CHAPTER 14 Blood Conservation
Jean-Baptiste Denis is thought to be the first to attempt an intravenous transfusion of whole blood. He was a Parisian physician and astrologer who, in 1667, gave a teenage boy the blood of either a lamb or a dog in an attempt to restore him after he had been bled multiple times for fever. Denis was later tried for murder when another of his patients died. He was exonerated, but transfusion was subsequently banned throughout Europe for more than 100 years (Moore, 2003).
More than 300 years after the death of Pope Innocent VIII, the first successful human transfusion was performed in Philadelphia and credited to the University of Edinburgh-trained “Father of American Surgery,” Philip Syng Physick (Fig. 14-1). He did not publish any writings about his accomplishment and few details exist of the circumstances or outcome (Jepson, 1974).
Hemoglobin structure and function in the neonate, infant, and child
Hemoglobin, the primary oxygen-carrying pigment, is a large complex tetrameric protein consisting of iron-containing heme groups (protoporphyrin IX ring with attached ferrous iron atom) and the globin protein moiety (Fig. 14-2). The paired arrangement of polypeptide globin chains each interacting with an attached heme group provides the complex reversible interactions that allow for the transport of oxygen. Because each heme moiety has the capacity to bind a single oxygen molecule, a molecule of hemoglobin can transport as many as four oxygen molecules; remarkably, this process is accomplished without the input of energy.
Hemoglobin structure during the embryologic period is characterized by three hemoglobin species, which include Gower-1(ζ2ε2), Gower-2 (α2ε2), and Portland (ζ2γ2) (Fig. 14-3). By the tenth week of gestation, these embryonic hemoglobin species are nearly completely replaced by fetal hemoglobin (α2γ2), which is also called hemoglobin F. At 10 to 12 weeks of gestation, the distribution of hemoglobin is about 80% to 90% fetal hemoglobin and 10% hemoglobin A. Synthesis of fetal hemoglobin ceases at approximately 38 weeks of gestation; at birth, the percentage of fetal hemoglobin has decreased to about 70% to 80% and, under normal circumstances, continues to decrease thereafter. By 6 months of age, fetal hemoglobin levels typically have decreased to less than 5% and by 1 year of age, to 2%, which is a level similar to that in adults.
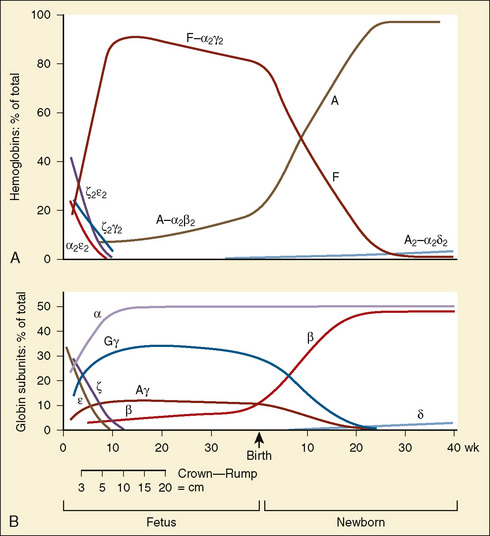
(Modified from Polin RA, Fox WW: Fetal and neonatal physiology, ed 2, Philadelphia, 1998, Saunders.)
The primary physiologic function of hemoglobin is to carry oxygen acquired in the capillary beds of the pulmonary alveoli (or, in fetal life, the chorionic villi of the maternal placenta) and release it in the reduced-oxygen environment of the tissues. Hemoglobin also is important as a biological buffer and in the transport of both carbon dioxide (the Bohr effect) and nitric oxide (Fig. 14-4). In its primary role as an oxygen carrier, hemoglobin functions by altering its affinity for oxygen through changes in the quaternary structure of the protein moiety. This relationship can be usefully illustrated by examining the appearance of the oxyhemoglobin dissociation curve (Fig. 14-5). The sigmoidal shape of the curve, which describes the relationship of hemoglobin saturation at various levels of oxygen tension, results from the four globin chains individually interacting with oxygen and collectively affecting the affinity of the other chains for oxygen. This complex interaction results in an affinity curve that demonstrates hemoglobin’s low affinity (flat portion of the lower curve) for oxygen in hypoxic environments and a rapidly increasing affinity (steep portion) as oxygenation of each heme group occurs until the molecule becomes saturated (flat portion of upper curve). The classic shape of the curve is described for hemoglobin A and reflects the physiologic requirement to load and unload oxygen within a narrow range of oxygen tensions. Other hemoglobin species and mixtures of species have different affinities for oxygen and therefore produce different dissociation curves that in turn reflect embryonic or fetal needs.
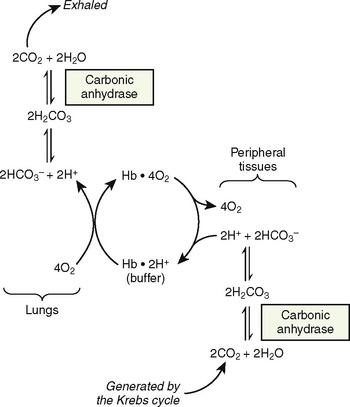
(Modified from Polin RA, Fox WW: Fetal and neonatal physiology, ed 2, Philadelphia, 1998, Saunders.)
The dissociation curve for fetal hemoglobin reflects its function as an acceptor of oxygen carried by maternal hemoglobin A. Therefore, fetal hemoglobin must have a greater affinity for oxygen than maternal hemoglobin (hemoglobin A) to accept oxygen carried to the uterine villi. The increased affinity of fetal hemoglobin for oxygen can be traced to its lower capacity to interact with 2,3-diphosphoglycerate (2,3-DPG), because the binding site for 2,3-DPG is on the β chain, which is absent in fetal hemoglobin (Jepson, 1974). Although one would expect that the increased affinity for oxygen characteristic of fetal hemoglobin would be essential for adequate oxygen delivery in the fetus, that appears not to be the case, as illustrated by the lack of deleterious effects on the fetus when hemoglobin A is transfused in utero (Mathers et al., 1970). Furthermore, infants born to mothers with hemoglobinopathies characterized by an increased affinity for oxygen show no apparent effects (Moore et al., 1967). By extension, it can be presumed that in the neonate, transfusion with blood containing hemoglobin A is not harmful and may in fact have clear advantages, especially in critical illness (Oski, 1973).
As previously discussed, the characteristic sigmoidal shape of the oxyhemoglobin dissociation curve of hemoglobin A is a reflection of its structure, whereas its position with respect to oxygen saturation and oxygen tension is a function of various factors. Under normal circumstances, the oxygen tension at which hemoglobin A is 50% saturated (P50) is 27 mm Hg. Temperature, pH, Pco2, and 2,3-DPG levels all have profound effects on oxygen affinity and therefore on P50, resulting in a leftward or rightward shift in the position of the curve. Because the appearance of the oxyhemoglobin dissociation curve for the neonate is identical to that of the adult at a pH of 7.6, environmental differences are potentially more important to hemoglobin’s functional affinity than are fundamental differences in the hemoglobin molecule itself (Nelson et al., 1964). Under normal circumstances, the P50 increases from about 19 mm Hg at 1 day of age to the adult level of 27 mm Hg at age 4 to 6 months (Fig. 14-6). At the end of the first year, the P50 actually exceeds that of the adult at a level slightly greater than 30 mm Hg (Oski, 1973). For further reading, see Chapter 3, Respiratory Physiology in Infants and Children.
Physiologic anemia and the anemia of prematurity
At term, the neonate has a hemoglobin concentration of approximately 17 g/dL. As hemoglobin F is replaced with hemoglobin A over the ensuing months, the hemoglobin level decreases to a low of 10 g/dL, and the oxyhemoglobin dissociation curve shifts rightward. This shift is the result of the combination of increasing levels of hemoglobin A and increased levels of 2,3-DPG as described by Oski (1973). An increase in the levels of either one alone is insufficient. The importance of this is apparent in infants who have respiratory distress syndrome with abnormally low levels of 2,3-DPG and who show improved oxygen unloading at the tissue level after transfusion with fresh adult blood. The transition from a P50 of 19 mm Hg in the term neonate to 27 mm Hg (as in the adult) typically occurs over 4 to 6 months. However, in the premature patient this transition may be delayed to as late as age 12 months.
At birth, oxygen tensions increase quickly, effectively halting erythropoietin synthesis, and consequently, erythropoiesis. In the full-term neonate, erythropoietin and, consequently, hemoglobin levels begin increasing around the age of 4 months, resulting in the correction of physiologic anemia, which is often called the physiologic nadir to emphasize the physiologic or nonpathologic nature of the decrease in hemoglobin. In premature infants, the phenomenon is more complex and is complicated by the need for repeated blood sampling in hospitalized premature infants. Often, the blood sampling requirements equal or exceed half of the total blood volume in infants weighing less than 1 kg (Stockman, 1986). The need for phlebotomy results in a need for the transfusion of adult banked blood—blood containing hemoglobin A with its characteristic lower affinity for oxygen. The resulting increase in tissue-oxygen tension further decreases erythropoietin synthesis and prolongs the duration of anemia for a period that is dependent on factors such as weight, gestational age, and the ongoing need for transfusion.
The anemia of prematurity is a true anemia that produces clinical signs and symptoms such as tachycardia, bradycardia, apnea, delayed growth, and poor weight gain. Treatment is directed at these consequences and consists of either transfusion, or more recently, the use of recombinant erythropoietin. Studies examining the potential benefit of booster transfusions targeted to keep hemoglobin levels greater than 10 g/dL in premature infants have been mixed; some have shown improvements in weight gain, and others have failed to demonstrate benefit (Blank et al., 1984; Stockman et al., 1984; Keyes et al., 1989).
The use of recombinant erythropoietin has also been studied extensively in the treatment of anemia of prematurity. A Cochrane Review described the results of 23 studies involving 2074 infants (Fig. 14-7) (Ohlsson and Aher, 2006). The end point of the majority of the studies was a reduction in the need for transfusion and donor exposure after enrollment. In the majority of studies, the need for transfusion was reduced; however, the reduction is of limited clinical significance. Several studies also found an increased incidence of retinopathy of prematurity among those treated with erythropoietin. The lack of clinically relevant benefit and the potential to increase the occurrence of retinopathy of prematurity prompted the recommendation against the use of erythropoietin as a means of reducing the need for transfusion in premature infants. Transfusion practices and indications in this group are discussed later in this chapter.
Perioperative strategies for blood conservation
Erythropoietin
As discussed in the previous section, erythropoietin is an inducible glycoprotein produced in the kidneys and extrarenal tissues. It regulates erythropoiesis in response to tissue hypoxia. Acute anemia is associated with exponential increases in erythropoietin in the plasma. However, in the critically ill, erythropoietin induction is blunted, as has also been observed in various chronic illnesses in childhood. The exact mechanism of the inhibition observed in both critical illness and chronic illness has not been described (Krafte-Jacobs et al., 1994). Regardless of the mechanism, both situations often lead to the need for blood transfusion. The use of recombinant erythropoietin has been investigated as a means of reducing the need for transfusion or the frequency of transfusion in chronic or critical illness.
Adult studies have shown mixed results in the efficacy of recombinant human erythropoietin to avoid or limit blood transfusion. Several randomized controlled studies, including a study reported by Silver et al. (2006), have shown that at least 1 unit of blood was saved in patients in an adult intensive care unit (Corwin et al., 2002). More recently, however, Corwin and colleagues (2007) reported that in a large, prospective, randomized controlled trial involving more than 1400 adult patients, the group receiving erythropoietin had a 10% decrease in the need for transfusion compared with the control group. However, that study and others found an increase in the instances of thrombotic events among patients receiving erythropoietin.
A benefit, albeit small, was found in studies that examined the use of perioperative erythropoietin for reducing the need for transfusion during and after procedures in which transfusion is normally required. Laupacis and Fergusson (1998), in a meta-analysis involving adults undergoing either orthopedic or cardiac surgery, found a significant reduction in allogenic RBC transfusion in both groups of patients (Fig. 14-8). In a study of children undergoing craniofacial repair, Helfaer et al. (1998) found that children receiving preoperative erythropoietin required transfusion significantly less often than controls (64% vs. 100%). Other small studies (183) and case reports have concluded that erythropoietin is efficacious in reducing the need for RBC transfusion in children, but in the absence of data from large, well-designed prospective trials, its use cannot be recommended.
Iron Supplementation
The incidence of iron deficiency anemia is approximately 9% among children 1 to 2 years old in the United States (Looker et al., 1997). For adult patients with normal iron storage, there is conflicting evidence as to whether iron supplementation perioperatively improves the hemoglobin level. Several randomized control trials have failed to show that oral iron supplementation increases hemoglobin levels perioperatively (Crosby et al., 1994; Sutton et al., 2004; Mundy et al., 2005). However, two clinical trials (one randomized and one nonrandomized) with colorectal surgical patients have shown that treatment with oral iron supplementation for two weeks significantly increased hemoglobin levels and decreased blood transfusion rates (Okuyama et al., 2005; Lidder et al., 2007). The conclusion of a review by Beris et al. (2008) for the Network for Advancement of Transfusion Alternatives was that there is insufficient evidence to recommend the use of intravenous iron as a means of reducing the need for perioperative transfusion in adults. As with the use of erythropoietin, few data for children are available.
