the catheter site. In order to determine whether a suspect catheter is contaminated, after removal the tip may be placed in a sterile container and cultured. No decrease in infections was found when catheters were routinely changed to prevent infection, and this practice is no longer recommended.53 A suspect CVC changed over a guidewire risks a higher rate of infection.52,53 Prevention is the best defense against complications resulting from infections. Most infections are transmitted from the skin, and infection prevention begins prior to insertion of the CVC. Insertion guidelines state that the physician must use effective hand-washing procedures, clean the insertion site with 2% chlorhexidine gluconate in 70% isopropyl, use sterile technique during catheter insertion, and maintain maximal sterile barrier precautions (Patient Safety Priorities box on Prevention of Central Venous Catheter-Related Bloodstream Infections).53
All clinicians must use good hand-washing technique and follow aseptic procedures during site care and any time the CVC system is entered to withdraw blood, give medications, or change tubing.53,54 The infusion of high-dextrose solutions such as total parental nutrition (TPN) may be associated with an increased risk of infection. Methods to lower TPN-related complications include use of a single-lumen CVC that is not accessed for other medications or laboratory samples.
Incidence of infection is higher with use of occlusive site dressings that do not allow removal of moisture. Transparent, breathable dressings that allow removal of skin moisture are recommended. The use of chlorhexidine gluconate-impregnated dressings to cover the insertion site has recently been shown to reduce CVC-related bloodstream infections.55 Additional improvements in catheter design may further reduce central line infection rates. Some catheters are impregnated with an antimicrobial substance53 or have a silver-impregnated, tissue-barrier cuff attached to the catheter. These catheters are designed to lower the rate of CVC infection.
Assessing Fluid Volume Status.
In the critically ill patient, the CVC is used to monitor CVP and waveform. The CVP catheter is used to measure the filling pressures of the right side of the heart. During diastole, when the tricuspid valve is open and blood is flowing from the right atrium to the right ventricle, the CVP accurately reflects right ventricular end-diastolic pressure (RVEDP). The normal CVP is 2 to 5 mm Hg (3 to 8 cm H2O).
Low Central Venous Pressure.
A low CVP often occurs in the hypovolemic patient and suggests that insufficient blood volume is in the ventricle at end-diastole to produce an adequate stroke volume. To maintain normal CO, the heart rate (HR) must increase. This HR increase produces the tachycardia often observed in hypovolemic states and increases myocardial oxygen demand.
The CVP is used in combination with the MAP and other clinical parameters to assess hemodynamic stability. In the hypovolemic patient, the CVP falls before a significant fall in MAP occurs, because peripheral vasoconstriction keeps the MAP normal. The CVP is an excellent early-warning system for the patient who is bleeding, vasodilating, receiving diuretics, or being rewarmed after cardiac surgery.
High Central Venous Pressure.
An elevated CVP occurs in cases of fluid overload. To circulate the excess blood volume, the heart must greatly increase its contractile force to move the large volume of blood. This increases the cardiac workload and increases myocardial oxygen consumption. The critical care nurse follows the trend of the CVP measurements to determine subsequent interventions for optimal fluid volume management.
Central Venous Pressure Limitations.
The CVP is not a reliable indicator of left ventricular dysfunction. LV dysfunction, which can occur after an acute myocardial infarction (MI), increases filling pressures on the left side of the heart. The CVP, because it measures RVEDP, remains normal until the increase in pressure from the left side of the heart is reflected back through the pulmonary vasculature to the right ventricle. In this situation, a pulmonary artery catheter that measures pressures on the left side of the heart is the monitoring method of choice. More information on PAP monitoring is provided later in this chapter.
Accommodating Changes in Patient Position.
To achieve accurate CVP measurements, the phlebostatic axis is used as a reference point on the body, and the transducer or water manometer zero must be level with this point. If the phlebostatic axis is used and the transducer or water manometer is correctly aligned, any head-of-bed position of up to 60 degrees may be used for CVP accurate readings for most patients.35 Elevating the head of the bed is especially helpful for the patient with respiratory or cardiac problems who cannot tolerate a flat position.
Interpreting the CVP Waveform.
The normal right atrial (CVP) waveform has three positive deflections—a, c, and v waves—that correspond to specific atrial events in the cardiac cycle (Figure 11-10). The a wave reflects atrial contraction and follows the P wave seen on the ECG. The downslope of this wave is called the x descent and represents atrial relaxation. The c wave reflects the bulging of the closed tricuspid valve into the right atrium during ventricular contraction; this wave is small and not always visible, but corresponds to the QRS-T interval on the ECG. The v wave represents atrial filling and increased pressure against the closed tricuspid valve in early diastole. The downslope of the v wave is named the y descent and represents the fall in pressure as the tricuspid valve opens and blood flows from the right atrium to the right ventricle.
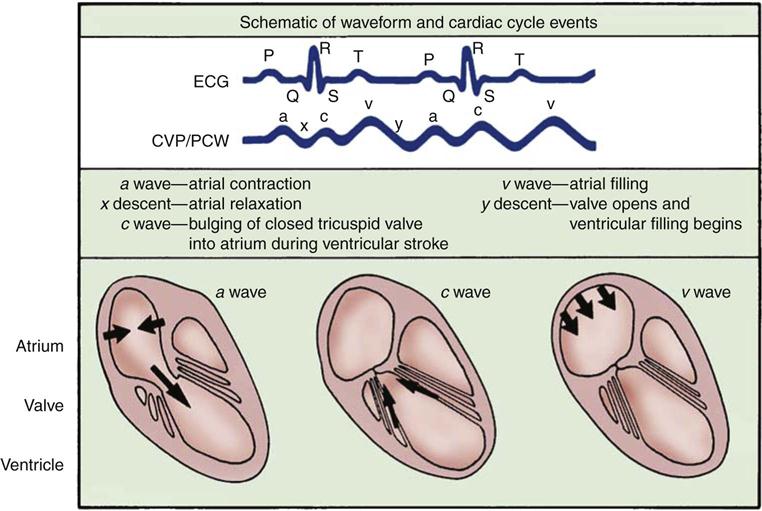
The a wave represents atrial contraction. The x descent represents atrial relaxation. The c wave represents the bulging of the closed tricuspid valve into the right atrium during ventricular systole. The v wave represents atrial filling. The y descent represents opening of the tricuspid valve and filling of the ventricle.
Cannon Waves.
Dysrhythmias can change the pattern of the CVP waveform. In a junctional rhythm or following a PVC, the atria are depolarized after the ventricles. When retrograde conduction to the atria occurs, this produces a retrograde P wave on the ECG and a large combined ac or cannon wave on the CVP waveform (Figure 11-11). Cannon waves are clearly visible as large pulses in the jugular veins, and correspond to the cannon waves on the CVP tracing.
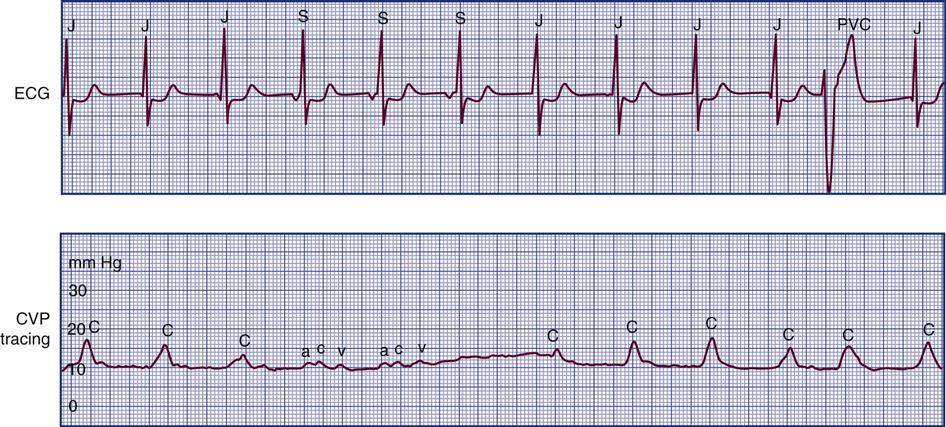
The CVP waveform shows large cannon waves (c waves) corresponding to the junctional beats or premature ventricular contractions (bottom strip). As the patient converts to sinus rhythm, the CVP waveform has a normal configuration. ac, normal right atrial pressure tracing; c, cannon waves on CVP tracing; J, junctional rhythm followed by cannon waves on CVP waveform; PVC, premature ventricular contraction followed by cannon wave on CVP; S, sinus rhythm followed by normal CVP tracing with a, c, and v waves.
Specialized Central Venous Oximetry Catheters.
A CVC that incorporates a fiberoptic sensor to continuously measure central venous oxygen saturation (SCVO2) can be used as a traditional CVC and additionally can be used to follow the trend of venous oxygen saturation.56,57 The physiology underlying use of this fiberoptic technology is discussed later in sections on monitoring mixed venous oxygen saturation (SvO2) and SCVO2.
