CHAPTER 28 Biomechanics in refractive surgery
Introduction
The shape-subtraction paradigm of refractive keratectomy assumes that the cornea is biologically and biomechanically inert1. This assumption ignores a large body of experience with incisional refractive surgeries such as radial keratotomy, astigmatic keratotomy, and anterior lamellar keratomileusis. Biomechanics figure prominently in any surgery in which corneal tissue is removed or incised, including routine LASIK, and the effect is more pronounced in corneas altered by previous surgery. Surgeon nomograms to reduce systematic errors do little to prevent refractive errors attributable to patient-to-patient biological and biomechanical variations, particularly if we lack critical preoperative predictors and the means to measure them. This chapter surveys corneal biomechanical behavior and its role in improving the safety and predictability of refractive surgery.
Anatomic considerations
The cornea is a complex biomechanical composite whose behavior depends on its structural subcomponents and their organizational motifs (Fig. 28.1). Bowman’s layer and the stroma provide the majority of the cornea’s tensile strength. The low stiffness of Descemet’s membrane ensure its laxity over a broad range of intraocular pressures (IOP)2 and may serve to buffer the endothelium from stromal stresses. The role of Bowman’s layer, an 8–12 µm thick acellular condensation of stroma with more randomly oriented collagen fibrils3, has been a subject of controversy4,5. Although some have proposed a structural role distinct from that of the stroma, extensiometry studies in normal corneas suggest that removal of Bowman’s layer does not measurably alter the bulk mechanical properties of the cornea5. The biomechanical importance of Bowman’s layer in abnormally thin or ectatic corneas is suggested by its fragmentation during histological examination of keratoconic tissue.
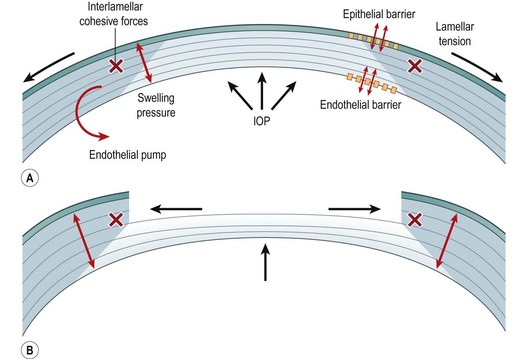
Fig. 28.1 Major biomechanical forces in the cornea (A) and a model of biomechanical central flattening associated with disruption of central lamellar segments (B). A reduction in lamellar tension in the peripheral stroma reduces resistance to swelling, and an acute expansion of peripheral stromal volume results10. Interlamellar cohesive forces11 and collagen interweaving12, indicated by gray shading, are greater in the anterior and peripheral stroma and provide a means of transmitting centripetal forces to underlying lamellae. Because the central portions of these lamellae constitute the immediate postoperative surface, flattening of the optical surface occurs, resulting in hyperopic shift. The degree of flattening is associated with the amount of peripheral thickening10. This phenomenon is exemplified clinically by PTK-induced hyperopic shift but is important in any central keratectomy, including PRK and LASIK. Simultaneous elastic weakening of the RSB may occur13, and the threshold for irreversible (plastic) deformation is a matter of great clinical concern.
The mechanical response of the cornea to injury is dominated by the stroma. On a weight basis, the stroma is approximately 78% water, 15% collagen, and 7% non-collagenous proteins, proteoglycans, and salts6. A total of 300–500 lamellae run from limbus to limbus and are stacked with angular offsets; this orientation becomes increasingly random in the anterior stroma where significantly more oblique branching and interweaving are noted3. Interlamellar branching is also more extensive in the corneal periphery (see Fig. 28.1)7,8. Interweaving of collagen bundles between neighboring lamellae provides a mechanism for shear resistance9 and sharing of tensile loads1,10. In addition, X-ray diffraction studies provide evidence of a predominantly circumferential fibril orientation in the corneal periphery11 that may favor conservation of limbal circumferential dimensions even in ectatic disease12,13. Proteoglycans play a critical role in collagen fibril assembly and spacing14, and their importance in promoting lamellar cohesion may be greater than currently recognized.