Hemostatic Drugs
Three currently available hemostatic drugs have been well investigated and extensively used to limit blood loss perioperatively. Two of the drugs, aminocaproic acid (EACA) and tranexamic acid (TA), are lysine amino-acid synthetic derivatives; the third, aprotinin, is a naturally occurring antifibrinolytic and proteinase inhibitor (Fig. 14-9). These drugs have been extensively used in adults and, more recently, in children.
Fibrinolysis, the lysis of formed fibrin clot, results from the enzymatic conversion of the proenzyme plasminogen to plasmin, a process that is mediated by tissue plasminogen activator, urokinase, factors XIa and XIIa, and kallikrein. Fibrinolysis results in the cleavage of polymerized fibrin strands at multiple sites and releases fibrin degradation products such as D dimer (Kolev and Machovich, 2003). EACA and TA exert their antifibrinolytic activity by reversibly blocking the lysine binding site on plasminogen, preventing binding to fibrin and conversion to active plasmin. As an inhibitor of fibrinolysis, TA is 10 times more potent than EACA. TA may also improve hemostasis by preventing plasmin-induced platelet activation, and both EACA and TA have antiinflammatory properties, but they are less than those of aprotinin (Eaton, 2008).
Antifibrinolytics have been used in children primarily for spine surgery and cardiac surgery, although they have been used for other procedures, including craniofacial reconstruction and repair of congenital diaphragmatic hernia during extracorporeal membrane oxygenation (ECMO). Sethna et al. (2005) reported on a randomized study of 44 pediatric patients undergoing elective spine surgery who received either TA (100 mg/kg loading dose followed by 10 mg/kg per hour) or saline placebo during the procedure; the treatment group had a 41% reduction in blood loss (Fig. 14-10). In a subsequent study involving children with Duchenne’s muscular dystrophy, the same authors found a similar reduction in the transfusion requirement (Shapiro et al., 2007).
For adult cardiac surgery, the use of antifibrinolytics is well established. For children, although the efficacy data are less available and of lower quality, they support the use of TA and EACA primarily in children undergoing repair of cyanotic congenital heart disease. Bulutcu et al. (2005), in a series of studies involving 750 cyanotic patients, found that both TA and EACA were beneficial in reducing transfusion requirements by up to 50%, reducing blood loss by 44%, and significantly reducing times for sternal closure and rate of reexploration.
Few complications have been associated with the use of TA and EACA, although concerns have been related to thrombosis in patients, such as those undergoing ECMO or a Fontan procedure requiring the use of a baffle fenestration. Although case reports suggest the potential for concern, studies involving 71 patients undergoing a Fontan procedure and 431 patients undergoing ECMO have failed to demonstrate an increased risk of thrombosis (Hocker and Saving, 1995; Gruber et al., 2000; Downard et al., 2003).
Aprotinin is a nonspecific serine protease inhibitor derived from bovine lung that inhibits proteases with active serine residues, especially plasmin. The resulting effects are an attenuation of inflammatory responses and antifibrinolysis. Aprotinin and the lysine analogues have very different modes and scopes of action, but ultimately both function by inhibiting fibrinolysis through the inhibition of plasmin. Additionally, aprotinin is thought to restore the adhesive properties of platelets independently of its effect on the inhibition of fibrinolysis (Bradfield and Bode, 2003). The efficacy of aprotinin is somewhat less clear than that of either EACA or TA. Eaton (2008), in a comprehensive review published in 2008, described in detail the available data on the efficacy of aprotinin use in pediatric cardiac surgery, in which it is most often used. Of the 14 randomized controlled trials, 11 showed a reduction in at least one parameter of blood loss or replacement. Three studies by Boldt et al. (1994, 1993a, 1993b) showed no benefit, whereas the majority of observational studies described in Eaton’s review did show benefit. The difficulty in evaluating the extensive literature on the use of aprotinin in pediatric cardiac surgery lies in the differences in end points (e.g., transfusion requirement, or chest tube output), dosing regimens (i.e., high dose vs. low dose), surgical procedures (e.g., Fontan procedure or ventricular septal defect), cardiopulmonary bypass management, and patients (e.g., infants, neonates, or reoperation). The relatively small numbers evaluated within each study and the virtually endless potential permutations of the resulting data make drawing conclusions about efficacy difficult, although the weight of the existing evidence in adults and children suggests a benefit similar to that obtained with the lysine derivatives TA and EACA.
The use of aprotinin has raised concerns about the potential for complications, including thrombosis, anaphylaxis, and, most importantly, renal failure. In 2006 the Multicenter Study of Perioperative Ischemia Research Group reported on the largest observational prospective study of antifibrinolytic therapy (Mangano et al., 2006). The study tracked 4374 patients undergoing coronary artery bypass grafting and compared the use of aprotinin (1295 patients), EACA (883 patients), and TA (822 patients) with placebo (1374 patients). Aprotinin was associated with higher risks of death, cardiovascular event, cerebrovascular event, and renal failure. EACA and TA were not associated with increases in renal, cardiac, or neurologic complications. All three agents decreased blood loss to essentially the same degree.
In 1993, the Food and Drug Administration (FDA) approved aprotinin for patients at high risk of bleeding who were undergoing coronary artery bypass grafting with cardiopulmonary bypass (Ray and Stein, 2008). After the publication of the Blood Conservation Using Antifibrinolytics in a Randomized Trial (BART) study (Fergusson et al., 2008), Bayer Pharmaceuticals notified the FDA of its intent to withdraw aprotinin from the market. In that study of 2331 high-risk adult cardiac surgery patients, the investigators sought to determine whether aprotinin was superior to either TA or EACA in decreasing significant postoperative bleeding. The trial was terminated early because of an excess of deaths in the aprotinin group (6%) compared with the TA group (3.9%) and the EACA group (4.0%) (Fig. 14-11).
Preoperative Autologous Blood Donation
Since the 1980s, preoperative autologous donation (PAD) of blood 2 to 3 weeks before the operation has been used for adult cardiac and noncardiac surgical procedures in which blood loss and the need for blood transfusion are expected. The primary goal is to decrease the amount of allogenic blood transfused (Nath and Pogrel, 2005; Schved, 2005; Ferraris et al., 2007).
Numerous studies have documented the safety and benefit of this practice for adults in various settings. The main benefit is that it decreases the exposure to allogenic blood. A concern, though, is the amount of blood transfused (both allogenic and autologous) in patients who undergo PAD (Henry et al., 2002). The increased rate of transfusion is thought to lead to an increased risk of administrative errors with the increased number of units transfused (Schved, 2005). Vega et al. (2008) reported statistically higher complication rates among patients having reconstructive breast surgery who had PAD compared with patients in a control group who did not preoperatively donate their own blood.
Masuda et al. (2000) studied children weighing less than 20 kg. The children were not given erythropoietin, and each child predonated a mean (with standard deviation indicated as SD) of 48 (SD = 17) mL/kg of blood over an average of 50 (SD = 16) days. No child in the study group received allogenic blood transfusion, but 80% of children in the control group did. Sonzogni et al. (2001) pretreated children in a PAD group with subcutaneous erythropoietin 3 times a week for 3 weeks preceding cardiac surgery and once intravenously on the day of the operation. The controls were 39 consecutive age-matched patients from the previous year. Children predonated 9 mL/kg of blood on two separate occasions if the hematocrit was greater than 33%. Three of the 39 children in the study group required transfusion with allogenic blood, compared with 24 of the 39 in the control group.
Most studies of PAD in orthopedics involve scoliosis surgery. Murray et al. (1997) studied 243 consecutive pediatric patients undergoing spinal fusion and found that 90% of the children who predonated did not require allogenic blood during surgery. Moran et al. (1995) reported similar results in their study of children undergoing spinal fusion. In that study, the proportion of patients who needed allogenic blood (11%) was nearly identical to that found by Murray and colleagues. In both studies, at least 70% of the children were able to complete the donation process. Concern about the ability of children, especially young children, to complete the donation program is often cited as an obstacle to PAD. However, in both of these studies, children younger than 10 years successfully completed the donation process. Clearly, the ability of infants, toddlers, and young school-aged children to tolerate the donation process is uncertain at best. The use of sedation or general anesthesia to facilitate this process would seem to be somewhat unreasonable and excessive, although it has been reported (Velardi et al., 1998). A contraindication for PAD includes predonation anemia.
Acute Normovolemic Hemodilution
ANH is a technique designed to decrease the need for allogenic blood transfusion by the preoperative withdrawal of the patient’s own blood to be reinfused as needed during the subsequent procedure. The withdrawn blood is collected in standard blood-collection bags containing anticoagulant. The circulating volume is restored with either colloid (1:1) or crystalloid (3:1), thereby reducing the hematocrit and the potential for loss of RBC mass as bleeding occurs during the procedure (Helm et al., 1996; Ferraris et al., 2007). Children weighing less than 35 kg typically do not fill an entire collection bag. As bags typically contain approximately 63 mL citrate-phosphate-dextrose (CPD) and hold 450 mL blood, the volume of the anticoagulant needs to be reduced if the volume of collected blood is less than 450 mL. Studies of adults have examined the potential benefits of various replacement fluids, including hetastarch, albumin, and Lactated Ringer’s solution. All appear to be acceptable in adults, but few data exist to guide practice in children. Given that phlebotomy takes place just before the procedure and the withdrawn blood never leaves the patient’s operating room, the potential for error in transfusing the wrong blood to the wrong patient is greatly reduced. The withdrawn fresh whole blood, which contains the non-RBC components not found in banked blood, is reinfused at the end of the procedure.
The volume of blood to be removed can be calculated with the following formula:
where V is volume to be removed; EBV is estimated blood volume; Hi is initial or starting hematocrit value; Hf is the final or desired hematocrit value; and Hav is the average hematocrit value. The final or desired hematocrit is unclear and is dependent on various factors, including age, procedure, coexisting disease, and expected blood loss. Some have suggested that young infants are not appropriate candidates for ANH, because they have a limited capacity to increase cardiac output in response to anemia and the presence of fetal hemoglobin may limit oxygen unloading (Weldon, 2005). Supporting data, however, are lacking. In studies of older children and adolescents, hematocrit values have been allowed to decrease to as low as 9% without the development of lactic acidosis or other evidence of hypoperfusion (Fontana et al., 1995). The lowest appropriate or safe level of the desired hematocrit value is a function of the critical hemoglobin, which is the hemoglobin or hematocrit value below which oxygen consumption becomes delivery dependent and the ability to increase cardiac output is exceeded as lactic acidosis develops, reflecting insufficient oxygen delivery to the tissues. The critical hemoglobin concentration for humans is unknown, although studies in young healthy volunteers have failed to produce evidence of insufficient oxygen delivery at hemoglobin concentrations as low as 4.8 g/dL despite pharmacologic maneuvers that limited oxygen delivery to 7.3 mL/kg per minute (Lieberman et al., 2000). With the absence of cardiovascular disease in children, the ability to tolerate hematocrits of this level can be reasonably assumed; however, no large body of data exists to support the safety of the practice in children. Safety concerns must be balanced against the evidence for benefit usually determined by a reduction in total allogenic blood transfused or the reduction in exposure to units of allogenic blood. Linderkamp et al. (1992) calculated the following minimally acceptable hemoglobin concentrations: 6 g/dL for children and adults, 12 g/dL for preterm infants at birth, and 11 g/dL for full-term neonates at birth. These critical values were determined from oxygen transport parameters and oxygen consumptions (Linderkamp et al., 1992). Of course, the size of the preterm infant and the full-term neonate would preclude them from ANH; sufficient blood could not be drawn without risking a suboptimal hemoglobin concentration.
Several prospective randomized studies in adults show a modest decrease in the need for allogenic blood transfusion in both cardiac and noncardiac surgery (Goodnough et al., 1994; Moran et al., 1995; Kumar et al., 2002). In a 1998 meta-analysis, Bryson et al. did not demonstrate clear benefit with regard to a reduction in exposure to allogenic blood. According to the Society of Thoracic Surgeons Practice Guidelines, in the adult population the usefulness of ANH is not well established but may have benefit when used in conjunction with other blood conservation strategies (Masuda et al., 2000; Ferraris et al., 2007). A small series of adult patients of the Jehovah’s Witness faith who underwent live donor hepatic transplants demonstrated a clear benefit when their results were compared with those of historical controls (Jabbour et al., 2005). The patients in this series not only underwent ANH but also were treated preoperatively with erythropoietin to increase the preoperative level of hemoglobin. The potential benefits of ANH or a combined approach to blood conservation remain unclear and can be addressed only by well-designed studies of sufficient size to provide clinicians with reassurance that the benefit outweighs any potential risk.
Deliberate Hypotension
Controlled, deliberate, or induced hypotension is a method of blood-conservation strategy first described by Cushing in 1917. Since then, the technique has been applied to numerous procedures in various settings, with a multitude of different pharmacologic agents, and with patients of all ages. In a literature review, Tobias (2002) specifically looked at agents and techniques that can be used in infants and children.