Pulmonary Artery Pressure Monitoring
The pulmonary artery (PA) catheter is the most invasive of the critical care monitoring catheters. It is also known as a right heart catheter or Swan-Ganz catheter (named after the catheter’s inventors). The practice of routinely using PA catheters has been called into question and is highly controversial. Several randomized, controlled trials of critically ill patients have not demonstrated a benefit to use of the PA catheter. A randomized, controlled trial of 676 critical care patients with acute respiratory distress syndrome (ARDS) in France reported no difference in mortality rates for patients when treatment was guided by PA catheter and for patients without this information.58 A randomized, controlled trial of 1000 patients with acute lung injury in the United States found no difference in mortality rates for patients when treatment was guided by a PA catheter and those for whom diagnostic information was obtained from a CVP.59
The impact of PA catheterization on mortality rates for patients with acute heart failure has also been examined. A randomized, controlled trial of 433 patients with acute heart failure in the United States reported no difference in mortality based on whether fluid volume management was guided by PA catheter insertion or not.60 Similar results were reported from a British multicenter trial enrolling more than 1000 critical care patients; there were no differences in mortality or in length of stay.61,62 No survival benefit was found in a randomized, controlled trial enrolling older high-risk surgical patients who required critical care monitoring whether treatment was guided by PA catheter diagnostics or not.63 Systematic reviews and meta-analysis of studies of PA catheter use have reached similar conclusions—that insertion of a PA catheter is neutral, it neither conferred a benefit nor increased risk to the patient. There was no increase in mortality or increase in the number of days in the critical care unit or the hospital.64,65
These findings have raised concerns about routine use of PA catheters for critically ill patients. The PA catheter is invasive. It previously seemed intuitive that the diagnostic information provided would confer a survival advantage over less invasive methods, but research has shown this is not the case. In 2000 the number of PA catheters used in the United States was reported as 1.5 million annually; 30% were used in cardiac surgery units, 30% in cardiac catheterization laboratories and coronary care units, 25% in high-risk surgery and trauma units, and 15% in medical intensive care units.66 As a result of studies that document the failure of the PA catheter to lower mortality, the use of PA catheters has declined considerably. A study of PA catheterization between 1993 and 2004 reported a decline of 65% in PA catheter use in critically ill patients.67 Although PA catheter use has declined in critical care units, right heart catheterization remains a useful diagnostic tool in the cardiac catheterization laboratory. While the PA catheter has not been associated with increased harm, the lack of clear benefit mandates that its importance in patient care management will continue to decline. In many critical care units, the PA catheter is reserved only for patients who are refractory to conventional treatment,68 and increasingly, it will be replaced by less invasive technologies.
Pulmonary Artery Catheter Educational Resources
Clinicians who do not frequently work with PA catheters are less knowledgeable about waveform analysis and interpretation of data. With the decline in PA catheterization, this issue has become even more important. As a response to this concern, several professional organizations, including the American Association of Critical-Care Nurses (AACN), the Society of Critical Care Medicine (SCCM), and the American College of Chest Physicians (ACCP), endorse the Internet-based Pulmonary Artery Catheter Education Project (PACEP).69 This website (www.pacep.org)69 is free and is designed to provide education about PA hemodynamic monitoring. Another useful and free Internet-based resource is the Pulmonary Artery Catheter Primer from the American Thoracic Society.70
Indications
The thermodilution PA catheter is used for diagnosis and evaluation of heart disease, shock states, ARDS, and medical conditions that compromise CO or fluid volume status. The PA catheter can simultaneously assess pulmonary artery systolic and diastolic pressures, pulmonary artery mean pressure, and PAOP (wedge pressure). The PA catheter is used to measure CO, determine mixed-venous oxygen saturation, and calculate additional hemodynamic parameters.
Pulmonary Artery Catheters
The traditional PA catheter, invented by Swan and Ganz, has four lumens for measurement of right atrial pressure (RAP) or CVP, PA pressures, PAOP, and CO (Figure 11-12, A). Multifunction catheters may have additional lumens, which can be used for intravenous infusion (Figure 11-12, B) and to measure continuous mixed venous oxygen saturation (SvO2), right ventricular volume, and continuous CO (Figure 11-12, C). Other PA catheters include transvenous pacing electrodes to pace the heart if needed.
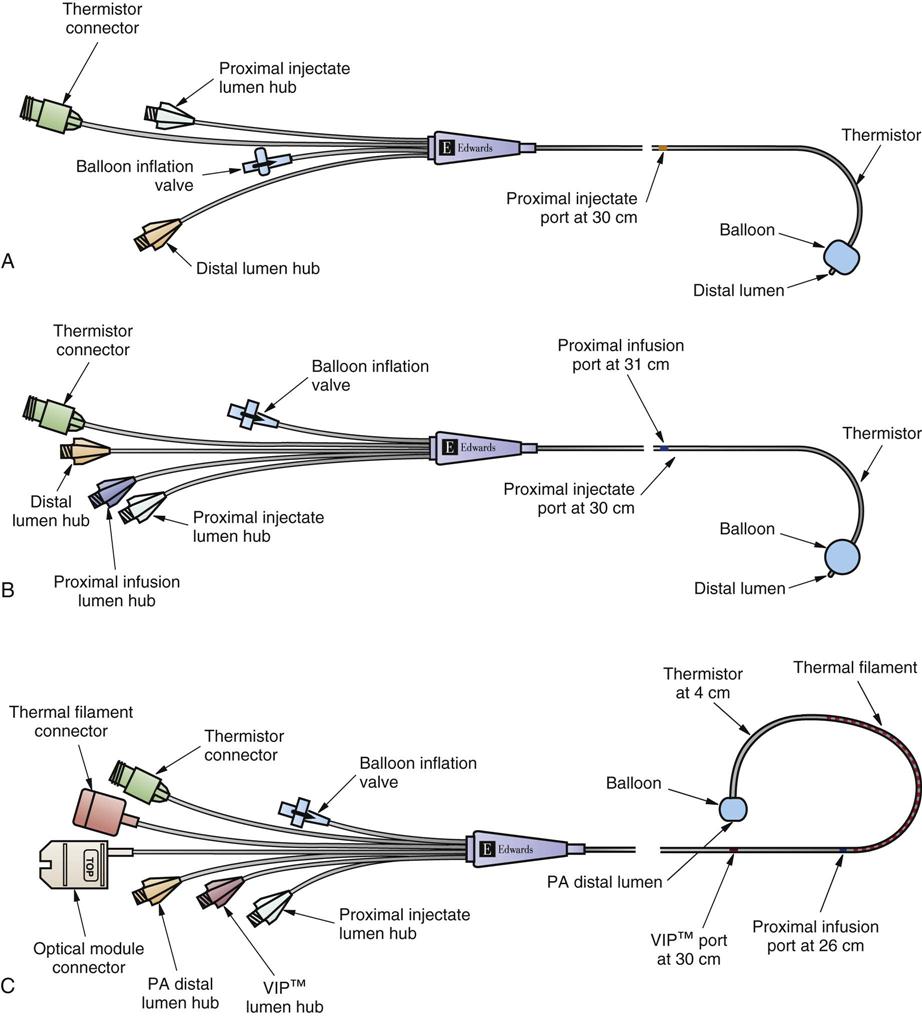
A, Four-lumen catheter. B, Five-lumen catheter that includes an additional venous infusion port (VIP) into the right atrium. C, Seven-lumen catheter that includes a VIP port and two additional lumens for continuous cardiac output (CCO) and a thermal filament and for continuous mixed venous oxygen saturation (SvO2) monitoring (i.e., optical module connector). An additional option is to combine the use of the CCO filament and the thermistor response time to calculate continuous end-diastolic volume (CEDV). (©2001 Edwards Lifesciences LLC. All rights reserved. Reprinted with permission of Edwards Lifesciences, Swan-Ganz is a trademark of Edwards Lifesciences Corporation, registered with the U.S. Patent and Trademark Office.)
The PA flow-directed catheter is 110 cm long. The most commonly used size is 7.5 or 8.0 Fr, although 5.0 and 7.0 Fr sizes are available. Each of the four lumens exits into the heart or pulmonary artery at a different point, graduated along the catheter length (see Figure 11-12, A).
Right Atrial Lumen.
The proximal lumen is situated in the right atrium and is used for intravenous infusion, CVP measurement, withdrawal of venous blood samples, and injection of fluid for CO determinations. This port is often described as the right atrial port, also called the CVP port.
Pulmonary Artery Lumen.
The distal PA lumen is located at the tip of the PA catheter and is situated in the pulmonary artery. It is used to record pulmonary artery pressures and can be used for withdrawal of blood samples to measure mixed-venous blood gases (e.g., SvO2).
Balloon Lumen.
The third lumen opens into a balloon at the end of the catheter that can be inflated with 0.8 mL (7 Fr) to 1.5 mL (7.5 Fr) of air. The balloon is inflated during catheter insertion after the catheter reaches the right atrium to assist in forward flow of the catheter and to minimize right ventricular ectopy from the catheter tip. The balloon is also inflated to obtain the PAOP measurements when the PA catheter is correctly positioned in the pulmonary artery.
Thermistor Lumen.