Fundamental principles
The elastic (Young’s) modulus provides an indicator of stiffness and is a critical material property in understanding the corneal response to refractive surgery. An elastic material regains its original geometry along the same stress–strain pathway when an imposed stress is removed. The elastic modulus is traditionally measured in excised tissue with an extensiometer that measures force generation during steady axial elongations of the sample. The slope of stress (force per unit area, N/m2) over strain (a dimensionless quantity defined by the current length divided by the starting length) is calculated for a representative portion of the curve. A high modulus indicates a stiff or low compliance material. While most biological soft tissues approximate linear elastic behavior over a small range of stresses, their overall elastic behavior is highly non-linear, as exemplified in Figure 28.2A. The reported range of ex vivo human cornea elastic modulus values spans orders of magnitude15. Although some biological variability is expected, this variability also reflects the challenges of obtaining representative data under highly variable experimental conditions ex vivo. Membrane inflation experiments in normohydrated donor globes provide a more physiological alternative to extensiometry16 but do not abrogate the need for in vivo measurement techniques.
Viscoelastic properties arise from the time-dependent nature of biomechanical responses and are a feature of all biological soft tissues. Viscoelastic materials return to their pre-stress configuration via different stress–strain pathways that depend on loading rates. This discordance between loading and unloading behavior can be partially characterized by hysteresis, which reflects the capacity of a tissue to dissipate energy. Viscoelastic creep and stress–relaxation (Fig. 28.2B) also describe time-dependent responses to sustained stress or strain that may be important in the mechanics of ectasia and post-refractive surgery instability17.
Preoperative assessment: clinical measurement of corneal biomechanical properties
Traditional extensiometry has revealed deficits in elastic tensile strength in keratoconus18 and suggests a diagnostic role for elastic modulus determination in the clinical setting. The obvious unsuitability of extensiometry for in vivo testing, however, has led to accelerated efforts to develop non-destructive, non-invasive tools for clinical biomechanical property measurement.
In sonic wave propagation velocimetry, an acoustic perturbation is emitted at one point on the ocular surface and detected by a receiver positioned at a fixed distance from the emitter. Young’s modulus is related to the corneal density and the wave velocity measured between the two transducer tips, that are positioned along the corneal arc. Stiffness is measured in the plane of the lamellae, similar to traditional extensiometry. Experiments with a prototype handheld device have explored directional and regional stiffness differences in porcine19 and human donor globes20, corneal stiffness changes with keratotomy, and increases in stiffness with stromal collagen cross-linking that lead to artifactually high applanation pressures19. Signal attenuation in the presence of the precorneal tear film is the primary challenge to clinical implementation.
The Ocular Response Analyzer (ORA, Reichert, Inc., Depew, NY) utilizes a high speed air-puff to quantify the dynamics of central corneal deformation and recovery21. Figure 28.3 illustrates a response waveform where the corneal hysteresis (CH) is the difference between the higher ingoing (P1) and lower outgoing (P2) applanation pressures. A high CH may indicate a greater capacity for absorption or dissipation of kinetic energy, which reflects viscoelastic behavior. The corneal resistance factor (CRF) is derived from the same dual-applanation signal with a linear factor emphasizing P1, the pressure required to achieve the first near-applanation endpoint, and thus reflects the overall corneal resistance. The ORA does not have regional or out-of-plane directional sensitivity, and the values are not directly comparable to the corneal elastic modulus. The ORA also reports two IOP values: Goldmann-correlated (IOPG) and cornea-compensated (IOPCC), where the latter was derived empirically to be less affected by LASIK than traditional applanation tonometry.
Though originally designed for IOP measurement, the ORA has supported a number of studies related to keratoconus and refractive surgery effects. Both CH and CRF are lower in eyes with keratoconus22,23, suggesting that both viscous damping capacity and overall corneal resistance are reduced in this disease, and the magnitude of this decrease is related to keratoconus severity22. However, the more challenging distinction between normal and forme fruste keratoconus (FFKC) is not aided significantly by CH or CRF24.
Other evolving techniques for measuring corneal biomechanical properties include measurements of bending resistance to stepwise indentations during Placido-ring topographic imaging25, interferometric determinations of apical displacement during IOP changes26, and three-dimensional corneal material property mapping with optical coherence elastography27. Because different combinations of perturbations and imaging modalities interrogate different aspects of the ocular biomechanical state, ongoing investigation will need to establish which measures are best suited for answering a particular clinical question.