Deliberate hypotension is defined as mean arterial pressure of 50 to 65 mm Hg, or 30% below baseline, which decreases bleeding in the surgical field and may be indicated for any procedure in which a relatively bloodless operating field is needed or whenever blood loss can be expected to require transfusion (Degoute, 2007). The ideal pharmacologic agent to induce controlled hypotension has the following characteristics: short onset, easily reversible and titratable, minimal toxic metabolites, minimal effects on vital organs, and predictable dose-dependent effect (Degoute, 2007). Numerous agents have been used successfully in both adults and children. A comprehensive review of agents useful in pediatrics was prepared by Degoute (2007). A summary of drugs is shown in Table 14-1.
The efficacy of deliberate hypotension to reduce the need for transfusion and improve the quality of the surgical field has been studied extensively for more than 30 years, although no large well-designed study or meta-analysis has demonstrated its efficacy in children. Still, the technique is widely accepted and used in children and infants. Most recent studies have focused on techniques and agents that may be used to achieve hypotension rather than on safety or efficacy. Shear and Tobias (2005), however, published a small study of cerebral oxygenation during controlled hypotension to 55 to 65 mm Hg using near infrared spectroscopy. They found that even with mean pressures of less than 54 mm Hg, oximetry values never decreased to less than 20% below baseline. They concluded that deliberate hypotension within the limits of 55 to 65 mm Hg was safe with regard to cerebral oxygenation.
Techniques for achieving deliberate hypotension are primarily pharmacologic, although positioning and regional techniques are occasionally used. The pharmacologic agents are derived from several different classes, including ganglionic blockers (e.g., trimethaphan), vasodilators (e.g., nitroglycerin and nitroprusside), β-blocking agents (e.g., esmolol), calcium channel blockers (most often nicardipine), and the volatile agents (presumably sevoflurane, with its greater titratability). The review by Tobias (2002) provides an extensive discussion of various agents. Often a combination of agents is required to provide adequate control of blood pressure, especially in young, healthy adolescents. The use of vasodilators such as nitroprusside or a volatile agent often results in reflex tachycardia that limits hypotension. Most practitioners find that satisfactory hypotension can be achieved only when heart rate is controlled, typically with a β-blocker (e.g., esmolol) or with a combination agent that blocks both α and β receptors (e.g., labetalol). Other agents that may be helpful in controlling heart rate, include clonidine (an α-receptor agonist) or a narcotic (especially remifentanil), as was shown in endoscopic sinus surgery and middle ear procedures (Degoute et al., 2003; Eberhart et al., 2003).
Dexmedetomidine has gained acceptance in various settings, but its use in this setting would appear to be limited. Nonetheless, it has been compared with remifentanil in two studies (one adult and one pediatric). In neither study was dexmedetomidine found to be superior (Tobias, 2002; Richa et al., 2008). A third article described only one patient for whom dexmedetomidine was used successfully to induce hypotension for spine instrumentation (Tobias and Berkenbosch, 2002). In the absence of additional data from larger prospective studies, the use of dexmedetomidine for controlled hypotension cannot be recommended.
Although the use of deliberate hypotension is widely accepted, the practitioner must be attentive to safety concerns, primarily those related to focal ischemia to such vital areas as the retina, spinal cord, and brain. Deliberate hypotension is contraindicated in patients with compromised circulation involving any critical vascular bed, elevated intracranial pressure, profound anemia or polycythemia, or sensitivity to any of the proposed hypotensive agents. The hematocrit concentration should be maintained at an adequate level. Despite the suggestion by some that deliberate hypotension and extreme ANH may be safely combined, deliberate hypotension should never be combined with ANH because of the risk of end organ ischemia (Schaller et al., 1983). The anesthesiologist must have experience with the technique and with the agents used to achieve hypotension. Adequate monitoring is essential, including invasive arterial blood pressure in all cases and central venous pressure monitoring in many or most cases. Patients placed in the reverse Trendelenburg position or in any position in which the head is higher than the heart must have the arterial pressure monitored and zeroed to reflect cerebral perfusion pressure. Arterial blood gases should be monitored frequently to ensure that a metabolic acidosis is not present, suggesting poor tissue perfusion or cyanide toxicity, when using sodium nitroprusside. Owing to its potential for toxicity, sodium nitroprusside deserves additional mention.
Sodium nitroprusside is 44% cyanide by weight. It interacts with oxyhemoglobin and spontaneously dissociates into methemoglobin, cyanide, and nitric oxide (Fig. 14-12). Its vasodilatory action is mediated principally through the action of nitric oxide. Although it is a highly efficacious vasodilator, when used in high doses for protracted periods, sodium nitroprusside may produce intoxication with its by-product, cyanide. Free cyanide radicals generated by the metabolism or breakdown of sodium nitroprusside are metabolized by one of the following four potential mechanisms:
Treatment, when required, usually consists of immediate discontinuation of the infusion, administration of oxygen, and correction of acidosis followed by the initiation of a thiosulfate infusion (at a dose of 60 mg/kg per hour after an initial bolus of 30 mg/kg infused over 20 to 30 minutes). Alternatively, dialysis may be beneficial to remove thiocyanate; hydroxocobalamin (50 mg/kg bolus followed by 100 mg/kg per hour) may assist by binding with cyanide to form nontoxic cyanocobalamin; or sodium thiosulfate (150 to 200 mg/kg slowly) with sodium nitrite (4 to 6 mg/kg, slowly) assists in converting hemoglobin to methemoglobin, which competes with cytochrome oxidase for cyanide (Friederich and Butterworth, 1995). Deliberate hypotension has become a useful and widely accepted technique in adults and children as experience has accumulated with its use over more than a generation. Still, the clinician must weigh the potential benefits against the risks associated with hypotensive anesthetic techniques.
Miscellaneous Techniques for Reducing Intraoperative Blood Loss
Vasoconstriction, positioning, and tourniquets are used to reduce operative blood loss and to optimize the surgical field. Tissue infiltration with epinephrine 1:200,000 as a vasoconstrictor for blood conservation has had positive outcomes in adult breast surgeries, but there are no reports from the pediatric population (Brantner and Peterson, 1985; Courtiss, 1987). Animal studies suggest that blood loss is limited for the duration of the epinephrine’s effect, but delayed bleeding may occur (Rey et al., 1996). Karl et al. (1983) evaluated the safety of subcutaneous epinephrine infiltration under halothane anesthesia and reported that epinephrine, 10 mcg/kg, was safe in the pediatric population and that children tolerate a higher dose than adults.
The reverse Trendelenburg position, or head-up tilt position, is often used in head and neck surgery to minimize blood loss and to ensure a dry surgical field. Orthostatic stress experiments on healthy, awake, adult subjects have shown a decrease in cardiac output of up to 25% while maintaining mean arterial blood pressure. However, blood pressure is maintained by a baroreceptor reflex, and volatile anesthetics decrease the sensitivity of this reflex (Muzi and Ebert, 1995; Cooper and Hainsworth, 2001). Quantitative data are not available for the graded change in circulatory responses to various degrees of the reverse Trendelenburg position and the loss of compensatory baroreceptor reflex during general anesthesia in adults or children.
The use of pneumatic tourniquets after exsanguination of the upper or lower extremities significantly improves the operative conditions, particularly for cementing of bone (Abdel-Salam and Eyres, 1995; Wakankar et al., 1999). However, although tourniquet use as a blood conservation strategy is widely accepted in adults and children, there are potential risks, including nerve injury, increased infection, vascular injury, and cardiac and pulmonary injury (Estebe and Malledant, 1996; Kaabachi et al., 2005). In pediatric orthopedic surgery, the use of a tourniquet was identified by Kaabachi et al. (2005) as a risk factor for postoperative wound infection. The use of wide contour cuffs may reduce the likelihood of injury by decreasing mean tourniquet cuff pressure without compromising the quality of the surgical field (Reilly et al., 2009). In children with sickle cell disease (SCD) who require an extremity procedure, tourniquets have been used safely, provided that moderate hyperventilation with pH 7.40 to 7.45 and excellent oxygenation are maintained throughout the procedure (Adu-Gyamfi et al., 1993).
Transfusion triggers
In 2005, approximately 29 million units of RBC and non-RBC blood components were transfused. Specific data are not available for the pediatric population, but at least one study indicates that approximately 1% of transfusions are administered to patients younger than 18 years (Sullivan et al., 2002). Although the present blood supply is safer than ever in terms of preventing transfusion-mediated infection, complications from blood component transfusions are not uncommon. Therefore, decisions about transfusion for any patient should weigh the potential benefits of transfusion against the risks, the occurrence of which may be unknown. Expert guidelines published for adult and pediatric patients all call for clinical judgments by the practitioner. As a result, transfusion practices vary considerably (Blanchette et al., 1991; Stehling et al., 1994; American Society of Anesthesiologists Task Force on Blood Component Therapy, 1996; Roseff et al., 2002; Desmet and Lacroix, 2004). The following sections summarize the existing guidelines and literature related to pediatric patients.
Transfusion of Fresh Whole Blood or Reconstituted Whole Blood
The transfusion of fresh whole blood (less than 5 days old) would appear to be the obvious choice for resuscitation of a bleeding child, because it replaces all the lost components. However, the storage of whole blood is problematic. In particular, factors V and VIII are especially labile and tend to be rapidly depleted (Brecher, 2002). Also, refrigerated platelets lose their discoid shape, resulting in decreased survival (Murphy and Gardner, 1969). These factors, combined with the desire to optimize the number of usable components from only one donor and to treat patient-specific needs, have led to special requests for fresh whole blood, if available, in most centers (Roseff et al., 2002).
If fresh whole blood is not available but desired, stored RBCs can be reconstituted with fresh frozen plasma (FFP) (Roseff et al., 2002). This method of administering whole blood, however, provides no platelets and exposes the patient to twice as many donors. Few clinical scenarios require whole blood (Box 14-1). For massive transfusion, it can probably be used without further justification (Blanchette et al., 1991). However, for acute but controllable blood loss, particularly in the larger child, replacement with RBCs, crystalloid fluids, and colloid fluids are probably sufficient unless the clotting factors become excessively diluted (greater than 1 blood volume of replacement in adults). If that happens, plasma or platelets (or both) may be administered as needed.
Transfusion of red blood cells
There are only two accepted indications for the transfusion of RBCs: to increase oxygen-carrying capacity or avoid an impending inadequate oxygen-carrying state; and to suppress the production or dilute the amount of endogenous hemoglobin in selected patients with thalassemia or sickle cell disease (Hume and Limoges, 2002). Because neonates, particularly premature low–birth-weight patients, differ from infants and older children in terms of blood volume and physiology, they are discussed separately.
Transfusion in Patients Younger than 4 Months
Beside having a smaller absolute blood volume than older patients, children younger than 4 months have different physiologic processes than older patients. Examples include the premature infant’s limited erythropoietin response to decreased oxygen delivery, as previously discussed, and the newborn’s inefficient humoral system that precludes formation of antibodies to allogenic RBCs (Stockman et al., 1984; DePalma and Luban, 1990). These young patients perhaps receive the most transfusions of all patient groups; infants receiving multiple transfusions are exposed to blood from approximately 10 different donors (Sacher et al., 1989; Strauss, 1991). Recent work has focused both on delineating guidelines for transfusion and on limiting the number of donors needed for these young patients.
Replacement of iatrogenic blood losses caused by blood draws for laboratory testing has historically accounted for approximately 90% of the transfusions in neonates (Kaabachi et al., 2005). Older guidelines and expert opinions have advocated replacing blood losses resulting from phlebotomy when losses exceed 5% to 10% of the total blood volume (Blanchette et al., 1991; Wakankar et al., 1999). These guidelines note that the decision to transfuse should not be made solely on the amount of blood lost but should include factors such as the clinical status of the patient, the patient’s hemoglobin level, and the length of time over which the losses occurred (Blanchette et al., 1991). Currently transfusion simply to replace phlebotomy losses is much less common (Ramasethu, 1999; Simon et al., 1998; Shannon et al., 1995).
Maintenance of hematocrit levels greater than 35% (hemoglobin greater than 13 g/dL) for neonates with severe respiratory disease continues to be common practice without the support of evidence (Roseff et al., 2002). In a study of 10 oxygen-dependent infants with bronchopulmonary dysplasia, small-volume transfusions increased oxygen transport and decreased oxygen extraction. However, hemoglobin levels alone were not predictive of which children would benefit from transfusion (Alverson et al., 1988).
Transfusion in Patients Older than 4 Months
The transfusion practice guidelines of the American Society of Anesthesiologists Task Force on Blood Component Therapy (1996) are largely applicable to pediatric patients without cardiopulmonary disease. The points about RBC transfusions are summarized as follows:
Other guidelines have also been published specifically for pediatric patients older than 4 months (Roseff et al., 2002; Stehling et al., 1994) (Box 14-2).
Box 14-2 Guidelines for Transfusion of Red Blood Cells in Patients Older Than 4 Months
Modified from Roseff SD, Luban NL, Manno CS: Guidelines for assessing appropriateness of pediatric transfusion, Transfusion 42(11):1398-1413, 2002.
Although numerous studies have been performed to delineate optimal hemoglobin levels in critically ill adults, few studies have been performed involving children. Traditionally, a hemoglobin level greater than 10 g/dL has been advocated for critically ill patients. In a landmark article by Hebert et al. (1999), a trial randomly assigned patients to receive RBC transfusions according to a liberal strategy (transfusion for hemoglobin less than 10 g/dL) or a restrictive strategy (transfusion for hemoglobin less than 7 g/dL). Although there was a higher in-hospital mortality rate in the liberally transfused group, there was no difference between the groups in the 30-day mortality rate. The liberal strategy group also tended to have higher estimates of severity of multiple organ dysfunction and nosocomial infections.