The fourth lumen is a thermistor (temperature sensor) used to measure changes in blood temperature. It is located 4 cm from the catheter tip and is used to measure thermodilution CO. The connector end of the lumen is attached directly to the CO computer.
Additional Features.
If continuous SvO2 is measured, the catheter has an additional fiberoptic lumen that exits at the tip of the catheter (see Figure 11-12, C). If cardiac pacing is used, two PA catheter methods are available. One type of catheter has three atrial (A) and two ventricular (V) pacing electrodes attached to the catheter so that when it is properly positioned, the patient can be connected to a pacemaker and be AV paced. The other catheter method uses a specific transvenous pacing wire that is passed through an additional catheter lumen and exits into the right ventricle if ventricular pacing is required. A right ventricular volumetric PA catheter is available that measures stroke volume in the right ventricle.
Insertion
If a PA catheter is to be inserted into a patient who is awake, some brief explanations about the procedure are helpful to ensure that the patient understands what is going to happen. The initial insertion techniques used for placement of a PA catheter are similar to those described for CVC insertion. Because the PA catheter is positioned within the heart chambers and pulmonary artery on the right side of the heart, catheter passage is monitored using fluoroscopy or waveform analysis on the bedside monitor (Figures 11-1 and 11-13).
Before inserting the catheter into the vein, the physician—using sterile technique—tests the balloon for inflation and flushes the catheter with normal saline solution to remove any air. The PA catheter is then attached to the bedside hemodynamic line setup and monitor so that the waveforms can be visualized while the catheter is advanced through the right side of the heart (see Figure 11-13). A larger introducer sheath (8.5 Fr)—which has the tip positioned in the vena cava and has an additional intravenous side-port lumen—is often used to cannulate the vein first.69 This introducer sheath is known by several different names in clinical practice, including sheath, cordis, introducer, or side port. This introducer sheath remains in place, and the supple PA catheter is threaded through it into the vena cava and into the right side of the heart.
Nursing Management
Nursing priorities for the patient with PA catheter monitoring focus on (1) accurately interpreting PA waveforms, (2) accommodating changes in patient position, (3) recognizing the effects of respiratory variation, (4) preventing PA catheter-related complications, (5) measuring cardiac output, and (6) evaluating hemodynamic performance.
Accurately Interpreting Pulmonary Artery Waveforms.
Each chamber of the heart has a distinctive waveform with recognizable characteristics. It is the responsibility of the critical care nurse to recognize each waveform displayed on the bedside monitor when the catheter enters the corresponding chamber during insertion and during routine monitoring.71
Right Atrial Waveform.
As the PA catheter is advanced into the right atrium during insertion, a right atrial waveform must be visible on the monitor, with recognizable a, c, and v waves (see Figure 11-13). The normal mean pressure in the right atrium is 2 to 5 mm Hg. Before passage through the tricuspid valve, the balloon at the tip of the catheter is inflated for two reasons. First, it cushions the pointed tip of the PA catheter so that if the tip comes into contact with the right ventricular wall, it will cause less myocardial irritability and, consequently, fewer ventricular dysrhythmias. Second, inflation of the balloon assists the catheter to float with the flow of blood from the right ventricle into the pulmonary artery. It is because of these features and the balloon that PA catheters are described as flow-directional catheters.
Right Ventricular Waveform.
The right ventricular waveform is distinctly pulsatile, with distinct systolic and diastolic pressures. Normal right ventricular pressures are 20 to 30 mm Hg systolic and 0 to 5 mm Hg diastolic. Even with the balloon inflated, it is not uncommon for some ventricular ectopy to occur during passage through the right ventricle. All patients who have a PA catheter inserted must have simultaneous ECG monitoring, with defibrillator and emergency resuscitation equipment nearby.
Pulmonary Artery Waveform.
As the catheter enters the pulmonary artery, the waveform again changes. The diastolic pressure rises. Normal PA pressures range from 20 to 30 mm Hg systolic over 10 mm Hg diastolic. A dicrotic notch, visible on the downslope of the waveform, represents closure of the pulmonic valve.
Pulmonary Artery Occlusion Waveform (Wedge).
While the balloon remains inflated, the catheter is advanced into the wedge position. This maneuver produces the PAOP. The waveform decreases in size and is nonpulsatile, reflecting a normal left atrial tracing with a and v wave deflections. This is known as a wedge tracing, because the balloon is “wedged” into a small pulmonary vessel, but it is technically described as the PAOP (see Figure 11-13). The balloon occludes a small pulmonary vessel so that the PA catheter tip and lumen are protected from the pulsatile influence of the pulmonary artery, and are exposed only to the left ventricular end-diastolic pressure (LVEDP). The relationship of the PA catheter tip to the left ventricle is shown in Figure 11-14. When the balloon is deflated, the catheter should spontaneously float back into the PA. When the balloon is reinflated, the wedge tracing should be visible. The normal PAOP ranges from 5 to 12 mm Hg.
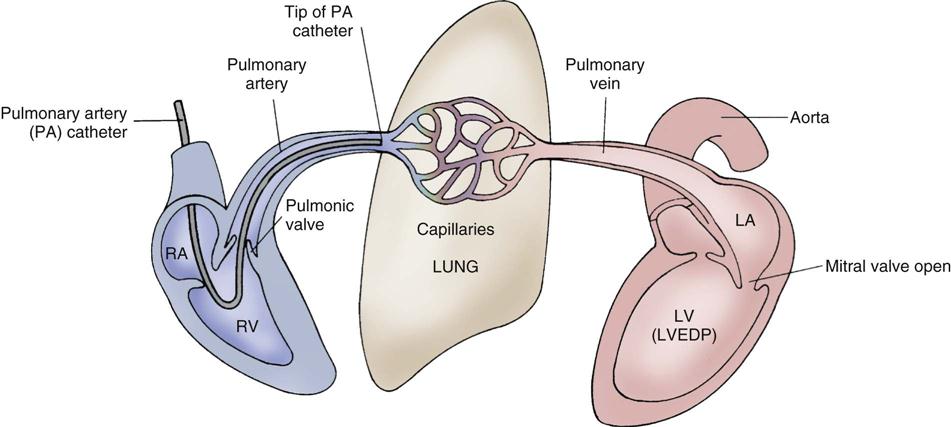
In most clinical situations, the PAOP accurately reflects the LVEDP. During diastole, when the mitral valve is open, there are no other valves or other obstructions between the tip of the catheter and the left ventricle (LV). The pressure exerted by the volume in the LV is reflected through the left atrium (LA), through the pulmonary veins, and to the pulmonary capillaries. PA, pulmonary artery; RA, right atrium; RV, right ventricle.
After insertion, the introducer is sutured to the skin, and the catheter, which lies within the introducer, is secured with tape or with a specialized catheter securement device. A chest radiograph is taken to verify placement. If the catheter is advanced too far into the pulmonary bed, the patient is at risk for pulmonary infarction. If the PA catheter is not sufficiently advanced into the pulmonary artery, it will not be useful for PAOP readings. However, in many critical care units, if the patient’s PADP and PAOP values approximate (within 0 to 3 mm Hg), the PADP is reliably used to follow the trend of LV filling pressure (preload). This prevents possible trauma from frequent balloon inflation; in such a situation, the PA catheter is consciously pulled back into the pulmonary artery, so that it is impossible to achieve an occlusion (wedged) tracing.
After insertion of the catheter, the chest radiograph or fluoroscopy is used to verify the PA catheter position to make sure that it is not looped or knotted in the right ventricle and to rule out pneumothorax or hemorrhagic complications. A thin plastic cuff can be placed on the outside of the catheter when it is inserted to maintain sterility of the part of the PA catheter that exits from the patient. If the PA catheter is not in the desired position or if it migrates out of position, it can be repositioned. The plastic cuff is designed to keep the external catheter sterile for a short period after insertion.
Accommodating Changes in Patient Position.
The patient does not need to be flat for accurate pressure readings to be obtained. In the supine position, when the transducer is placed at the level of the phlebostatic axis, a head-of-bed position from flat up to 60 degrees is appropriate for most patients.35 It is important to know that PADP and PAOP measurements in the lateral position may be significantly different from those taken when the patient is lying supine. If there is concern about the validity of pressure readings in a particular patient, it is more reliable to take measurements with the patient on his or her back, with the head of the bed elevated from flat to 60 degrees as tolerated. After a patient changes position, a stabilization period of 5 minutes is recommended before taking pressure readings if the patient has a healthy heart (normal left ventricle), and a stabilization period of 15 minutes is recommended if the patient has LV dysfunction.72
Recognizing the Effects of Respiratory Variation.