Operative techniques: the biomechanical response to keratectomy
Several forces contribute to the preoperative steady state and undergo complex disruptions during corneal refractive surgery (see Fig. 28.1). The hydrophilia of glycosaminoglycans produces a negative intrastromal fluid pressure that effectively compresses the stroma28. The IOP manifests both as centripetal force and as a lamellar tension6 to counteract this stromal swelling pressure, which is also balanced by tear film evaporation, the epithelial and endothelial barriers, and the active endothelial transport29. Cohesive forces between lamellae provide further resistance to expansion of the interfibrillary space during swelling. Greater cohesive forces in the peripheral and superior cornea may have implications for the inferocentral predilection of keratoconus30 and in surgically induced astigmatism after ablation and flap creation.
During any procedure involving central ablation, corneal lamellae are immediately circumferentially severed. In a simple elastic shell model, this would result in a forward herniation with corneal steepening31. However, central ablation also relaxes lamellar tension in residual peripheral lamellar segments, which decreases local resistance to swelling and results in peripheral stromal thickening10. Expansion of the peripheral stroma’s dimensions and any limbal displacement owing to lamellar disruption may generate centripetal stress in underlying lamellae through the dense interlamellar connections at the margin of the ablation zone. Because the central portions of these lamellae comprise the new anterior surface, central flattening occurs (see Fig. 28.1). This flattening response appears to dominate any central steepening tendency with shallow ablations, but progressively deeper insults result in a net shift toward corneal steepening32,33. The elastic modulus of the residual stroma bed and the shear resistance in the bordering peripheral stroma may influence the rate of ablation-dependent flattening and the depth at which flattening effects lose ground to pre-ectatic elastic steepening. Outward limbus rotation with the relaxation of anterior lamellae may also contribute to central corneal flattening34.
This response is most clearly demonstrated by unintended hyperopic shift during PTK, in which an ablation depth-dependent flattening can be observed despite attempts at a uniform, plano ablation profile10,35. In a multivariate paired-control donor analysis of PTK-induced hyperopia, biomechanical peripheral thickening was more strongly associated with the degree of flattening than the measured ablation pattern itself10. While the relative influence of ablation profile is presumably greater in ametropic treatments than in PTK, this analysis demonstrated that changes in central curvature are not solely a product of ablative shape subtraction.
In clinical practice, an intrinsic flattening response augments the effects of a myopic procedure and impedes efforts to correct hyperopia. Thus, large over-corrections must be attempted to effectively treat high levels of primary hyperopia36. If an identical algorithm is used to treat secondary hyperopia of the same magnitude (i.e. after previous myopic LASIK), significant over-correction results. This difference in effectiveness can be attributed to the biomechanical status of a virgin cornea versus that of a surgically altered cornea that has adapted to an entirely different load-bearing milieu.
A comparison of surface ablation and LASIK
During any procedure involving central ablation, an immediate circumferential severing of corneal lamellae occurs and triggers the biophysical changes previously presented. CH and CRF have been shown to decrease after both LASIK and surface ablation21,23,37. LASIK clearly represents a more complex biomechanical insult than surface ablation, and its effects are additive to those described above. First, the total depth of the lamellar disruption is greater for a given refractive correction and will alter the balance between hyperopic effects and steepening effects from weakening of the corneal cap. Depth-dependent differences in corneal properties will affect this balance and likely vary from eye to eye and patient to patient. Creation of the LASIK flap even without subsequent photoablation induces hyperopia, astigmatism, and higher order aberrations that depend on hinge position and microkeratome type38,39. The meniscus-shaped flap produced by some mechanical microkeratomes interrupts more lamellae in the mid-peripheral bed than in its center, possibly potentiating the biomechanical response40 and confounding nomogram adjustments based on central flap thickness estimates. The optical effects of flap creation may arise not only from the biomechanically mediated shape changes in the residual stromal bed described above but also to thickness changes within the retained flap related to circumferential keratotomy41. To account for the separate optical effects of flap creation and photoablation, some have investigated a staged procedure incorporating a re-measurement delay after flap creation42,43. This approach is more strongly advocated for correction of ametropia and astigmatism after penetrating keratoplasty, where the biomechanical and wound healing responses to flap creation are far less predictable44,45.