The Hebert article spawned an interest in performing a similar study in children. The Transfusion Requirements in the Pediatric Intensive Care Unit (TRIPICU) trial was published in 2007 (Lacroix et al., 2007). In this trial, 637 infants and children in the intensive care unit were randomly assigned to a liberal strategy (transfusion for hemoglobin less than 9.5 g/dL) or a restrictive strategy (transfusion for hemoglobin less than 7 g/dL). There was no difference between the groups in the percentage of patients with new or progressive multiple organ dysfunction syndrome (the primary outcome) or death. However, patients in the restrictive strategy group received 44% fewer transfusions than did those in the liberal strategy group. This study suggests that in stable, critically ill children, a transfusion threshold of 7 g/dL decreases transfusion requirements without increasing adverse outcomes.
Clinicians should remember that the goal of RBC transfusion is to ensure adequate oxygen delivery to the tissues. The clinical picture of the patient is at least as important as the hemoglobin level in determining whether RBC transfusion is required or justified. At the minimum, findings of tachycardia or tachypnea, decreased urine output, or cool vasoconstricted extremities may indicate the need for increased oxygen-carrying capacity. Alternatively, acid-base balance or blood lactate levels can be monitored. More recently, central venous catheters that continually report mixed venous oxygen saturation have been developed in sizes appropriate for children. In addition, noninvasive cerebral oximetry measurements correlate well with measured mixed venous oxygenation in some patients (Cua et al., 2004; Tortoriello et al., 2005). In the future, these and other monitors under development may allow clinicians to better individualize treatment and predict which patients will benefit from RBC transfusion.
When the decision has been made to transfuse RBCs into a child, the appropriate volume must then be determined. Babies in the intensive care unit often receive “top-up” transfusions for slowly progressive anemia caused by phlebotomy. Typically, the dose is 10 to 15 mL/kg administered over 2 to 3 hours. For the larger child in the operating room, an estimate of allowable blood losses may be calculated before any procedure during which blood loss is expected. This can be accomplished with the patient’s estimated blood volume (EBV), weight, initial hematocrit (Hi), and lowest allowable hematocrit (Hp) (Table 14-2). For example, the maximal allowable blood loss in a 5-kg infant with an Hi of 40% and in whom a hematocrit value of 24% is determined safe would be calculated as follows:
Age Group | Estimated Blood Volume (mL/kg) |
Premature infants | 90-100 |
Term newborns | 80-90 |
Infants younger than 1 yr | 75-80 |
Older children | 70-75 |
A simpler method of calculating allowable blood loss uses the Hi, the Hp, and the average of the two hematocrits (Hav) as follows (Desmet and Lacroix, 2004):
RBC Transfusion for Uncorrected Cyanotic Congenital Heart Disease
Children with uncorrected cyanotic congenital heart disease deserve special mention. Intuitively, cyanotic patients require a higher oxygen-carrying capacity to maintain adequate oxygen delivery in the presence of mixing lesions. Indeed, in the absence of phlebotomy in these children, polycythemia develops because of increased erythropoietin in response to poor tissue-oxygen tension. The usual recommendation for these children is to use transfusion to maintain hemoglobin concentrations of 13 to 18 g/dL, although support for this practice is lacking (Meliones et al., 1995; Stehling et al., 1994; Paridon, 1998; Lacroix et al., 2007). Anecdotal evidence from patients of the Jehovah’s Witness faith who underwent operations to repair congenital heart disease suggests that lower hemoglobin levels may be tolerated in cyanotic patients (Kawaguchi et al., 1984; Henling et al., 1985).
Transfusion in patients with cyanotic heart disease may have effects beyond increasing oxygen-carrying capacity. Beekman and Tuuri (1985) studied seven children with right-to-left shunts in the catheterization laboratory. Partial exchange transfusions were used to increase the mean (shown with SD) baseline hemoglobin of 13.7 (0.5) g/dL to 16.4 (0.4) g/dL. This intervention not only increased the arterial oxygen saturation; it also decreased the degree of shunting by 59%. Presumably the decrease in shunting resulted from an increase in systemic vascular resistance as a result of an increase in blood viscosity.
RBC Transfusion for Sickle Cell Disease
Patients with SCD often have perioperative complications or a crisis. Early reports cited perioperative mortality rates of 10% and an incidence of postoperative complications of up to 50% (Vichinsky et al., 1995). The two indications for RBC transfusion in a patient with SCD are correction of a preexisting anemia and dilution of hemoglobin S concentration. Some experts advise a preoperative dilution of hemoglobin S levels to less than 30% (Bhattacharyya et al., 1993). However, this practice has never been shown to be of clear benefit.
To more completely address this controversy, Vichinsky and colleagues (1995) performed a multicenter, randomized trial that compared a conservative transfusion strategy (transfusion to increase the hemoglobin level to 10 g/dL) with an aggressive transfusion strategy (transfusion to decrease hemoglobin S to less than 30%). The patients underwent a total of 604 operations. The groups were well matched, and the mean preoperative hemoglobin levels were 10.6 g/dL in the conservative group and 11.0 g/dL in the aggressive group. When transfusion-associated complications were excluded, there was no difference in the incidence of major complications (e.g., acute chest syndrome, painful crisis, neurologic event, or death) even though the conservatively treated group had a higher concentration of hemoglobin S (59% vs. 31%). However, the incidence of transfusion-related complications was twice as high in the aggressively treated group (14% vs. 7%). These authors concluded that a transfusion protocol aimed at correcting anemia to a hemoglobin of 10 g/dL was as effective as a protocol designed to decrease the hemoglobin S concentration to less than 30% and resulted in half as many transfusion-related complications
The incidence of complications in patients with SCD varies widely with the type of operation. In a review by Koshy et al. (1995) of 1,079 procedures, the rates of perioperative complications were 0% for tonsillectomy and adenoidectomy, 2.9% for hip surgery, 3.9% for myringotomy, 7.8% for abdominal surgery, 16.9% for cesarean section and hysterectomy, and 18.6% for dilation and curettage. Patient characteristics that appear to be associated with increased postoperative complications include increased age, incidence of recent complications, inpatient hospital status, pregnancy, and preexisting infection (Firth and Head, 2004).
Transfusion of fresh frozen plasma
FFP is no longer indicated for volume replacement (Consensus conference, 1985; Northern Neonatal Nursing Initiative Trial Group, 1996). If colloid therapy is thought to be more beneficial than crystalloid therapy for volume replacement, albumin or a starch-based product such as hetastarch (≤ 20 mL/kg) may be used. In addition, nearly all congenital bleeding disorders can now be treated with factor-specific therapies and should not be treated with FFP or cryoprecipitate unless these targeted therapies are unavailable. The typical dose of FFP in the pediatric patient is 10 to 20 mL/kg (American Society of Anesthesiologists Task Force on Blood Component Therapy, 1996; Hume and Limoges, 2002; Roseff et al., 2002).
Transfusion of platelets
Guidelines for the infusion of platelets in pediatric patients resemble those for adults (American Society of Anesthesiologists Task Force on Blood Component Therapy, 1996). If the rest of the coagulation system is normal, platelet counts greater than 50 ×109/L are probably sufficient for invasive procedures (McVay and Toy, 1991). If patients undergoing invasive procedures have platelet counts between 50 × 109/L and 100 × 109/L, platelets should be readily available for transfusion if needed. For massive transfusion with microvascular bleeding, platelet counts should be obtained before transfusion if possible. However, platelets may be given during a massive transfusion if a platelet count is not readily obtainable and there is evidence of microvascular bleeding. Platelet transfusions are probably not indicated when thrombocytopenia is caused by increased platelet destruction (idiopathic thrombocytopenic purpura) (American Society of Anesthesiologists Task Force on Blood Component Therapy, 1996).
Preterm infants who are critically ill are of great concern, because they are at high risk of intracranial hemorrhage. These patients have an underdeveloped subependymal matrix that is predisposed to rupture (Sacher et al., 1989; Strauss, 1991). Some experts recommend platelet transfusion for sick preterm babies when the platelet count is less than 100 × 109/L and for healthy preterm babies when the platelet count is less than 50 × 109/L. Andrew and colleagues (1993) randomly assigned 152 preterm thrombocytopenic babies to receive platelet transfusions to maintain a platelet count greater than 150 × 109/L (treatment group) or greater than 50 × 109/L (conventional group). The authors found no difference in the incidence of intracranial hemorrhage between the two groups (28% in the treatment group vs. 26% in the conventional group). They concluded that early infusion of platelet concentrates in thrombocytopenic premature infants did not alter the incidence of intracranial hemorrhage.
Platelets can be recovered from donors in two ways. One method is to remove whole blood from the donor and use centrifugal forces to separate its constituents. This results in the recovery of approximately 5.5 ×1010 platelets in 50 mL of plasma per 450 mL of whole blood removed from the donor. Typically, these platelet concentrates are then pooled with those recovered from other donors in groups of 6 to 8 for transfusion. Alternatively, apheresis can be used. This method draws blood into a circuit, separates the components with centrifugation or filtration, collects the desired blood component, and returns the remaining components to the donor. A single apheresis unit results in a similar number of platelets as 6 to 8 pooled platelet concentrates from whole blood, with the obvious advantage of exposure to only one donor (Fasano and Luban, 2008).
Transfusion-associated complications
Viral Infections
The current blood supply is safer than ever from the risks of transmitting viral infections. The estimated risk of transmission for some of the major viruses is shown in Table 14-3. The incidence of viral transmission is now too low to measure, so mathematical models are used to estimate risk (Goodnough et al., 1999a). Improvement in the safety of the blood supply has been multifactorial. After early descriptions of human immunodeficiency virus (HIV) transmission through blood transfusion in 1982 and 1983, the U.S. Public Health Service recommended that persons at increased risk of HIV not donate blood (Ammann et al., 1983). In addition, blood banks began asking donors about high-risk behaviors and giving donors the opportunity to specify that their blood should not be used (Hebert et al., 1999; Tortoriello et al., 2005). These simple interventions alone resulted in significant decreases in the incidence of transfusion-related HIV infection (Busch et al., 1991). With current donor-screening techniques and modern testing of donated blood, which includes antibody screening and nucleic acid testing, patients can generally be assured that the risk of acquiring a major viral infection is quite low. However, new infectious risks, such as exposure to West Nile virus, continue to emerge and will require ongoing surveillance to ensure the safety of the blood supply (Dodd, 2003a; 2003b).
Disease | Incidence with Transfusion | Comments |
Hepatitis B virus | 1 in 137,000 | Decreasing because of vaccination of health care professionals and school-aged children. |
Hepatitis C virus | <1 in 1,000,000 | Antibody screening began in 1990; NAT testing was added in 1999. |
Human immunodeficiency virus | <1 in 1,900,000 | First blood test was available in 1985; NAT testing was universal in 1999. |
Human T lymphotropic virus types I and II | 1 in 250,000 to 1 in 2,000,000 | Viruses cause blood or nervous system disease in few infected patients. |
NAT, Nucleic amplification testing.
Data from Goodnough LT et al.: Transfusion medicine: blood transfusion, parts 1 and 2, N Engl J Med, 340(6, 7):438,525, 1999, and AABB Buyers’ Guide: Transfusion-transmitted diseases (website): http://www.aabb.org/Content/About_Blood/Facts_About_Blood_and_Blood_Banking/fabloodtrans.htm. Accessed April 2007.
Bacterial Infections
There has been an increasing focus on the bacteriologic safety of the blood supply. Between 1985 and 1999 in the United States, 694 transfusion-related deaths were reported to the FDA. Of these, 11.1% were attributed to bacterial contamination (Dodd, 2003a). The most likely bacterial contaminant of stored RBCs is Yersinia enterocolitica, but other gram-negative bacteria are possible as well (CDC, 1997). The risk of contamination is directly related to the duration of storage (Goodnough et al., 1999a; 1999b). In the United States, the risk of bacterial infection from the transfusion of RBCs is less than 1 in 1 million, but the mortality rate from infection is high: 12 of 20 patients who received RBCs infected with Yersinia died from 1987 to 1996 (Goodnough et al., 1999a; Dodd, 2003a). Symptoms of infection usually begin during the transfusion, and patients who die usually die within the next 24 hours.
The risk of sepsis from platelets is higher than from stored RBCs because platelets are stored warm. For this reason, the shelf life of platelets stored at 20° C to 24° C is only 5 days. The reported incidence of sepsis after platelet transfusion is 10 to 138 cases per million transfusions, and risk of death from platelet-associated infection is 2 to 18 per million transfusions (Kuehnert et al., 2001; Ness et al., 2001). There is evidence that the risk of infection is higher when pooled donors are used than when an apheresed unit is transfused from only one donor. The most common bacteria causing death after platelet transfusion are Staphylococcus aureus, Klebsiella pneumoniae, Serratia marcescens, and Staphylococcus epidermidis (Beekman and Tuuri, 1985). The clinical course for platelet-related bacterial infection is more variable than that associated with RBCs. Symptoms range from mild fever to florid sepsis with hypotension and death. The reported mortality rate is 26% (Goldman and Blajchman, 1991).