All PADP and PAOP (wedge) tracings are subject to respiratory interference, especially when the patient is on a positive-pressure, volume-cycled ventilator.35,71 During the positive-pressure inhalation phase, the increase in intrathoracic pressure may “push up” the pulmonary artery tracing, producing an artificially high reading (Figure 11-15, A). During inhalation with spontaneous breaths, negative intrathoracic pressure “pulls down” the waveform, producing an erroneously low measurement (see Figure 11-15, B). To minimize the impact of respiratory variation, the PADP is read at end-expiration, which is the most stable point in the respiratory cycle when intrapleural pressures are close to zero.71 If the digital number fluctuates with respiration, a printed readout on paper can be obtained to verify true PADP. In some clinical settings, ECG signals or airway pressure and flow are recorded simultaneously with the PADP/PAOP tracing to identify end-expiration.35,73
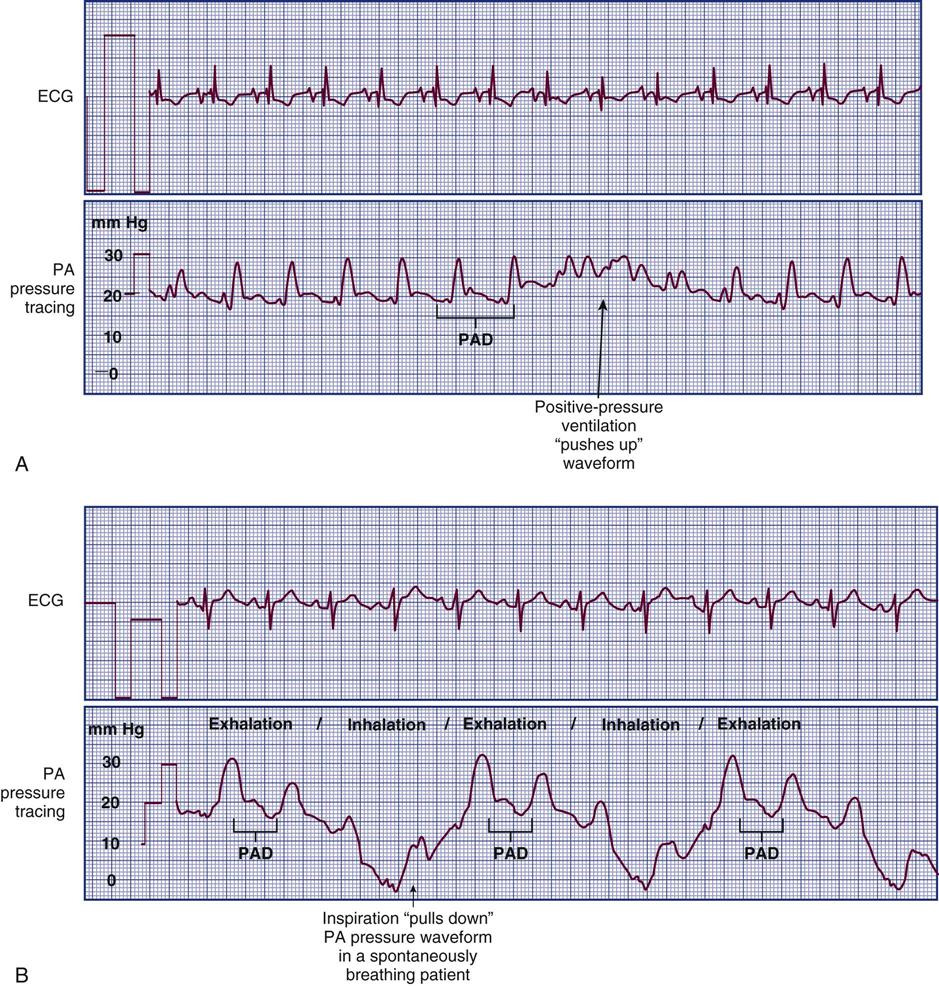
For accuracy, PA pressures are read at the end of exhalation. A, In positive-pressure ventilation, the increase in intrathoracic pressure during inhalation “pushes up” the PA pressure waveform, creating a falsely high reading. B, In spontaneous breathing, the decrease in intrathoracic pressure during normal inhalation “pulls down” the PA waveform, creating a falsely low reading.
Positive End-Expiratory Pressure.
Some clinical diagnoses, such as ARDS, require the use of high levels of PEEP set with the ventilator to treat refractory hypoxemia. If a PEEP of greater than 10 cm H2O is used, PAOP (wedge) and pulmonary artery pressures will be artificially elevated, and CO may be negatively affected.74,75 Because of this impact of PEEP, in the past, patients in some critical care units were taken off the ventilator to record pulmonary artery pressure measurements. It has since been shown that this practice closes alveoli, decreases the patient’s oxygenation level, and may result in persistent hypoxemia.
Because patients remain on PEEP for treatment, they remain on it during measurement of pulmonary artery pressures. In this situation, the trend of pulmonary artery readings is more important than one individual measurement. The most important factor is not one individual measurement or the absolute number obtained; it is instead the trend of the measurements being used as a basis for clinical interventions to support and improve cardiopulmonary function in the critically ill.
Preventing PA Catheter-Related Complications.
Potential cardiac complications include ventricular dysrhythmias, endocarditis, valvular damage, cardiac rupture, and cardiac tamponade. Potential pulmonary complications include rupture of a pulmonary artery, pulmonary artery thrombosis, embolism or hemorrhage, and infarction of a segment of lung. The PA catheter tracing is continuously monitored to ensure that the catheter does not migrate forward into a spontaneous wedge or PAOP position. A segment of lung can suffer infarction if the wedged catheter occludes an arteriole for a prolonged period. If the catheter is spontaneously wedged, the critical care nurse can gently pull the catheter back out of the wedge position if the institutional policy allows.76
Infection is always a risk with a PA catheter. The risks are similar to those discussed in the section on CVCs (Patient Safety Priorities box on Prevention of Central Venous Catheter-Related Bloodstream Infections on page 137).
Removal of the Pulmonary Artery Catheter.
PA catheters can be safely removed from the patient by critical care nurses competent in this procedure.35,76,77 Removal is not usually associated with major complications. The most common incidents are PVCs in about 2% of patients as the catheter is pulled through the right ventricle.35,77,78
Monitoring Cardiac Output.
Cardiac output (CO) is the product of heart rate (HR) multiplied by stroke volume (SV). Stroke volume is the volume of blood ejected by the heart during each beat (reported in milliliters).

The normal adult stroke volume is 60 to 70 mL. The clinical factors that contribute to the heart’s stroke volume are preload, afterload, and contractility (Figure 11-16). Preload and afterload are assessed using data from the PA catheter. Heart rate is recorded from the ECG leads.
The PA catheter measures CO using an intermittent (bolus) or a continuous CO method.
Thermodilution Cardiac Output Bolus Method.
The bolus thermodilution method is performed at the bedside and results in CO calculated in liters per minute. Three CO values that are within a 10% mean range are obtained at one time and are averaged to calculate CO. A known amount (5 mL) of iced or, more typically, 10 mL of room-temperature normal saline solution is injected into the proximal lumen of the PA catheter. The injectate exits into the right atrium and travels with the flow of blood past the thermistor (temperature sensor) located at the distal end of the catheter in the pulmonary artery. The injectate can be delivered by hand injection using individual syringes of saline. Frequently, a closed in-line system attached to a 500-mL bag of normal saline is used as a reservoir to deliver the individual injections.79
Sometimes, the right atrial (proximal) port is clotted and not usable. If another right atrial port is available, it can be substituted. However, if a usable port is not available, to ensure accurate CO data, a new pulmonary artery catheter is inserted.
Cardiac Output Curve Interpretation.
The thermodilution CO method uses the indicator-dilution method, in which a known temperature is the indicator. It is based on the principle that the change in temperature over time is inversely proportional to blood flow. Blood flow can be diagrammatically represented as a CO curve on which temperature is plotted against time (Figure 11-17). Most hemodynamic monitors display this CO curve, which must then be interpreted to determine whether the CO injection is valid. The normal curve has a smooth upstroke, with a rounded peak and a gradually tapering downslope. If the curve has an uneven pattern, it may indicate faulty injection technique, and the CO measurement must be repeated. Patient movement or coughing also alters the CO measurement.
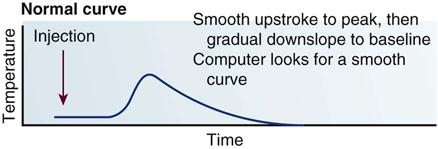
Injectate Temperature.
If the CO is within the normal range, and the patient has a normal body temperature, both iced and room temperature injectate are equally accurate.80 To ensure accurate CO values, the difference between injectate temperature and body temperature must be at least 10° C.81 If the patient is hypothermic, iced injectate may be needed to achieve this 10 degree difference. The injectate must be delivered within 4 seconds, with minimal handling of the syringe to prevent warming of the solution. With all delivery systems, the injectate is delivered at the same point in the respiratory cycle, usually end-exhalation.
Patient Position and Cardiac Output.
In the normovolemic, stable patient, reliable CO measurements can be obtained in a supine position (patient lying on his or her back) with the head of the bed elevated up to 45 degrees.82 If the patient is hypovolemic or unstable, leaving the head of the bed in a flat position or only slightly elevated is the most clinically appropriate choice. CO measurements performed when the patient is turned to the side are not considered as accurate as those performed with the patient in the supine position.
Clinical Conditions That Alter Cardiac Output.