Sub-Bowman’s keratomileusis (SBK) involves creation of a stromal–epithelial flap 90 µm in depth or thinner, often facilitated with a femtosecond laser, and could provide advantages over traditional LASIK by reducing the magnitude and variability of biomechanical responses associated with treatment depth. A recent study using the ORA has shown that PRK and SBK cause similar reductions in CH46. While these results have been used to suggest that thin-flap LASIK is no more destabilizing than surface ablation, it is important to remember that CH and CRF are not necessarily direct or highly sensitive measures of biomechanical stability. By circumventing the immediate and long-term biomechanical effects of flap creation on the cornea, surface ablation significantly reduces the number of variables that can affect the optical predictability of laser vision correction. But many of these advantages are offset by the greater impact of wound healing on the predictability of surface ablation procedures41, which is a subject beyond the scope of this chapter.
Postoperative complications
IOP measurement after refractive surgery
Friedenwald acknowledged the importance of corneal resistance in applanation tonometry in 193747. Many studies have demonstrated a decrease in applanation pressures after myopic PRK and LASIK that was initially attributed to decreases in CCT. Other studies demonstrating decreases in central applanation pressures after hyperopic LASIK without a decrease in CCT48 suggest that a decrease in corneal resistance to applanation does occur and can affect IOP measurement independently of the CCT. A sensitivity analysis of the various factors influencing applanation pressure suggests that the elastic modulus may be considerably more influential than corneal thickness or curvature49. In a pneumotonometry-based comparison of PRK and LASIK over 36 months, LASIK exhibited a greater long-term drop in apparent IOP than PRK in two populations with carefully matched refractive corrections, thus supporting the possibility of procedure-specific reduction in corneal resistance50. This issue and the important role of CCT in risk of progression from ocular hypertension to glaucoma51 have spawned efforts to decouple IOP measurement from confounding corneal biomechanical properties using devices such as the Ocular Response Analyzer and the Dynamic Contour Tonometer (DCT). The DCT provides a measure of IOP without applanation of the cornea, thus reducing the influence of corneal bending resistance and corneal thickness on the measurement52.
Ectasia
Debate continues about whether post-refractive surgery ectasia should be considered an iatrogenic condition or a subclinical predisposition to keratoconus that manifests after laser vision correction. Clinical risk factors for post-LASIK ectasia include high myopia, forme fruste keratoconus, and low RSB thickness53. While a lower limit of 250–300 µm has been recommended for the RSB thickness54, its actual value cannot be wholly determined preoperatively because it is affected by microkeratome predictability and a stromal ablation rate that varies with hydration and from patient to patient. More importantly, even the most accurate estimates of RSB thickness will not fully account for elastic and viscoelastic risk factors, just as presence of a normal central corneal thickness does not rule out keratoconus. Because focal weaknesses produce increases in local strain, spatial inhomogeneities in material strength may carry greater risk than low but spatially uniform properties. More uniform distributions of thickness and material strength may allow for long-term stability of some corneas with total thicknesses less than 250 µm55.
An apparent forward shift or steepening of the posterior corneal surface has been noted after LASIK and has been a source of concern to clinicians. Elastic bowing of the cornea may be difficult to distinguish from ectasia without the benefit of retrospect. Although the former can be a precursor of ectasia, ectasia involves a progressive deformation, by definition, and can therefore be described as a viscoelastic phenomenon or a progressive change in elastic properties that leads to optical failure and sometimes frank mechanical failure in the form of hydrops17. Immediate postoperative increases in central posterior corneal elevation often noted on scanning slit topography do not necessarily represent pre-ectasia, but may reflect a relative posterior movement of the peripheral stroma in response to the differential swelling described previously10,56. Artifactual posterior steepening can also result from minification of the central posterior radius of curvature after myopic photokeratectomy57.
LASIK may introduce a greater risk of viscoelastic failure than surface ablation procedures because of deeper forays into the posterior stroma. Lower keratocyte density, less collagen interweaving, and more hydrophilic proteoglycans may all contribute to a region more prone to viscoelastic failure and abnormal repair. Kerautret et al. published a case of unilateral corneal ectasia after bilateral LASIK demonstrating unexpectedly similar CH and CRF values in both eyes, but qualitative and quantitative differences in the ORA signals representing response abnormalities in the ectatic eye58. Further exploration of other features of the ORA signal has the potential to improve the sensitivity and specificity of the ORA for discriminating biomechanical abnormalities23,59.