Hyperkalemia
There have been several reports of transfusion-associated hyperkalemic cardiac arrest in pediatric patients (Hall et al., 1993; Ivens and Camu, 1996; Buntain and Pabari, 1999; Chen et al., 1999; Smith et al., 2008). The RBC membrane is poorly permeable to potassium and relies on energy-dependent mechanisms to move potassium in and out of the cell. As RBCs age during storage, adenosine triphosphate synthesis and potassium pumping decrease, and potassium leaks into the supernatant (Ronquist and Waldenstrom, 2003). Indeed, among units stored for prolonged periods, the potassium concentration in the supernatant may exceed 60 mEq/L (Hall et al., 1993). In an analysis of 74 units of banked blood at the Mayo Clinic, Smith and colleagues (2008) found a range of potassium concentrations, from 7.3 to 77.2 mEq/L. In the first week of storage, the average (with SD) measured potassium was 19.0 (7.8) mEq/L; during the second week, 31.5 (14.1) mEq/L; and between 15 and 28 days of storage, 39.9 (10.3) mEq/L. Intraoperative washing of packed RBCs through autotransfusion and use of blood salvage devices may be effective at decreasing the potassium burden (Knichwitz et al., 2002). Alternatively, if notified in advance, blood banks can provide reduced-potassium blood. However, the emergent nature of many massive transfusions often precludes these interventions.
Hyperkalemic arrest seems to be more common among children than adults (Smith et al., 2008). This difference is likely because of smaller circulating volumes, immature renal function, and differences in autonomic tone. In most published reports, some degree of acidosis, hyperglycemia, or hypothermia was present before the arrest. Therefore, it is difficult to sort out the roles of these factors in augmenting the toxicity of potassium associated with the transfusion. Regardless, the combination of all these factors results in extremely high mortality for patients with transfusion-associated hyperkalemic arrest (87.5% mortality in one series [Smith et al., 2008]).
Immunomodulation
Allogenic blood transfusions seem to lead to modulation of the immune system. Indeed, targeted transfusions were initially used in the 1970s for immunosuppression after renal transplants, but the effect of transfusions on long-term outcome is still not understood (Opelz et al., 1973). More than 100 studies have been published on perioperative transfusion and postoperative infection (Mauermann and Nemergut, 2006). Although many of the conclusions were limited by the biases of small cohorts and retrospective studies, several prospective series showed that perioperative transfusion increased the infection rate in various settings, including cardiac surgery, orthopedic surgery, trauma, and colorectal surgery (Ammann et al., 1983; Busch et al., 1991; Goodnough et al., 1999a; Dodd, 2003). However, the transfusion literature remains divided on the subject of transfusion-associated infections; for every study with positive results, a study with negative results can also be found. The situation is similar for the risk of cancer recurrence after a presumed curative operation. In a 1996 article, Landers et al. (1996) reviewed the available studies, which included various surgical patients. The authors concluded that the burden of evidence pointed toward an association between transfusion and cancer recurrence. Other experts continue to argue that this is simply an association and not a causal relation (Vamvakas and Blajchman, 2001).
Most of the discussion surrounding immunosuppression and transfusion focuses on the effects of donor leukocytes (Kuehnert et al., 2001). Leukoreduction is thought to help reduce transfusion-associated nosocomial infections. Hebert and colleagues (2003) retrospectively reviewed the effects of a universal leukoreduction program in 23 Canadian centers. The patients were undergoing cardiac, orthopedic, or trauma surgery. Although leukoreduction appeared to decrease mortality and the incidence of antibiotic use, the overall rate of serious nosocomial infections was not affected. Fergusson et al. (2004) performed a meta-analysis of all available trials that used leukoreduction and found no difference in the infection rate. Although most of the trials studying this issue have been performed with adult patients, at least one analysis of patients in a pediatric intensive care unit suggested that increased numbers of RBC transfusions were independently associated with an increased incidence of bloodstream infections in critically ill children (Elward and Fraser, 2006).
Transfusion-Associated Graft-Versus-Host Disease
Not only can transfusions depress immune function, they also may activate it in adverse ways, as in the case of transfusion-associated graft-versus-host disease (TA-GVHD), a preventable disease associated with high mortality. Typically, adults with TA-GVHD show symptoms 10 days after transfusion and neonates with TA-GVHD experience symptoms 28 days after transfusion (Parshuram et al., 2002). The clinical features include fever, rash, diarrhea, hepatitis, and pancytopenia, with death occurring approximately 50 days after transfusion (Ohto and Anderson, 1996). Treatments include corticosteroids, azathioprine, methotrexate, cyclosporine, and bone marrow transplants. However, even with aggressive treatment, the mortality rate in children is nearly 100% (Leitman and Holland, 1985).
The pathophysiology of TA-GVHD has been described (Parshuram et al., 2002). Circulating donor leukocytes persist in transfusion recipients for up to 18 months (Lee et al., 1999). Normally these cells are recognized as foreign and are eliminated, but if they are not, donor leukocyte engraftment may occur, with proliferation of CD4 and CD8 T cells (Nishimura et al., 1997). The clinical effects are mediated by donor T-cell cytotoxicity and by recipient cytokines, including tumor necrosis factor α and interleukin 1 (Vogelsang and Hess, 1994).
Because TA-GVHD is mediated by donor leukocytes, it is preventable by removal of the same. Washing of donor RBCs and use of leukoreduction filters decrease the donor leukocyte burden but do not eliminate all the leukocytes. These methods may be effective at reducing the incidence of TA-GVHD, but they do not eliminate its occurrence (Ronquist and Waldenström, 2003; Smith et al., 2008). Gamma irradiation of 2,500 cGy is the only accepted and widely available method of preventing TA-GVHD. This degree of irradiation decreases the recovery of RBCs, so that the FDA recommends a shelf life of 28 days for irradiated RBCs compared with 42 days for nonirradiated packed RBCs (Davey et al., 1992). Potassium and free hemoglobin levels increase after irradiation and increase further with the duration of storage. Thus, it is preferable to irradiate blood products shortly before administration, particularly in small children, who may not tolerate a potassium load.
Transfusion-Related Acute Lung Injury
Transfusion-related acute lung injury (TRALI) is the leading cause of transfusion-related death in the United States (Kleinman et al., 2004). Although underreporting surely exists, the estimated risk of TRALI is one in 5000 transfusions, with FFP being the most commonly associated product (Goodnough et al., 1999a). This complication and its fatal consequences have been described in pediatric patients (Ririe et al., 2005). The originally proposed mechanism of TRALI is an interaction between antibodies from the donor and leukocyte antigens from the recipient (Opelz et al., 1973). Donor lipids and cytokines have also been implicated. Regardless of the mechanism, the end result is activation of recipient neutrophils in the pulmonary vascular bed. Neutrophil and complement activation culminates in increased pulmonary capillary permeability and pulmonary edema. Interestingly, the most commonly implicated donors are multiparous females, presumably as a result of an increase in human leukocyte antibodies to paternal antigens during pregnancy (Densmore et al., 1999).
The clinical features of TRALI are similar to those of acute respiratory distress syndrome and include dyspnea, hypoxia, and radiographic evidence of pulmonary edema without increased pulmonary capillary wedge pressure. Onset of symptoms usually occurs within 4 to 6 hours after transfusion. Treatment is supportive, because there is no evidence that corticosteroids or other specific therapy is helpful. It is estimated that 90% of patients with TRALI recover (Hebert et al., 1999). The majority of reported cases and studies of TRALI involve adults, but children are certainly at risk (Ririe et al., 2005; Church et al., 2006).
Massive transfusion
Massive transfusion is typically defined as the need to transfuse more than 1 blood volume in a 24-hour period and, in the case of trauma, portends a poor prognosis (Vaslef et al., 2002; Como et al., 2004). Several potential complications of massive transfusion are listed in Table 14-4. The cause of coagulopathy during massive blood loss and resuscitation continues to be debated and is probably different for relatively controlled losses in the operating suite than for uncontrolled acute losses caused by trauma (Ketchum, 2006). The dilution and consumptive loss of coagulation factors (especially platelets) appear to vary widely. However, after losing 2 blood volumes, most adult patients have platelet counts less than 50 ×109/L, and many require correction of symptomatic thrombocytopenia before the platelet count decreases to that level (Hiippala et al., 1995). In otherwise healthy adults, the intraoperative loss of about 1.5 blood volumes requires replacement of coagulation factors with FFP. This is in contrast to trauma patients who appear to benefit from FFP administration early in the course of resuscitation (Ketchum, 2006; Shaz et al., 2009).
Complication | Mechanism |
Acidosis | Poor oxygen delivery, lactate accumulation |
Alkalosis | Citrate metabolism to bicarbonate by the liver |
Hypocalcemia | Citrate binding of calcium |
Hyperglycemia | Dextrose preservative in packed red blood cells |
Hypothermia | Transfusion of cold blood products |
Hyperkalemia | Multifactorial |
Data from Smith HM et al.: Cardiac arrests associated with hyperkalemia during red blood cell transfusion: a case series, Anesth Analg 106(4):1062-1069, 2008.
It is difficult to conduct quality studies with patients experiencing major intraoperative blood loss, because measurements of blood loss, assumptions of intravascular volume status, and subjective observations of hemostasis are potential sources of error and bias. However, at least one observational study has attempted to identify the source of coagulopathy in pediatric patients undergoing a major operation. Murray and colleagues (1995) studied 32 patients (mean [SD] age, 15.6 [2.3] years) who lost more than 50% of their blood volume while undergoing major spine surgery and were resuscitated with packed RBCs and crystalloid solutions. In 17 of these 32 patients, coagulopathy developed, and it was felt that treatment with FFP was required. Patients with increased clinical bleeding had more blood loss and blood volume loss than the patients who did not have increased clinical bleeding. Although 30 of the 32 patients had increased prothrombin times and activated partial thromboplastin times, only 17 of these patients had excessive clinical bleeding. After loss of 1 blood volume, platelet counts estimated by regression analysis were 195,000/mL. In 14 of the 17 patients with excessive bleeding, FFP alone was adequate to achieve hemostasis. Two patients received platelets as well. The authors concluded that in pediatric patients undergoing spinal fusion, coagulation factor dilution rather than thrombocytopenia is the major cause of coagulopathy and in the majority of cases FFP is the only blood component needed to correct the coagulopathy.
Whenever possible, the use of platelet and FFP transfusions should be guided by laboratory values, but even in an efficient hospital, prothrombin time results may not be available for 45 to 60 minutes, making laboratory guidance for blood component therapy difficult. For controlled blood loss in the operating room, therefore, the clinician must anticipate the need for blood products and evaluate coagulation status at regular intervals. As point-of-care testing becomes more widely available, values for prothrombin time and partial thromboplastin time may be accessible more quickly. Recently, thromboelastography has become an increasingly popular measure of dynamic clotting parameters (Fig. 14-13). Thromboelastography assesses the viscoelastic properties of blood under low shear conditions (Ganter and Hofer, 2008). Devices from different manufacturers use different technologies. However, the premise is similar. A pin is immersed in a blood sample. The blood sample or pin is then rotated through a specific cycle. As clot begins to form in the blood sample, torque is applied to the immersed pin. The changes in torque are transmitted to a recording device. The devices record changes in viscoelasticity at all stages of clot formation. The terminology varies between devices, but the important measurements and the corresponding stages of clot formation for the TEG system (Haemoscope Corp, Niles, Illinois) are shown in Table 14-5 as an example (Ganter and Hofer, 2008).
TABLE 14-5 Measurements and Corresponding Stages of Clot Formation for the TEG System*
Output | Stage of Clot Formation | Potential Causes of Abnormal Results |
Reaction time | Initial fibrin formation | Factor deficiency, heparin |
Alpha angle | Kinetics of fibrin formation and clot development | Fibrinogen deficiency |
Maximum amplitude | Ultimate strength and stability of clot | Platelet deficiency or dysfunction |
Clot lysis | Clot lysis | Fibrinolysis |
Intraoperative blood recovery
Cell salvage devices are made by several manufacturers, but they all operate under the same general principles: centrifugal and hydrostatic forces are used to differentiate between blood constituents on the basis of their different densities (Waters, 2005). Suction is used to transfer blood from the surgical field to a collection reservoir. In the reservoir, the blood must be anticoagulated with either a heparin or a citrate-based anticoagulant. When a sufficient amount of blood has been captured for processing, a cycle is activated and the blood is drained into a centrifuge bowl. Blood enters the bowl while the centrifuge is spinning. Centrifugal forces separate the various blood components, with the larger, heavier RBCs forming sediment against the walls and the lighter particles (plasma) forming sediment closer to the center of the bowl. Hydrostatic forces then draw the plasma out through the center of the bowl. After separation of the RBCs, they are washed with a balanced salt solution to remove debris. Typically, the wash volume is at least three times the bowl volume. After washing, the RBCs are transferred to a collection bag for infusion into the patient (Waters, 2005).
The average hematocrit level of a properly processed salvaged unit is approximately 60%, and the survival of salvaged RBCs is reportedly similar to that of allogenic RBCs (Williamson and Taswell, 1991). The efficacy of intraoperative cell salvage depends largely on the efficiency of capturing the patient’s shed blood. High suction pressures lead to turbulence that may ultimately destroy the RBCs, so the lowest suction pressure that is effective at clearing the surgical field should be used. Suction tips with larger orifices further decrease turbulence on the RBCs. Because sponges may contain a considerable amount of shed blood, they should be rinsed and wrung out into a basin; the rinse solution is then drawn into the collection reservoir (Waters, 2005).