Two clinical conditions produce errors in the thermodilution CO measurement: tricuspid valve regurgitation and ventricular septal rupture. If the patient has tricuspid valve regurgitation, the expected flow of blood from the right atrium to the pulmonary artery is disrupted by backflow from the right ventricle to the right atrium. This creates a lower CO measurement than the patient’s actual output. If the person has an intracardiac left-to-right shunt, as occurs after ventricular septal rupture, the thermodilution CO measures the large pulmonary volume and records a higher CO than the patient’s true systemic output.
Continuous Cardiac Output Measurement Using the PA Catheter.
The bolus thermodilution method is reliable but performed intermittently. Continuous CO monitoring using a PA catheter is also frequently used in clinical practice. One method employs a thermal filament on the PA catheter to emit small energy signals (the indicator) into the bloodstream. These signals are then detected by the thermistor near the tip of the PA catheter. The equivalent of an indicator curve is created, and a CO value is calculated from this data.
Noninvasive and Minimally Invasive Measurement of Cardiac Output.
As a result of concerns over use of the pulmonary artery thermodilution catheter, combined with studies that have not shown improved outcomes with routine monitoring, there is tremendous interest in using less-invasive methods of CO measurement. Evolving techniques are less invasive than the PA catheter, some are noninvasive, and others are minimally invasive and involve vascular catheters or esophageal probes as described in Table 11-5.
TABLE 11-5
NONINVASIVE AND MINIMALLY INVASIVE CARDIAC OUTPUT MEASUREMENT TECHNIQUES
DEVICE NAME | PROBE PLACEMENT | METHOD | CARDIAC OUTPUT CALCULATION | CLINICAL ISSUES |
Noninvasive Methods | ||||
Bioimpedance | External electrodes placed on the neck and chest | Thoracic electric bioimpedance | Noninvasive Less accurate with low body temperature. |
|
Minimally Invasive Methods | ||||
Pulse Contour Waveform Methods | ||||
LiDCO (LiDCO Ltd., Cambridge, United Kingdom) | Requires a venous access catheter (central or peripheral) and an arterial catheter with a lithium-monitoring sensor attached | Pulse contour waveform analysis method (calibrated) |
Independent calibration with a lithium dilution technique is initially required: 1. A small, subtherapeutic dose of isotonic lithium chloride is injected through the venous catheter. 3. A concentration-time curve is produced for lithium before recirculation. 4. CO is calculated based on the lithium dose given and the measurement of area under the curve. |
Easy to set up, uses conventional venous and arterial catheters; can measure extravascular lung water for patients in pulmonary edema. CO measurement is affected by artifact on arterial waveform and by irregular and damped arterial waveforms; can be used in conscious and in unresponsive patients. Requires calibration at least every 8 hours to maintain accuracy; cannot be used in patients on lithium therapy because this interferes with the calibration. |
PiCCO (Pulsion Medical Systems, Munich, Germany) | Requires a central venous access catheter and uses a specialized arterial thermistor-tipped catheter in the femoral artery | Pulse contour waveform analysis method that uses transpulmonary thermodilution | CO measurement is affected by artifact on arterial waveform and irregular arterial waveforms. Three calibrations are required initially, and frequent recalibration is required to maintain accuracy. |
|
Vigileo (Edwards Lifesciences, Irvine, CA) | Requires a functional arterial catheter | Pulse contour waveform analysis method (does not require calibration) | Does not require external calibration but requires zeroing of the transducer. Lack of calibration procedures is controversial. |
|
Esophageal Probe Methods | ||||
Esophageal Doppler | Ultrasound probe placed in the lower esophagus | Stroke volume is calculated by measurement of the aortic blood velocity in the descending thoracic aorta (by continuous wave Doppler) plus calculation of the cross-sectional area of the aorta; these values are used to calculate the CO. | Useful in the operating room or with deeply sedated patients. Probe is stiff, and placement is not well tolerated by conscious patients. |
|
Transesophageal echocardiography (TEE) | Ultrasound probe placed in the esophagus | Probe placement allows imaging of the left ventricular outflow tract. Stroke volume is measured by Doppler. | Useful in the operating room or with deeply sedated patients. Probe is stiff, and placement is not well tolerated by conscious patients. Requires skill to accurately position probe to visualize the LV outflow tract. |
|
Partial CO2 Rebreathing Method | ||||
NICO 2 (Philips, Respironics) | Addition of a partial CO2 rebreathing circuit to ventilator | Partial CO2 rebreathing method | Can only be used in intubated and ventilated patients. Specialized additional tubing setup on ventilator. Cannot be used in patients who cannot tolerate hypercapnia (elevated CO2). CO measurement is altered by intrapulmonary shunt. |
CO, cardiac output; CO2, carbon dioxide; HR, heart rate; LV, left ventricle; SV, stroke volume.
Evaluating Hemodynamic Profiles.
For the patient with a thermodilution PA catheter in place, additional hemodynamic information can be calculated using routine vital signs, CO, and body surface area (BSA). These measurements are calculated using specific formulas that are indexed to a patient’s body size. The BSA is calculated when the height and weight are entered into the hemodynamic bedside monitor.
Continuous Monitoring of Venous Oxygen Saturation
Indications
Continuous monitoring of venous oxygen saturation is indicated for the critically ill patient who has the potential to develop an imbalance between oxygen supply and metabolic tissue demand. This includes patients after high-risk surgery,57 and in severe sepsis or septic shock.83,84
Continuous venous oxygen monitoring permits a calculation of the balance achieved between arterial oxygen supply (SaO2) and oxygen demand at the tissue level by sampling desaturated venous blood from the pulmonary artery catheter distal tip. This sample is called mixed venous oxygen saturation (SvO2) because it is a mixture of all of the venous blood drained from many body tissues. The same fiberoptic technology has been used in combination with a fiberoptic triple-lumen CVC. In this situation, the venous blood is sampled from the superior vena cava, just above the right atrium, and the central venous oxygen saturation (SCVO2) is measured.
Under normal conditions, the cardiopulmonary system achieves a balance between oxygen supply and demand. Four factors contribute to this balance:
Three of these factors (CO, Hgb, and SaO2) contribute to the supply of oxygen to the tissues. Tissue metabolism (VO2) determines oxygen consumption or the quantity of oxygen extracted at tissue level that creates the demand for oxygen. The relationships of these factors are illustrated in Figure 11-18.
In addition to measurement of venous oxygen saturation, it is possible to calculate the quantity of oxygen (in mL/min) that is provided to the tissues by the cardiopulmonary system and to assess the amount of oxygen consumed by the body tissues. These calculations rely on principles of oxygen transport physiology and are the basis for calculation of SvO2. These formulas are listed in Appendix B.
Catheters
The type of catheter used to measure venous oxygen saturation is defined by where the fiberoptic tip is located, either at the tip of a CVC or on the distal tip of a PA catheter.
SVO2 Catheter.
The pulmonary arterial SVO2 catheter has the traditional four lumens plus a lumen containing two or three optical fibers. The fiberoptics are attached to an optical module that is connected to a small bedside computer. The optical module transmits a narrow band of light down one optical fiber. This light is reflected off the hemoglobin in the blood and returns to the optical module through the receiving fiberoptic. The SvO2 signal is recorded on a continuous display.
SCVO2 Catheter.
The central venous SCVO2 technology is incorporated into a multi-lumen CVC. The fiberoptic catheter tip is positioned in a central vein, such as the superior vena cava. The technology used to measure the venous saturation is identical in both types of catheters, and the same continuous display module is used for both catheters.
The SCVO2 catheter has been used successfully to guide hemodynamic fluid resuscitation in septic patients.83,84 The relationship between the values obtained from the traditional PA (SvO2) catheter and the central venous (SCVO2) catheter are very similar, although the SCVO2 values are slightly higher. The trend of parallel measurements (up or down as patient condition changes) is in the same direction in about 90% of cases.
SVO2 or SCVO2 Catheter Calibration.
The catheter is calibrated before insertion into the patient through a standardized color reference system, which is part of the catheter package. Insertion technique and sites are identical to those used for placement of conventional PA or CVC catheters. Waveform analysis or venous saturation measurement, or both, can be used for accurate placement. After the catheter is inserted, recalibration is unnecessary unless the catheter becomes disconnected from the optical module.
To recalibrate the fiberoptic module to verify accuracy when the catheter is already inserted in a patient, a mixed venous blood sample (SvO2) or central venous sample (SCVO2) must be withdrawn from the appropriate catheter tip and sent to the laboratory for oxygen saturation analysis. In many critical care units, this is a standard daily procedure to ensure that readings used to guide patient care remain accurate.85
Nursing Management
Nursing priorities for the patient with a catheter that monitors venous oxygenation include: (1) assessing the accuracy of the SVO2/SCVO2 values, and (2) incorporating the SVO2/SCVO2 values into the hemodynamic assessment.
Assessing the Accuracy of the SvO2 or SCVO2 Values.
SvO2 monitoring provides a continuous assessment of the balance of oxygen supply and demand for an individual patient. Nursing assessment includes evaluation of the SvO2 or SCVO2 value and evaluation of the four factors that maintain the oxygen supply-demand balance. These factors are: arterial oxygen saturation (SaO2, or SpO2), cardiac output (CO), hemoglobin (Hgb), and tissue oxygenation (VO2), to maintain the oxygen supply-demand balance.
Normal SVO2 Values.
Normal SvO2 is approximately 75% in the healthy individual (range, 60% to 80%). In critically ill patients, an SvO2 value between 60% and 80% is evidence of adequate balance between oxygen supply and demand.
Normal SCVO2 Values.
The normal values for the SCVO2 catheter are slightly higher,56 because the reading is taken before the blood enters the right heart chambers, where the cardiac sinus (vein) delivers venous blood drained from the myocardium into the right atrium. The heavily desaturated myocardial blood decreases the oxygen saturation slightly. For this reason, SvO2 values are always slightly lower than SCVO2 readings in the same patient.56
If SvO2 or SCVO2 is within the normal range of 60% to 80% and the patient is not clinically compromised, the nurse can assume that oxygen supply and demand are balanced for that individual.
Assessment of SVO2 or SCVO2.
If SvO2 or SCVO2 falls below 60% and is sustained, the clinician must assume that oxygen supply is not equal to demand (Table 11-6). It is helpful to assess the cause of decreased SvO2 or SCVO2 in a logical sequence that reflects knowledge of the meaning of the venous saturation value. The following is one such assessment sequence:
TABLE 11-6
ALTERATIONS IN OXYGEN CONSUMPTION, IDENTIFIED FROM MIXED VENOUS OXYGEN SATURATION MONITORING
CONDITION OR ACTIVITY | % INCREASE OVER RESTING ![]() |
% DECREASE UNDER RESTING ![]() |
Clinical Conditions That Increase ![]() |
||
Fever | 10% (for each 1° C above normal) | |
Skeletal injuries | 10%-30% | |
Work of breathing | 40% | |
Severe infection | 60% | |
Shivering | 50%-100% | |
Burns | 100% | |
Routine postoperative procedures | 7% | |
Nasal intubation | 25%-40% | |
Endotracheal tube suctioning | 27% | |
Chest trauma | 60% | |
Multiple organ dysfunction syndrome | 20%-80% | |
Sepsis | 50%-100% | |
Head injury, with patient sedated | 89% | |
Head injury, with patient not sedated | 138% | |
Critical illness in emergency department | 60% | |
Nursing Activities That Increase ![]() |
||
Dressing change | 10% | |
Electrocardiogram | 16% | |
Agitation | 18% | |
Physical examination | 20% | |
Visitor | 22% | |
Bath | 23% | |
Chest x-ray examination | 25% | |
Weighing on sling scale | 36% | |
Conditions That Decrease ![]() |
||
Anesthesia | 25% | |
Anesthesia in burned patients | 50% |
Incorporating SVO2 or SCVO2 Values into the Hemodynamic Assessment.
Therapeutic Goals for SVO2 or SCVO2.
The concept of therapeutic goals or targets is helpful when developing protocols for hemodynamic assessment. Accepted therapeutic values for venous oximetry are: an SvO2 of 70% or above and an SCVO2 of 65% or above. The concept is that patients with values below these values are at greater risk for organ hypoperfusion and increased mortality. This was seen in a study of intraoperative and postoperative surgical patients, in whom an SCVO2 below 65% was associated with increased mortality.86 However, cardiac surgery patients who were below the SCVO2 65% target did not have lower mortality rates.87 Based on studies of patients with sepsis, the Surviving Sepsis Campaign guidelines recommend that SCVO2 be maintained above 70% for septic patients.83,84 This represents a clinically useful target to aim for even as research is ongoing to find the SCVO2 value for optimal survival.
Low SVO2 or SCVO2.
If SvO2 or SCVO2 falls below 40%, and is maintained at this low value, the imbalance of oxygen supply and demand will not be adequate to meet tissue needs at the cellular level. At some point, the cells change from an aerobic to anaerobic mode of metabolism, which results in the production of lactic acid and is representative of a shock state in which cellular injury or cell death may result. At this point, every attempt must be made to determine the cause of the low SvO2 or SCVO2 and to correct the oxygen supply-demand imbalance. To avoid the risk of lactic acidosis, it is helpful to watch the trend of the SvO2 or SCVO2 and to intervene early with a goal of returning the venous oxygen saturation to 70%.84
High SVO2 or SCVO2.
In certain clinical conditions, SvO2 or SCVO2 may increase to an above-normal level (>80%). This occurs during times of low oxygen demand, such as during anesthesia or hypothermia. In some cases of septic shock, the tissue cells cannot use the oxygen supplied to them, and the oxygen is not extracted from the blood at the tissue level. In this situation, the venous oxygen reserve remains elevated, and the SvO2 or SCVO2 value is higher than normal (Table 11-7). If the SvO2 PA catheter drifts into a wedged position, the SvO2 increases because the fiberoptic tip of the catheter comes into contact with newly oxygenated blood.
TABLE 11-7
MEASUREMENTS OF MIXED VENOUS OXYGEN SATURATION
![]() |
PHYSIOLOGICAL BASIS FOR CHANGES IN ![]() ![]() |
CLINICAL DIAGNOSIS AND RATIONALE |
High ![]() (80%-95%) |
Increased oxygen supply Decreased oxygen demand |
Patient receiving more oxygen than required by clinical condition Anesthesia, which causes sedation and decreased muscle movement Hypothermia, which lowers metabolic demand (e.g., during cardiopulmonary bypass) Sepsis caused by decreased ability of tissues to use oxygen at a cellular level False high-positive result because the pulmonary artery catheter is wedged in a pulmonary arteriole ![]() |
Normal ![]() ![]() Low ![]() ![]() |
Normal oxygen supply and metabolic demand Decreased oxygen supply caused by: Low hemoglobin (Hgb) Low arterial saturation (SaO2) Low cardiac output (CO) Increased oxygen consumption ( ![]() |
Balanced oxygen supply and demand Anemia or bleeding with compromised cardiopulmonary system Hypoxemia resulting from decreased oxygen supply or lung disease Cardiogenic shock caused by left ventricular pump failure Metabolic demand exceeds oxygen supply in conditions that increase muscle movement and increase metabolic rate, including physiological states such as shivering, seizures, and hyperthermia and nursing interventions such as being weighed on a bed scale and turning |
Modified from White KM, et al: The physiologic basis for continuous mixed venous oxygen saturation monitoring, Heart Lung 19(5 Pt 2):548, 1990.
Electrocardiography
Nursing Management
Nursing priorities for the patient with bedside ECG monitoring focus on accurate: (1) selection of the ECG lead, (2) interpretation of the ECG, and (3) initiation of emergency measures to treat dysrhythmias when required.
Selecting ECG Leads
ECG Leads.
The basic 3-lead ECG system consists of three bipolar electrodes that are applied to the chest wall and labeled right arm (RA), left arm (LA), and left leg (LL) (Figure 11-19). Three leads are created from this configuration, called simply Lead I, Lead II, and Lead III, (Figure 11-20). A 5-lead ECG system is typically used in critical care. This system incorporates Leads I-III, plus three augmented vector leads: aVR, aVL, and aVF, (see Figure 11-20) and at least one chest lead (precordial lead), also known as a “V” lead. Leads are color-coded to decrease the risk of misplacement. A multi-select function on the bedside monitor allows clinicians to switch between views and quickly obtain multiple images of the patient’s ECG rhythm.88
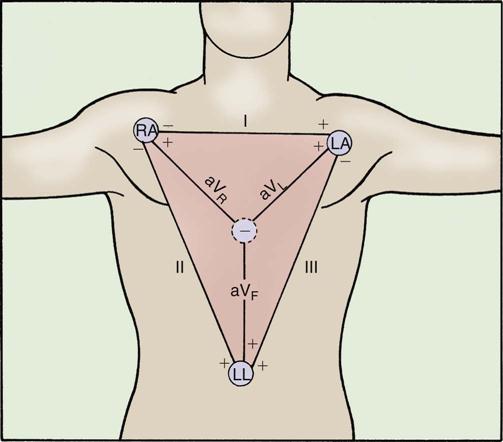
Leads are located on the extremities: right arm (RA), left arm (LA), and left leg (LL). The right leg electrode serves as a ground. Leads I, II, and III are bipolar, with each using a positive electrode and a negative electrode. Leads aVR, aVL, and aVF are augmented unipolar leads that use the calculated center of the heart as their negative electrode.
A bipolar lead system means that each displayed ECG lead has a positive and a negative pole. One lead also acts as a ground. The function of the ground electrode is to prevent the appearance of background electrical interference on the ECG tracing. Leads do not transmit any electricity to the patient; they sense and record it.
ECG Paper.
ECG paper records the speed and magnitude of electrical impulses on a grid composed of small and large boxes (Figure 11-21). Every large box has five small boxes in it. At a standard paper speed of 25 mm/sec, on the horizontal axis, one small box (1 mm) is equivalent to 0.04 second, and one large box (5 mm) represents 0.20 second. Distances along the horizontal axis represent speed and are stated in seconds rather than in millimeters or number of boxes. The vertical axis represents the magnitude, or force, of the electrical signal. One small vertical box equals 0.1 mm. The vertical scale is also standardized to a specific calibration, usually a rise of 10 mm (2 large boxes) in response to a 1 mV electrical signal, evident at the beginning of the ECG tracing.