Studies evaluating the efficacy of intraoperative cell salvage in pediatric patients have had mixed results. One potential obstacle is the use of a large, adult-sized collection system for small children (Weldon, 2005). Although infants may lose a large portion of their blood volume during an operation, this loss is likely not of sufficient volume to allow processing. An exception is the use of an adult cell-salvage device to recover RBCs from the cardiopulmonary circuit after cardiac surgery. When a smaller, 125-mL bowl is used, the results have been more encouraging. One retrospective study found that cell salvage using a 125-mL collection bowl decreased the need for banked RBCs from 100% to 67% during craniosynostosis surgery (Jimenez and Barone, 1995). A second study, using an even smaller system that required only 35 mL of shed blood for processing, also showed a reduction in the exposure to donor RBCs (Dahmani et al., 2000). Not all cell salvage devices are equal. In a study by Booke and colleagues (1999), three cell-salvage devices were compared. Of the three devices, the Continuous Autotransfusion System (CATSplus) was the most efficient, providing the highest hematocrit and the largest volume of salvaged blood. The cost-benefit ratio of cell salvage is directly proportional to the number of units processed (Waters, 2005). Thus, with small patients, a large financial savings is unlikely to be realized. Regardless of cost, fewer donor exposures appears to have clear benefits.
Intraoperative cell salvage is not without risk. Transfer of blood directly from the holding bag carries the potential for air embolism, requiring that blood be transferred from the holding bag to a transfusion bag with the air removed except in extreme emergencies when time does not allow. The wash solution must be a balanced salt solution, because the inadvertent substitution of sterile water would cause massive cell lysis and the readministration of lysed cells could result in potentially fatal hyperkalemia. Given that washing does not completely remove bacteria from the captured blood, gross bacterial contamination of the surgical field is a relative contraindication to the use of cell salvage (Hebert et al., 2003). Other relative contraindications include the presence of malignant cells or contaminants such as ascitic fluid, amniotic fluid, and thrombin gel.
Blood substitutes
PFCs are inert substances that do not bind gases but act as solvents that can carry numerous gases, including oxygen. PFCs are insoluble in water and must be emulsified before administration. They are ultimately broken down by the reticuloendothelial system and exhaled as vapor; consequently they have a relatively short half-life (Tremper et al., 1982). Repeated administration results in hepatosplenomegaly in animals, and there appears to be a dose-dependent decrease in platelet count associated with administration (Leese et al., 2000). Because PFCs do not bind oxygen, their oxygen-carrying capacity is dependent on the partial pressure of oxygen. Patients must therefore receive high concentrations of inspired oxygen to have any meaningful oxygen delivery, and these high concentrations may carry the risk of pulmonary toxicity. In addition, unloading of oxygen at the tissue level is completely dependent on the oxygen gradients, and delivery tends to be poor. There are currently no FDA-approved PFCs, although third-generation PFCs are in preclinical development (Chang, 2000).
HBOCs were first studied in 1934 when Amberson purified bovine hemoglobin and administered it to animals (Jahr et al., 2007). These products consist of hemoglobin solutions that are polymerized or cross-linked (or both). When hemoglobin molecules are not contained by RBCs they rapidly scavenge nitric oxide, resulting in systemic (especially pulmonary) vasoconstriction, a release of proinflammatory mediators, and a loss of platelet inactivation (Wahr, 2003; Natanson et al., 2008). Cross-linking and polymerization of hemoglobin molecules has been proposed to increase the half-life of the molecules (free hemoglobin is rapidly scavenged by the reticuloendothelial system) and reduce nitric oxide-associated complications.
A meta-analysis of 16 trials that used five different HBOCs included a total of 3711 adult patients in various situations (Natanson et al., 2008). This analysis showed a significant increase in the risk of death (relative risk [RR], 1.30; 95% confidence interval [CI], 10.05 to 1.61) for patients treated with HBOCs. There was also a 2.7-fold increase in the risk of myocardial infarction for treatment patients (RR, 2.71; 95% CI, 1.67-4.40).
Summary
For questions and answers on topics in this chapter, go to “Chapter Questions” at www.expertconsult.com.
Abdel-Salam A., Eyres K.S. Effects of tourniquet during total knee arthroplasty: a prospective randomised study. J Bone Joint Surg Br. 1995;77(2):250-253.
Adu-Gyamfi Y., Sankarankutty M., Marwa S. Use of a tourniquet in patients with sickle-cell disease. Can J Anaesth. 1993;40(1):24-27.
Agarwal N., Murphy J.G., Cayten C.G., Stahl W.M. Blood transfusion increases the risk of infection after trauma. Arch Surg. 1993;128(2):171-176.
Akahoshi M., Takanashi M., Masuda M., et al. A case of transfusion-associated graft-versus-host disease not prevented by white cell-reduction filters. Transfusion. 1992;32(2):169-172.
Alverson D.C., Isken V.H., Cohen R.S. Effect of booster blood transfusions on oxygen utilization in infants with bronchopulmonary dysplasia. J Pediatr. 1988;113(4):722-726.
American Society of Anesthesiologists Task Force on Blood Component Therapy: a report on practice guidelines for blood component therapy. Anesthesiology. 1996;84(3):732-747.
Ammann A.J., Cowan M.J., Wara D.W., et al. Acquired immunodeficiency in an infant: possible transmission by means of blood products. Lancet. 1983;1(8331):956-958.
Andrew M., Vegh P., Caco C., et al. A randomized, controlled trial of platelet transfusions in thrombocytopenic premature infants. J Pediatr. 1993;123(2):285-291.
Beekman R.H., Tuuri D.T. Acute hemodynamic effects of increasing hemoglobin concentration in children with a right to left ventricular shunt and relative anemia. J Am Coll Cardiol. 1985;5(2 Pt 1):357-362.
Beris P., Munoz M., Garcia-Erce J.A., Thomas, et al. Perioperative anaemia management: consensus statement on the role of intravenous iron. Br J Anaesth. 2008;100(5):599-604. Epub 2008 Mar 27
Bhattacharyya N., Wayne A.S., Kevy S.V., Shamberger R.C. Perioperative management for cholecystectomy in sickle cell disease. J Pediatr Surg. 1993;28(1):72-75.
Blanchette V.S., Hume H.A., Levy G.J., et al. Guidelines for auditing pediatric blood transfusion practices. Am J Dis Child. 1991;145(7):787-796.
Blank J.P., Sheagren T.G., Vajaria J., et al. The role of RBC transfusion in the premature infant. Am J Dis Child. 1984;138(9):831-833.
Boldt J., Knothe C., Zickmann B., et al. Aprotinin in pediatric cardiac operations: platelet function, blood loss, and use of homologous blood. Ann Thorac Surg. 1993;55(6):1460-1466.
Boldt J., Knothe C., Zickmann B., et al. Comparison of two aprotinin dosage regimens in pediatric patients having cardiac operations: influence on platelet function and blood loss. J Thorac Cardiovasc Surg. 1993;105(4):705-711.
Boldt J., Zickmann B., Schindler E., et al. Influence of aprotinin on the thrombomodulin/protein C system in pediatric cardiac operations. J Thorac Cardiovasc Surg. 1994;107(5):1215-1221.
Booke M., Hagemann O., Van Aken H. Intraoperative autotransfusion in small children: an in vitro investigation to study its feasability. Anesth Analg. 1999;88:763-765.
Bradfield J.F., Bode A.P. Aprotinin restores the adhesive capacity of dysfunctional platelets. Thromb Res. 2003;109(4):181-188.
Brantner J.N., Peterson H.D. The role of vasoconstrictors in control of blood loss in reduction mammaplasty. Plast Reconstr Surg. 1985;75(3):339-341.
Brecher M. Technical manual, ed 14. Bethesda (MD): Association of Blood Banks, 2002.
Bryson G.L., Laupacis A., Wells G.A. Does acute normovolemic hemodilution reduce perioperative allogeneic transfusion? A meta-analysis: the International Study of Perioperative Transfusion. Anesth Analg. 1998;86(1):9-15.
Bulutcu F.S., Ozbek U., Polat B., et al. Which may be effective to reduce blood loss after cardiac operations in cyanotic children: tranexamic acid, aprotinin or a combination? Paediatr Anaesth. 2005;15(1):41-46.
Buntain S.G., Pabari M. Massive transfusion and hyperkalaemic cardiac arrest in craniofacial surgery in a child. Anaesth Intensive Care. 1999;27(5):530-533.
Busch M.P., Young M.J., Samson S.M., The Transfusion Safety Study Group. Risk of human immunodeficiency virus (HIV) transmission by blood transfusions before the implementation of HIV-1 antibody screening. Transfusion. 1991;31(1):4-11.
Centers for Disease Control and Prevention (CDC). Red blood cell transfusions contaminated with Yersinia enterocolitica: United States, 1991-1996, and initiation of a national study to detect bacteria-associated transfusion reactions. MMWR Morb Mortal Wkly Rep. 1997;46(24):553-555.
Chang T.M. Is there a need for blood substitutes in the new millennium and what should we expect in the way of safety and efficacy? Artif Cells Blood Substit Immobil Biotechnol. 2000;28(1):v-xi.
Chen C.H., Hong C.L., Kau Y.C., et al. Fatal hyperkalemia during rapid and massive blood transfusion in a child undergoing hip surgery: a case report. Acta Anaesthesiol Sin. 1999;37(3):163-166.
Church G.D., Price C., Sanchez R., Looney M.R. Transfusion-related acute lung injury in the paediatric patient: two case reports and a review of the literature. Transfus Med. 2006;16(5):343-348.
Como J.J., Dutton R.P., Scalea T.M., et al. Blood transfusion rates in the care of acute trauma. Transfusion. 2004;44(6):809-813.
Consensus conference. Fresh-frozen plasma: indications and risks. JAMA. 1985;253(4):551-553.
Cooper V.L., Hainsworth R. Carotid baroreceptor reflexes in humans during orthostatic stress. Exp Physiol. 2001;86(5):677-681.
Corwin H.L., Gettinger A., Fabian T.C., EPO Critical Care Trials Group. efficacy and safety of epoetin alfa in critically ill patients. N Engl J Med. 2007;357(10):965-976.
Corwin H.L., Gettinger A., Pearl R.G., EPO Critical Care Trials Group. efficacy of recombinant human erythropoietin in critically ill patients: a randomized controlled trial. JAMA. 2002;288(22):2827-2835.
Courtiss E.H. Gynecomastia: analysis of 159 patients and current recommendations for treatment. Plast Reconstr Surg. 1987;79(5):740-753.
Crosby L., Palarski V.A., Cottington E., Cmolik B. Iron supplementation for acute blood loss anemia after coronary artery bypass surgery: a randomized, placebo-controlled study. Heart Lung. 1994;23(6):493-499.
Cua C.L., Hoffman T.M., Taeed R., et al. Cerebral saturations trend with mixed venous saturations in patients undergoing extracorporeal life support. Perfusion. 2004;19(3):171-176.
Cushing H. Tumors of the nervus acusticus and the syndrome of the cerebellopontine angle. Philadelphia: Saunders, 1917.
Dahmani S., Orliaguet G.A., Meyer P.G., et al. Perioperative blood salvage during surgical correction of craniosynostosis in infants. Br J Anaesth. 2000;85(4):550-555.
Davey R.J., McCoy N.C., Yu M., et al. The effect of prestorage irradiation on posttransfusion red cell survival. Transfusion. 1992;32(6):525-528.
Degoute C.S. Controlled hypotension: a guide to drug choice. Drugs. 2007;67(7):1053-1076.
Degoute C.S., Ray M.J., Gueugniaud P.Y., Dubreuil C. Remifentanil induces consistent and sustained controlled hypotension in children during middle ear surgery. Can J Anaesth. 2003;50(3):270-276.
Densmore T.L., Goodnough L.T., Ali S., et al. Prevalence of HLA sensitization in female apheresis donors. Transfusion. 1999;39(1):103-106.
DePalma L., Luban N.L. Blood component therapy in the perinatal period: guidelines and recommendations. Semin Perinatol. 1990;14(5):403-415.
Desmet L., Lacroix J. Transfusion in pediatrics. Crit Care Clin. 2004;20(2):299-311.
Dodd R.Y. Bacterial contamination and transfusion safety: experience in the United States. Transfus Clin Biol. 2003;10(1):6-9.
Dodd R.Y. Emerging infections, transfusion safety, and epidemiology. N Engl J Med. 2003;349(13):1205-1206. Epub 2003b Sep 18
Downard C.D., Betit P., Chang R.W., et al. Impact of AMICAR on hemorrhagic complications of ECMO: a ten-year review. J Pediatr Surg. 2003;38(8):1212-1216.
Eaton M.P. Antifibrinolytic therapy in surgery for congenital heart disease. Anesth Analg. 2008;106(4):1087-1100.
Eberhart L.H., Folz B.J., Wulf H., Geldner G. Intravenous anesthesia provides optimal surgical conditions during microscopic and endoscopic sinus surgery. Laryngoscope. 2003;113(8):1369-1373.
Elward A.M., Fraser V.J. Risk factors for nosocomial primary bloodstream infection in pediatric intensive care unit patients: a 2-year prospective cohort study. Infect Control Hosp Epidemiol. 2006;27(6):553-560. Epub 2006 May 31
Estebe J.P., Malledant Y. Pneumatic tourniquets in orthopedics. Ann Fr Anesth Reanim. 1996;15(2):162-178. French
Fasano R., Luban N.L. Blood component therapy. Pediatr Clin North Am. 2008;55(2):421-445.
Fearon J., Weinthal J. The use of recombinant erythropoietin in the reduction of blood transfusion rates in craniosynostosis repair in infants and children. Plast Reconstr Surg. 2002;109(7):2190-2196.
Fergusson D., Khanna M.P., Tinmouth A., Hebert P.C. Transfusion of leukoreduced red blood cells may decrease postoperative infections: two meta-analyses of randomized controlled trials. Can J Anaesth. 2004;51(5):417-424.
Fergusson D.A., Hebert P.C., Mazer C.D., et al. BART Investigators: a comparison of aprotinin and lysine analogues in high-risk cardiac surgery. N Engl J Med. 2008;358(22):2319-2331.
Ferraris V.A., Ferraris S.P., Saha S.P., et al. Society of Thoracic Surgeons Blood Conservation Guideline Task Force; Society of Cardiovascular Anesthesiologists Special Task Force on Blood Transfusion. Perioperative blood transfusion and blood conservation in cardiac surgery: the Society of Thoracic Surgeons and The Society of Cardiovascular Anesthesiologists clinical practice guideline. Ann Thorac Surg. 2007;83(5 Suppl):S27-S86.
Firth P.G., Head C.A. Sickle cell disease and anesthesia. Anesthesiology. 2004;101(3):766-785.
Fontana J.L., Welborn L., Mongan P.D., et al. Oxygen consumption and cardiovascular function in children during profound intraoperative normovolemic hemodilution. Anesth Analg. 1995;80(2):219-225.
Forgie M.A., Wells P.S., Laupacis A., Fergusson D. International Study of Perioperative Transfusion (ISPOT) Investigators. Preoperative autologous donation decreases allogeneic transfusion but increases exposure to all red blood cell transfusion: results of a meta-analysis. Arch Intern Med. 1998;158(6):610-616.
Friederich J.A., Butterworth J.F.4th. Sodium nitroprusside: twenty years and counting. Anesth Analg. 1995;81(1):152-162.
Ganter M.T., Hofer C.K. Coagulation monitoring: current techniques and clinical use of viscoelastic point-of-care coagulation devices. Anesth Analg. 2008;106(5):1366-1375.
Goldman M., Blajchman M.A. Blood product-associated bacterial sepsis. Transfus Med Rev. 1991;5(1):73-83.
Goodnough L.T., Brecher M.E., Kanter M.H., AuBuchon J.P. Transfusion medicine: first of two parts: blood transfusion. N Engl J Med. 1999;340(6):438-447.
Goodnough L.T., Brecher M.E., Kanter M.H., AuBuchon J.P. Transfusion medicine: second of two parts: blood conservation. N Engl J Med. 1999;340(7):525-533.
Goodnough L.T., Grishaber J.E., Monk T.G., Catalona W.J. Acute preoperative hemodilution in patients undergoing radical prostatectomy: a case study analysis of efficacy. Anesth Analg. 1994;78(5):932-937.
Gross J.B. Estimating allowable blood loss: corrected for dilution. Anesthesiology. 1983;58(3):277-280.
Gruber E.M., Shukla A.C., Reid R.W., et al. Synthetic antifibrinolytics are not associated with an increased incidence of baffle fenestration closure after the modified Fontan procedure. J Cardiothorac Vasc Anesth. 2000;14(3):257-259.
Hall T.L., Barnes A., Miller J.R., et al. Neonatal mortality following transfusion of red cells with high plasma potassium levels. Transfusion. 1993;33(7):606-609.
Hayashi H., Nishiuchi T., Tamura H., Takeda K. Transfusion-associated graft-versus-host disease caused by leukocyte-filtered stored blood. Anesthesiology. 1993;79(6):1419-1421.
Hebert P.C., Fergusson D., Blajchman M.A., Leukoreduction Study Investigators. Clinical outcomes following institution of the Canadian universal leukoreduction program for red blood cell transfusions. JAMA. 2003;289(15):1941-1949.
Hebert P.C., Wells G., Blajchman M.A., Transfusion Requirements in Critical Care Investigators, Canadian Critical Care Trials Group. A multicenter, randomized, controlled clinical trial of transfusion requirements in critical care. N Engl J Med. 1999;340(6):409-417. Erratum in: N Engl J Med. 1999;340(13):1056.
Helfaer M.A., Carson B.S., James C.S., et al. Increased hematocrit and decreased transfusion requirements in children given erythropoietin before undergoing craniofacial surgery. J Neurosurg. 1998;88(4):704-708.
Helm R.E., Klemperer J.D., Rosengart T.K., et al. Intraoperative autologous blood donation preserves red cell mass but does not decrease postoperative bleeding. Ann Thorac Surg. 1996;62(5):1431-1441.
Henling C.E., Carmichael M.J., Keats A.S., Cooley D.A. Cardiac operation for congenital heart disease in children of Jehovah’s Witnesses. J Thorac Cardiovasc Surg. 1985;89(6):914-920.
Henry D.A., Carless P.A., Moxey A.J., et al. Pre-operative autologous donation for minimising perioperative allogeneic blood transfusion. Cochrane Database of Systematic Reviews. (2):2002. CD003602
Hiippala S.T., Myllyla G.J., Vahtera E.M. Hemostatic factors and replacement of major blood loss with plasma-poor red cell concentrates. Anesth Analg. 1995;81(2):360-365.
Hocker J.R., Saving K.L. Fatal aortic thrombosis in a neonate during infusion of epsilon-aminocaproic acid. J Pediatr Surg. 1995;30(10):1490-1492.
Houbiers J.G., van de Velde C.J., van de Watering L.M., et al. Transfusion of red cells is associated with increased incidence of bacterial infection after colorectal surgery: a prospective study. Transfusion. 1997;37(2):126-134.
Hume H.A., Limoges P. Perioperative blood transfusion therapy in pediatric patients. Am J Ther. 2002;9(5):396-405.
Ivens D., Camu F. Sudden hyperkalemia during cardiopulmonary bypass with hypothermic cardiac arrest in an infant. J Cardiothorac Vasc Anesth. 1996;10(2):258-260.
Jabbour N., Gagandeep S., Mateo R., et al. Transfusion free surgery: single institution experience of 27 consecutive liver transplants in Jehovah’s Witnesses. J Am Coll Surg. 2005;201(3):412-417.
Jahr J.S., Walker V., Manoochehri K. Blood substitutes as pharmacotherapies in clinical practice. Curr Opin Anaesthesiol. 2007;20(4):325-330.
Jepson J.H. Factors influencing oxygenation in mother and fetus. Obstet Gynecol. 1974;44(6):906-914.
Jimenez D.F., Barone C.M. Intraoperative autologous blood transfusion in the surgical correction of craniosynostosis. Neurosurgery. 1995;37(6):1075-1079.
Kaabachi O., Letaief I., Nessib M.N., et al. Prevalence and risk factors for postoperative infection in pediatric orthopedic surgery: a study of 458 children. Rev Chir Orthop Reparatrice Appar Mot. 2005;91(2):103-108. French
Karl H.W., Swedlow D.B., Lee K.W., Downes J.J. Epinephrine-halothane interactions in children. Anesthesiology. 1983;58(2):142-145.
Kawaguchi A., Bergsland J., Subramanian S. Total bloodless open heart surgery in the pediatric age group. Circulation. 1984;70(3 Pt 2):I30-I37.
Ketchum L., Hess J.R., Hiippala S. Indications for early fresh frozen plasma, cryoprecipitate, and platelet transfusion in trauma. J Trauma. 2006;60(6 Suppl):S51-S58.
Keyes W.G., Donohue P.K., Spivak J.L., et al. Assessing the need for transfusion of premature infants and role of hematocrit, clinical signs, and erythropoietin level. Pediatrics. 1989;84(3):412-417.
Klein H.G., Dodd R.Y., Ness P.M., et al. Current status of microbial contamination of blood components: summary of a conference. Transfusion. 1997;37(1):95-101.
Kleinman S., Caulfield T., Chan P., et al. Toward an understanding of transfusion-related acute lung injury: statement of a consensus panel. Transfusion. 2004;44(12):1774-1789.
Knichwitz G., Zahl M., Van Aken H., et al. Intraoperative washing of long-stored packed red blood cells by using an autotransfusion device prevents hyperkalemia. Anesth Analg. 2002;95(2):324-325.
Kolev K., Machovich R. Molecular and cellular modulation of fibrinolysis. Thromb Haemost. 2003;89(4):610-621.
Koshy M., Weiner S.J., Miller S.T., et al. Surgery and anesthesia in sickle cell disease: cooperative study of sickle cell diseases. Blood. 1995;86(10):3676-3684.
Krafte-Jacobs B., Levetown M.L., Bray G.L., et al. Erythropoietin response to critical illness. Crit Care Med. 1994;22(5):821-826.
Kuehnert M.J., Roth V.R., Haley N.R., et al. Transfusion-transmitted bacterial infection in the United States, 1998 through 2000. Transfusion. 2001;41(12):1493-1499.
Kumar R., Chakraborty I., Sehgal R. A prospective randomized study comparing two techniques of perioperative blood conservation: isovolemic hemodilution and hypervolemic hemodilution. Anesth Analg. 2002;95(5):1154-1161.
Lacroix J., Hebert P.C., Hutchison J.S., TRIPICU Investigators; Canadian Critical Care Trials Group; Pediatric Acute Lung Injury and Sepsis Investigators Network. Transfusion strategies for patients in pediatric intensive care units. N Engl J Med. 2007;356(16):1609-1619.
Landers D.F., Hill G.E., Wong K.C., Fox I.J. Blood transfusion-induced immunomodulation. Anesth Analg. 1996;82(1):187-204.
Laupacis A., Fergusson D. The International Study of Peri-operative Transfusion (ISPOT) Investigators. Erythropoietin to minimize perioperative blood transfusion: a systematic review of randomized trials. Transfus Med. 1998;8(4):309-317.
Leal-Noval S.R., Rincon-Ferrari M.D., Garcia-Curiel A., et al. Transfusion of blood components and postoperative infection in patients undergoing cardiac surgery. Chest. 2001;119(5):1461-1468.
Lee T.H., Paglieroni T., Ohto H., et al. Survival of donor leukocyte subpopulations in immunocompetent transfusion recipients: frequent long-term microchimerism in severe trauma patients. Blood. 1999;93(9):3127-3139.
Leese P.T., Noveck R.J., Shorr J.S., et al. Randomized safety studies of intravenous perflubron emulsion. I. Effects on coagulation function in healthy volunteers. Anesth Analg. 2000;91(4):804-811.
Leitman S.F., Holland P.V. Irradiation of blood products: indications and guidelines. Transfusion. 1985;25(4):293-303.
Lenes B.A., Sacher R.A. Blood component therapy in neonatal medicine. Clin Lab Med. 1981;1(2):285-309.
Levi N., Sandberg T. Blood transfusion and postoperative wound infection in intracapsular femoral neck fractures. Bull Hosp Jt Dis. 1998;57(2):69-73.
Lidder P.G., Sanders G., Whitehead E., et al. Pre-operative oral iron supplementation reduces blood transfusion in colorectal surgery: a prospective, randomised, controlled trial. Ann R Coll Surg Engl. 2007;89(4):418-421.
Lieberman J.A., Weiskopf R.B., Kelley S.D., et al. Critical oxygen delivery in conscious humans is less than 7.3 ml O2 • kg-1 • min-1. Anesthesiology. 2000;92(2):407-413.
Linderkamp O., Zilow E.P., Zilow G. [The critical hemoglobin value in newborn infants, infants, and children]. Beitr Infusionsther. 1992;30:235-246. German
Looker A.C., Dallman P.R., Carroll M.D., et al. Prevalence of iron deficiency in the United States. JAMA. 1997;277(12):973-976.
Mangano D.T., Tudor I.C., Dietzel C., Multicenter Study of Perioperative Ischemia Research Group; Ischemia Research and Education Foundation. The risk associated with aprotinin in cardiac surgery. N Engl J Med. 2006;354(4):353-365.
Masuda H., Moriyama Y., Hisatomi K., et al. Preoperative autologous donation of blood for a simple cardiac anomaly: analysis of children weighing under twenty kilograms. J Thorac Cardiovasc Surg. 2000;120(4):783-789.
Mathers N.P., James G.B., Walker J. The oxygen affinity of the blood of infants treated by intrauterine transfusion. J Obstet Gynaecol Br Common. 1970;77(7):648-653.
Mauermann W.J., Nemergut E.C. The anesthesiologist’s role in the prevention of surgical site infections. Anesthesiology. 2006;105(2):413-421. Erratum in: Anesthesiology. 2006;105(4):868.
McVay P.A., Toy P.T. Lack of increased bleeding after paracentesis and thoracentesis in patients with mild coagulation abnormalities. Transfusion. 1991;31(2):164-171.