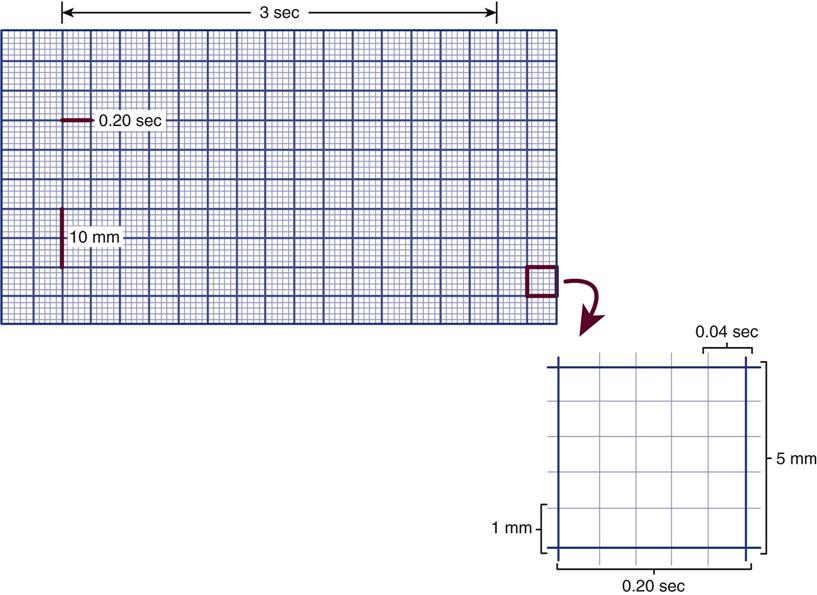
The horizontal axis represents time, and the vertical axis represents the magnitude of voltage. Horizontally, each small box is 0.04 second, and each large box is 0.20 second. Vertically, each large box is 5 mm. Markings are present every 3 seconds at the top of the paper for ease in calculating heart rate.
Interpreting the ECG
The analysis of waveforms and intervals provide the basis for ECG interpretation (Figure 11-22).
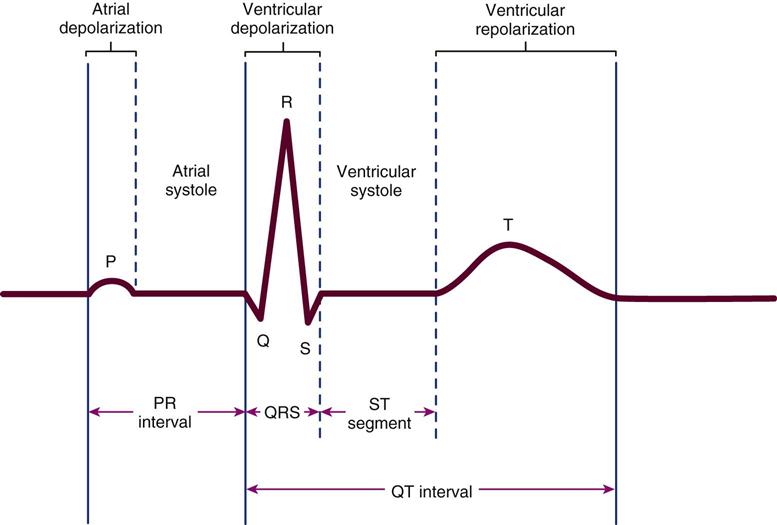
The P wave represents atrial depolarization, followed immediately by atrial systole. The QRS represents ventricular depolarization, followed immediately by ventricular systole. The ST segment corresponds to phase 2 of the action potential, during which time the heart muscle is completely depolarized and contraction normally occurs. The T wave represents ventricular repolarization. The PR interval, measured from the beginning of the P wave to the beginning of the QRS, corresponds to atrial depolarization and impulse delay in the atrioventricular (AV) node. The QT interval, measured from the beginning of the QRS complex to the end of the T wave, represents the time from initial depolarization of the ventricles to the end of ventricular repolarization.
P Wave.
The P wave represents atrial depolarization.
QRS Complex.
The QRS complex represents ventricular depolarization, corresponding to phase 0 of the ventricular action potential. It is referred to as a complex because it consists of several different waves. The letter Q is used to describe an initial negative deflection; only if the first deflection from the baseline is negative will it be labeled a Q wave. The letter R applies to any positive deflection. If there are two positive deflections in one QRS complex, the second is labeled R′ (“R prime”) and is commonly seen in lead V1 in patients with right bundle branch block. The letter S refers to any subsequent negative deflections. Any combination of these deflections can occur and is collectively called the QRS complex (Figure 11-23). The QRS duration is normally less than 0.10 second (2.5 small horizontal boxes).
T Wave.
The T wave represents ventricular repolarization. The onset of the QRS to approximately the midpoint or peak of the T wave represents an absolute refractory period, during which the heart muscle cannot respond to another stimulus no matter how strong that stimulus may be (Figure 11-24). From the midpoint to the end of the T wave, the heart muscle is in the relative refractory period. The heart muscle has not yet fully recovered, but it can be depolarized again if a strong enough stimulus is received. This can be a particularly dangerous time for ventricular ectopy to occur, especially if any portion of the myocardium is ischemic, because the ischemic muscle takes even longer to fully repolarize. This sets the stage for the disorganized, self-perpetuating depolarization of various sections of the myocardium known as ventricular fibrillation (VF).
Intervals between Waveforms.
The intervals between waveforms are evaluated (see Figure 11-22).
PR Interval.
The PR interval is measured from the beginning of the P wave to the beginning of the QRS complex. Normally, the PR interval is 0.12 to 0.20 second long and represents the time between sinus node discharge and the beginning of ventricular depolarization (see Figure 11-22). Because most of this period results from delay of the impulse in the AV node, the PR interval is an indicator of AV nodal function.
In the electrophysiology laboratory and in some critical care units, these time values are described in milliseconds. There are 1000 milliseconds (msec) in 1 second. The normal PR interval value can also be written as 120 to 200 msec.
ST Segment.
The ST segment is the portion of the wave that extends from the end of the QRS to the beginning of the T wave. Its duration is not measured. Instead, its shape and location are evaluated.89,90 The ST segment is normally flat and at the same level as the isoelectric baseline. The baseline measurement is taken 60 to 80 msec after the J point (Figure 11-25). Any vertical change in the ST segment from baseline is expressed in millimeters and may indicate myocardial ischemia (one small box equals 1 mm). ST-segment elevation (vertical increase above baseline greater than 1 mm) is associated with acute myocardial injury91 and pericarditis. ST-segment depression (decrease from baseline more than 1 mm) is associated with myocardial ischemia.92 The ST segment must be monitored carefully in high-risk patients.92
QT Interval.
The QT interval is measured from the beginning of the QRS complex to the end of the T wave and indicates the total time interval from the onset of depolarization to the completion of repolarization (see Figure 11-22). There is no established bedside monitoring lead recommended for measuring the QT interval.89 On the 12-lead ECG, the QT interval is usually the longest in precordial (chest) leads V3 and V4.89 The important point is that each clinician measures the QT interval using the same ECG lead.89 At normal heart rates, the QT interval is less than one half of the R-R interval when measured from one QRS complex to the next. However, the length of a QT interval depends on heart rate and must be adjusted according to the heart rate to be evaluated accurately.
Because the QT interval shortens with faster heart rates and lengthens with slower heart rates, it is often written as a “corrected” value (QTc), meaning the QT value was mathematically corrected as if the heart rate were 60 beats/min.93 This allows comparison of QTc across a range of heart rates. The normal QTc is less than 0.46 second (460 msec) in women and less than 0.45 second (450 msec) in men.89 A prolonged QT interval is significant because it can predispose the patient to the development of polymorphic VT, also known as torsades de pointes. A long QT interval can be the result of a congenital chromosomal abnormality, or it can be acquired as a result of electrolyte imbalance or antidysrhythmic drug therapy.94,95
Heart Rate Determination.
The first thing to assess when evaluating a rhythm strip is the ventricular rate. Regardless of the dysrhythmia involved, the ventricular rate holds the key to whether the patient can tolerate the dysrhythmia (i.e., maintain adequate blood pressure, CO, and mentation). If the ventricular rate is consistently greater than 200 or less than 30, emergency measures must be started to correct the rate. A detailed analysis of the underlying rhythm disturbance can proceed later, when the immediate crisis is over. The three methods for calculating rate when the rhythm is regular (Figure 11-26, A) follow:
2. Number of large boxes between QRS complexes divided into 300
3. Number of small boxes between QRS complexes divided into 1500
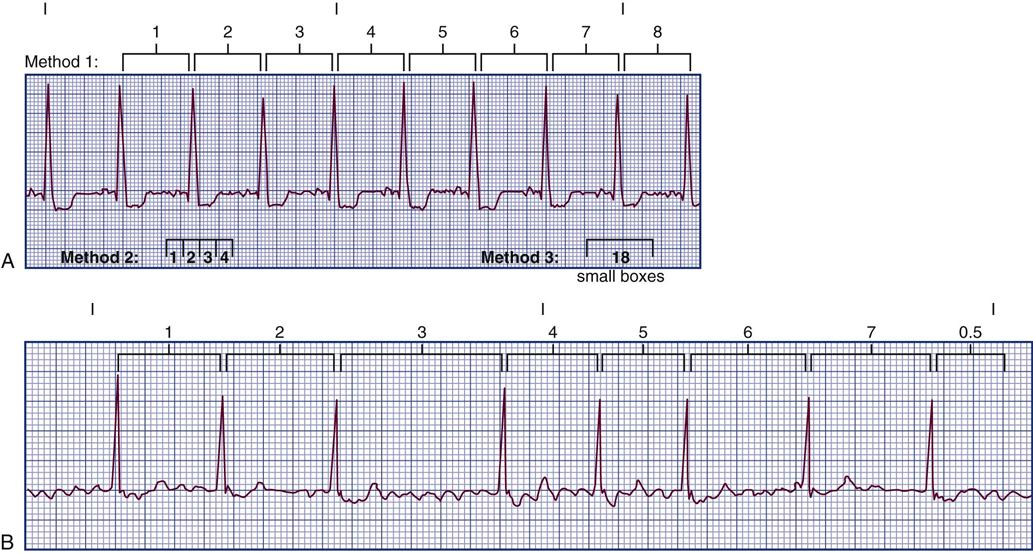
Method 1: number of R-R intervals in 6 seconds multiplied by 10 (e.g., 8 × 10 = 80/min). Method 2: number of large boxes between QRS complexes divided into 300 (e.g., 300 ÷ 4 = 75/min). Method 3: number of small boxes between QRS complexes divided into 1500 (e.g., 1500 ÷ 18 = 84/min).
B, Rate calculation if the rhythm is irregular, as in this example of atrial fibrillation.
Only method 1 can be used (e.g., 7.5 intervals × 10 = 75/min).
In the healthy heart, the atrial rate and the ventricular rate are the same. However, in many dysrhythmias, the atrial and ventricular rates are different, and both must be calculated. To find the atrial rate, the PP interval, instead of the R-R interval, is used in one of the three methods listed for determining rate.
When the rhythm is irregular, the first method (R-R intervals in 6 seconds × 10) is the only method that can be used (see Figure 11-26, B).
Rhythm Determination.
The term rhythm refers to the regularity with which the P waves or R waves occur. Calipers assist in determining rhythm. One point of the calipers is placed on the beginning of one R wave, and the other point is placed on the next R wave. Leaving the calipers “set” at this interval, each succeeding R-R interval is checked to be sure it is the same width as the first one measured.
P-Wave Evaluation.
The P wave is analyzed by answering the following questions. First, is the P wave present or absent? Second, is it related to the QRS? It is hoped that one P wave will be in front of every QRS. Sometimes, two, three, or four P waves may be in front of every QRS. If this pattern is consistent, the P wave and QRS are still related, although not on a 1 : 1 basis.
PR-Interval Evaluation.
The duration of the PR interval, which normally is 0.12 to 0.20 second (120 to 200 msec), is measured first. This is measured from the start of a visible P wave to the beginning of the next QRS (see Figure 11-22). All PR intervals on the strip are verified to be sure they have the same duration as the original interval.
QRS Complex Evaluation.
The entire ECG strip must be evaluated to ascertain that the QRS complexes are consistently the same shape and width. The normal QRS duration is 0.06 to 0.10 second (60 to 100 msec). If more than one QRS shape is on the strip, each QRS must be measured. The QRS is measured from where it leaves the baseline to where it returns to the baseline (see Figure 11-22).
QT Evaluation.
The length of the QT varies with the heart rate. The QT interval is shorter when the heart rate is faster. A QT interval corrected for heart rate (QTc) that is longer than 0.50 second (500 msec) is of concern, as discussed earlier under “QT Interval.”
Dysrhythmia Interpretation
In clinical practice the terms “dysrhythmia” and “arrhythmia” often are used interchangeably. Both are correct, and either may be used in clinical practice; this text favors “dysrhythmia.” A dysrhythmia is any disturbance in the normal cardiac conduction pathway. Patients in critical care units always have continuous ECG monitoring. ECG rhythm strips are recorded routinely, and any time a significant rhythm change occurs.
Sinus Rhythms.
There are four rhythms that begin with the word sinus, indicating that the rhythm originates from within the sinus node. Table 11-8 provides a summary of the major features of this category of rhythms.
TABLE 11-8
PARAMETERS | NORMAL SINUS RHYTHM | SINUS BRADYCARDIA | SINUS TACHYCARDIA | SINUS DYSRHYTHMIA |
Rate | 60-100/min | <60/min | >100/min | Variable |
Rhythm | Regular | Regular | Regular | Irregular; respiratory variation |
P wave | Present, with one per QRS | Present, with one per QRS | Present, with one per QRS | Present, with one per QRS |
PR interval | 0.12-0.20 sec and constant | 0.12-0.20 sec and constant | 0.12-0.20 sec and constant | 0.12-0.20 sec and constant |
QRS | 0.06-0.10 sec | 0.06-0.10 sec | 0.06-0.10 sec | 0.06-0.10 sec |
Normal Sinus Rhythm.
(Figure 11-27.)
Sinus Bradycardia.
Sinus bradycardia meets all of the criteria for normal sinus rhythm except that the rate is fewer than 60 beats/min (see Table 11-8). Sinus bradycardia usually is not treated unless the patient displays symptoms of hypoperfusion, such as hypotension, dizziness, chest pain, or changes in level of consciousness.
Sinus Tachycardia.
Sinus tachycardia meets all the criteria for normal sinus rhythm except that the rate is greater than 100 beats/min (see Table 11-8). Rates may be as high as 180 to 200 beats/min in healthy, young adults during strenuous exercise. However, in the critical care setting, bed rest is prescribed for most patients. It is wise to be skeptical of any “sinus tachycardia” with a rate greater than 150 and to search for a triggering focus other than the sinus node. Many drugs used in critical care can cause sinus tachycardia; common culprits are dopamine, hydralazine, atropine, and epinephrine. Tachycardia is detrimental to anyone with ischemic heart disease because it decreases the time for ventricular filling, decreases stroke volume, and compromises CO. Tachycardia increases heart work and myocardial oxygen demand while decreasing oxygen supply by decreasing coronary artery filling time. If the cause of the tachycardia can be determined, such as fever or pain, that symptom is treated rather than trying to treat the heart rate directly.
Sinus Dysrhythmia.
Sinus dysrhythmia, commonly called sinus arrhythmia in clinical practice, meets all of the criteria for normal sinus rhythm except that the rhythm is irregular (see Table 11-8). This irregularity coincides with the respiratory pattern; heart rate increases with inhalation and decreases with exhalation96 (Figure 11-28). Sinus dysrhythmia often occurs in children and young adults, and the incidence decreases with age. No treatment is required. To accurately detect sinus dysrhythmia, look at all P waves closely to verify that they are all the same shape and that the PR intervals are all constant.
Atrial Dysrhythmias.
Atrial dysrhythmias originate from an ectopic focus in the atria, somewhere other than the sinus node (Table 11-9). The ectopic impulse occurs prematurely, before the normal sinus impulse occurs. The premature atrial depolarization may initiate a normal QRS complex, an abnormal or aberrant complex, or an SVT. Huge advances have been made in the understanding of the pathogenesis and management of atrial dysrhythmias.
TABLE 11-9
PARAMETER | PAROXYSMAL SUPRAVENTRICULAR TACHYCARDIA | MULTIFOCAL ATRIAL TACHYCARDIA | ATRIAL FLUTTER | ATRIAL FIBRILLATION |
Rate | ||||
Atrial | 150-250/min | 100-160/min | 250-350/min | >350/min (unable to count it) |
Ventricular | Same or less | Same | 250-350/min, one half or less | 100-180/min (uncontrolled); <100/min (controlled) |
Rhythm | Regular | Irregular | Atrial; regular; ventricular; may or may not be regular | Irregularly irregular |
P Wave | Present; abnormally shaped | Present; three or more different shapes | F waves | Fibrillatory baseline |
PR Interval | May be normal or prolonged | Variable | Conduction ratio: flutter waves per QRS | Absent |
QRS | 0.06-0.10 sec | 0.06-0.10 sec | 0.06-0.10 sec | 0.06-0.10 sec |
Premature Atrial Contractions.
Premature atrial contractions (PACs) are isolated, early beats from an ectopic focus in the atria. The underlying rhythm is usually sinus. The regular sinus rhythm is interrupted by an early, abnormally shaped atrial P wave. The early atrial wave usually looks different from the sinus P wave and may be inverted. The PR interval may be longer, shorter, or the same as the PR interval of a sinus impulse. The QRS that follows the ectopic atrial P wave can vary in shape depending on the degree of refractoriness of the AV node.