Meliones J.N., Nichols D.G., Wetzel R.C., Greeley W.J. Perioperative management of patients with congenital heart disease: a multidisciplinary approach. In: Nichold D.G., Cameron D.E., Greeley W.J., Lappe D.G., Ungerleider R.M., Wetzel R.C., editors. Critical heart disease in infants and children. St. Louis: Mosby; 1995:553-579.
Moore P. Blood and justice: the seventeenth-century Parisian doctor who made blood transfusion history. Chichester, NJ: Wiley, 2003;223.
Moore W.M., Battaglia F.C., Hellegers A.E. Whole blood oxygen affinities of women with various hemoglobinopathies. Am J Obstet Gynecol. 1967;97(1):63-66.
Moran M.M., Kroon D., Tredwell S.J., Wadsworth L.D. The role of autologous blood transfusion in adolescents undergoing spinal surgery. Spine. 1995;20(5):532-536.
Mundy G.M., Birtwistle S.J., Power R.A. The effect of iron supplementation on the level of haemoglobin after lower limb arthroplasty. J Bone Joint Surg Br. 2005;87(2):213-217.
Murphy S., Gardner F.H. Effect of storage temperature on maintenance of platelet viability: deleterious effect of refrigerated storage. N Engl J Med. 1969;280(20):1094-1098.
Murray D.J., Forbes R.B., Titone M.B., Weinstein S.L. Transfusion management in pediatric and adolescent scoliosis surgery: efficacy of autologous blood. Spine. 1997;22(23):2735-2740.
Murray D.J., Pennell B.J., Weinstein S.L., Olson J.D. Packed red cells in acute blood loss: dilutional coagulopathy as a cause of surgical bleeding. Anesth Analg. 1995;80(2):336-342.
Muzi M., Ebert T.J. A comparison of baroreflex sensitivity during isoflurane and desflurane anesthesia in humans. Anesthesiology. 1995;82(4):919-925.
Napier J.A., Bruce M., Chapman J., British Committee for Standards in Haematology Blood Transfusion Task Force; Autologous Transfusion Working Party. Guidelines for autologous transfusion. II. Perioperative haemodilution and cell salvage. Br J Anaesth. 1997;78(6):768-771.
Natanson C., Kern S.J., Lurie P., et al. Cell-free hemoglobin-based blood substitutes and risk of myocardial infarction and death: a meta-analysis. JAMA. 2008;299(19):2304-2312. Epub 2008 Apr 28 Erratum in: JAMA. 2008;300(11):1300.
Nath A., Pogrel M.A. Preoperative autologous blood donation for oral and maxillofacial surgery: an analysis of 913 patients. J Oral Maxillofac Surg. 2005;63(3):347-349.
Nelson N.M., Prodhom L.S., Cherry R.B., Smith C.A. A further extension of the in vivo oxygen-dissociation curve for the blood of the newborn infant. J Clin Invest. 1964;43:606-610.
Ness P., Braine H., King K., et al. Single-donor platelets reduce the risk of septic platelet transfusion reactions. Transfusion. 2001;41(7):857-861.
Nishimura M., Uchida S., Mitsunaga S., et al. Characterization of T-cell clones derived from peripheral blood lymphocytes of a patient with transfusion-associated graft-versus-host disease: fas-mediated killing by CD4+ and CD8+ cytotoxic T-cell clones and tumor necrosis factor beta production by CD4+ T-cell clones. Blood. 1997;89(4):1440-1445.
Northern Neonatal Nursing Initiative Trial Group. Randomised trial of prophylactic early fresh-frozen plasma or gelatin or glucose in preterm babies: outcome at 2 years. Lancet. 1996;348(9022):229-232.
Ohlsson A., Aher S.M. Early erythropoietin for preventing red blood cell transfusion in preterm and/or low birth weight infants. Cochrane Database Syst Rev. 3, 2006. CD004863
Ohto H., Anderson K.C. Posttransfusion graft-versus-host disease in Japanese newborns. Transfusion. 1996;36(2):117-123.
Okuyama M., Ikeda K., Shibata T., et al. Preoperative iron supplementation and intraoperative transfusion during colorectal cancer surgery. Surg Today. 2005;35(1):36-40.
Opelz G., Sengar D.P., Mickey M.R., Terasaki P.I. Effect of blood transfusions on subsequent kidney transplants. Transplant Proc. 1973;5(1):253-259.
Oski F.A. The unique fetal red cell and its function: E. Mead Johnson Award address. Pediatrics. 1973;51(3):494-500.
Paridon S.M.. Consequence of chronic hypoxemia and pulmonary vascular disease. Garson J.T.Jr, Bricker A., Fisher D.J., Neish S.R., editors. The science and practice of pediatric cardiology, ed 2, II. Baltimore: Williams & Wilkins, 1998;2261-2272.
Parshuram C., Doyle J., Lau W., Shemie S.D. Transfusion-associated graft versus host disease. Pediatr Crit Care Med. 2002;3(1):57-62.
Popovsky M.A., Moore S.B. Diagnostic and pathogenetic considerations in transfusion-related acute lung injury. Transfusion. 1985;25(6):573-577.
Practice Guidelines for blood component therapy: a report by the American Society of Anesthesiologists Task Force on Blood Component Therapy. Anesthesiology. 1996;84(3):732-747.
Ramasethu J.L.N. Red blood cell transfusion in the newborn. Semin Neonatol. 1999;4:5-16.
Ray W.A., Stein C.M. The aprotinin story: is BART the final chapter? N Engl J Med. 2008;358(22):2398-2400. Epub 2008 May 14
Reilly C.W., McEwen J.A., Leveille L., et al. Minimizing tourniquet pressure in pediatric anterior cruciate ligament reconstructive surgery: a blinded, prospective randomized controlled trial. J Pediatr Orthop. 2009;29(3):275-280.
Rey R.M.Jr, Smoot E.C., Nguyen D., Lesavoy M.A. A study of the effects of epinephrine infiltration on delayed bleeding in a rat flap model. Ann Plast Surg. 1996;37(4):406-410.
Richa F., Yazigi A., Sleilaty G., Yazbeck P. Comparison between dexmedetomidine and remifentanil for controlled hypotension during tympanoplasty. Eur J Anaesthesiol. 2008;25(5):369-374. Epub 2008 Feb 25
Ririe D.G., Lantz P.E., Glazier S.S., Argenta L.C. Transfusion related acute lung injury in an infant during craniofacial surgery. Anesth Analg. 2005;101(4):1003.
Ronquist G., Waldenstrom A. Imbalance of plasma membrane ion leak and pump relationship as a new aetiological basis of certain disease states. J Intern Med. 2003;254(6):517-526.
Roseff S.D., Luban N.L., Manno C.S. Guidelines for assessing appropriateness of pediatric transfusion. Transfusion. 2002;42(11):1398-1413.
Sacher R.A., Luban N.L., Strauss R.G. Current practice and guidelines for the transfusion of cellular blood components in the newborn. Transfus Med Rev. 1989;3(1):39-54.
Schaller R.T.Jr, Schaller J., Morgan A., Furman E.B. Hemodilution anesthesia: a valuable aid to major cancer surgery in children. Am J Surg. 1983;146(1):79-84.
Schmidt P.J. Edinburgh and early transfusion in the New World. Vox Sang. 2004;87(Suppl 2):81-83.
Schved J.F. Preoperative autologous blood donation: a therapy that needs to be scientifically evaluated. Transfus Clin Biol. 2005;12(5):365-369.
Sethna N.F., Zurakowski D., Brustowicz R.M., et al. Tranexamic acid reduces intraoperative blood loss in pediatric patients undergoing scoliosis surgery. Anesthesiology. 2005;102(4):727-732.
Shannon K.M., Keith J.F., Mentzer W.C., et al. Recombinant human erythropoietin stimulates erythropoiesis and reduces erythrocyte transfusions in very low birth weight preterm infants. Pediatrics. 1995;95(1):1-8.
Shapiro F., Zurakowski D., Sethna N.F. Tranexamic acid diminishes intraoperative blood loss and transfusion in spinal fusions for duchenne muscular dystrophy scoliosis. Spine. 2007;32(20):2278-2283.
Shaz B.H., Dente C.J., Harris R.S., et al. Transfusion management of trauma patients. Anesth Analg. 2009;108(6):1760-1768.
Shear T., Tobias J.D. Cerebral oxygenation monitoring using near infrared spectroscopy during controlled hypotension. Paediatr Anaesth. 2005;15(6):504-508.
Silver M., Corwin M.J., Bazan A., et al. Efficacy of recombinant human erythropoietin in critically ill patients admitted to a long-term acute care facility: a randomized, double-blind, placebo-controlled trial. Crit Care Med. 2006;34(9):2310-2316.
Simon T.L., Alverson D.C., AuBuchon J., et al. Practice parameter for the use of red blood cell transfusions: developed by the Red Blood Cell Administration Practice Guideline Development Task Force of the College of American Pathologists. Arch Pathol Lab Med. 1998;122(2):130-138.
Smith H.M., Farrow S.J., Ackerman J.D., et al. Cardiac arrests associated with hyperkalemia during red blood cell transfusion: a case series. Anesth Analg. 2008;106(4):1062-1069.
Sonzogni V., Crupi G., Poma R., et al. Erythropoietin therapy and preoperative autologous blood donation in children undergoing open heart surgery. Br J Anaesth. 2001;87(3):429-434.
Stehling L., Luban N.L., Anderson K.C., et al. Guidelines for blood utilization review. Transfusion. 1994;34(5):438-448.
Stockman JA 3rd, Graeber J.E., Clark D.A., et al. Anemia of prematurity: determinants of the erythropoietin response. J Pediatr. 1984;105(5):786-792.
Stockman J.A.3rd. Anemia of prematurity: current concepts in the issue of when to transfuse. Pediatr Clin North Am. 1986;33(1):111-128.
Strauss R.G. Transfusion therapy in neonates. Am J Dis Child. 1991;145(8):904-911.
Sullivan M.T., McCullough J., Schreiber G.B., Wallace E.L. Blood collection and transfusion in the United States in 1997. Transfusion. 2002;42(10):1253-1260.
Sutton P.M., Cresswell T., Livesey J.P., et al. Treatment of anaemia after joint replacement: a double-blind, randomised, controlled trial of ferrous sulphate versus placebo. J Bone Joint Surg Br. 2004;86(1):31-33.
Tobias J.D., Berkenbosch J.W. Initial experience with dexmedetomidine in paediatric-aged patients. Paediatr Anaesth. 2002;12(2):171-175.
Tobias J.D. Controlled hypotension in children: a critical review of available agents. Paediatr Drugs. 2002;4(7):439-453.
Tortoriello T.A., Stayer S.A., Mott A.R., et al. A noninvasive estimation of mixed venous oxygen saturation using near-infrared spectroscopy by cerebral oximetry in pediatric cardiac surgery patients. Paediatr Anaesth. 2005;15(6):495-503.
Tremper K.K., Friedman A.E., Levine E.M., et al. The preoperative treatment of severely anemic patients with a perfluorochemical oxygen-transport fluid, Fluosol-DA. N Engl J Med. 1982;307(5):277-283.
Vamvakas E.C., Blajchman M.A. Deleterious clinical effects of transfusion-associated immunomodulation: fact or fiction? Blood. 2001;97(5):1180-1195.
Vaslef S.N., Knudsen N.W., Neligan P.J., Sebastian M.W. Massive transfusion exceeding 50 units of blood products in trauma patients. J Trauma. 2002;53(2):291-295.
Vega S.J., Nguyen T.V., Forsberg C., et al. Efficacy of preoperative autologous blood donation in free TRAM flap breast reconstruction. Plast Reconstr Surg. 2008;121(5):241e-246e.
Velardi F., Di Chirico A., Di Rocco C., et al. “No allogeneic blood transfusion” protocol for the surgical correction of craniosynostoses. II. Clinical application. Childs Nerv Syst. 1998;14(12):732-739.
Vichinsky E.P., Haberkern C.M., Neumayr L., The Preoperative Transfusion in Sickle Cell Disease Study Group. A comparison of conservative and aggressive transfusion regimens in the perioperative management of sickle cell disease. N Engl J Med. 1995;333(4):206-213.
Vogelsang G.B., Hess A.D. Graft-versus-host disease: new directions for a persistent problem. Blood. 1994;84(7):2061-2067.
Wahr J.A. Clinical potential of blood substitutes or oxygen therapeutics during cardiac surgery. Anesthesiol Clin North Am. 2003;21(3):553-568.
Wakankar H.M., Nicholl J.E., Koka R., D’Arcy J.C. The tourniquet in total knee arthroplasty: a prospective, randomised study. J Bone Joint Surg Br. 1999;81(1):30-33.
Waters J.H. Red blood cell recovery and reinfusion. Anesthesiol Clin North Am. 2005;23(2):283-294.
Weatherall M., Maling T.J. Oral iron therapy for anaemia after orthopaedic surgery: randomized clinical trial. ANZ J Surg. 2004;74(12):1049-1051.
Weldon B.C. Blood conservation in pediatric anesthesia. Anesthesiol Clin North Am. 2005;23(2):347-361.
Williamson K.R., Taswell H.F. Intraoperative blood salvage: a review. Transfusion. 1991;31(7):662-675.
Zauber N.P., Zauber A.G., Gordon F.J., Tillis A.C., Leeds H.C., Berman E., et al. Iron supplementation after femoral head replacement for patients with normal iron stores. JAMA. 1992;267(4):525-527.