Intrastromal ring segments and ultraviolet light/riboflavin-mediated corneal collagen cross-linking (CXL) are two biomechanical approaches to restoring structural stability in keratoconus and ectasia patients, and the latter has been shown in vitro to increase the elastic strength of the cornea60,19.
Assessment of surgery
Computational modeling in refractive surgery
Finite element analysis has been used in attempts to simulate surgical results in radial61 and astigmatic keratotomy62, phototherapeutic keratectomy31,63, PRK64, and LASIK34,64–66. Even with the most elegant models, however, assignment of appropriate material properties and boundary conditions is a great challenge and has a profound impact on the accuracy of surgical simulation and optimization34. Simulation-based surgical planning is within the scope of current computational capabilities but relies ultimately on the development of clinical methods for characterizing the complex corneo-scleral mechanics discussed above on a patient-to-patient basis.
1 Roberts C. The cornea is not a piece of plastic. J Refract Surg. 2000;16(4):407-413.
2 Jue B, Maurice DM. The mechanical properties of the rabbit and human cornea. J Biomech. 1986;19(10):847-853.
3 Komai Y, Ushiki T. The three-dimensional organization of collagen fibrils in the human cornea and sclera. Invest Ophthalmol Vis Sci. 1991;32(8):2244-2258.
4 Wilson SE, Hong JW. Bowman’s layer structure and function: critical or dispensable to corneal function? A hypothesis. Cornea. 2000;19(4):417-420.
5 Seiler T, Matallana M, Sendler S, et al. Does Bowman’s layer determine the biomechanical properties of the cornea? Refract Corneal Surg. 1992;8(2):139-142.
6 Maurice DM. The cornea and sclera, vol. 1b: Vegetative Physiology and Biochemistry. Davson H, editor. The eye. Orlando, FL: Academic Press, 1984.
7 Polack FM. Morphology of the cornea. I. Study with silver stains. Am J Ophthalmol. 1961;17:1051-1056.
8 Smolek MK, McCarey BE. Interlamellar adhesive strength in human eyebank corneas. Invest Ophthalmol Vis Sci. 1990;31(6):1087-1095.
9 Ehlers N. Studies on the hydration of the cornea with special reference to the acid hydration. Acta Ophthalmol (Copenh). 1966;44(6):924-931.
10 Dupps WJJr, Roberts C. Effect of acute biomechanical changes on corneal curvature after photokeratectomy. J Refract Surg. 2001;17(6):658-669.
11 Meek KM, Newton RH. Organization of collagen fibrils in the corneal stroma in relation to mechanical properties and surgical practice. J Refract Surg. 1999;15(6):695-699.
12 Edmund C. Corneal topography and elasticity in normal and keratoconic eyes. A methodological study concerning the pathogenesis of keratoconus. Acta Ophthalmol Suppl. 1989;193:1-36.
13 Smolek MK, Klyce SD. Is keratoconus a true ectasia? An evaluation of corneal surface area. Arch Ophthalmol. 2000;118(9):1179-1186.
14 Chakravarti S, Magnuson T, Lass JH, et al. Lumican regulates collagen fibril assembly: skin fragility and corneal opacity in the absence of lumican. J Cell Biol. 1998;141(5):1277-1286.
15 Bryant MR, McDonnell PJ. Constitutive laws for biomechanical modeling of refractive surgery. J Biomech Eng. 118(4), 1996. 473–441
16 Hjortdal JO, Jensen PK. In vitro measurement of corneal strain, thickness, and curvature using digital image processing. Acta Ophthalmol Scand. 1995;73(1):5-11.
17 Dupps WJJr. Biomechanical modeling of corneal ectasia. J Refract Surg. 2005;21(2):186-190.
18 Andreassen TT, Simonsen AH, Oxlund H. Biomechanical properties of keratoconus and normal corneas. Exp Eye Res. 1980;31(4):435-441.
19 Dupps WJ, Netto MV, Herekar S, et al. Surface wave elastometry of the cornea in porcine and human donor eyes. J Refract Surg. 2007;23(1):66-75.
20 Dupps WJ, Krueger RR, Jeng BH. Regional stiffness of human donor corneas measured by sonic wave elastometry. Invest Ophthalmol Vis Sci. 2006. (ARVO E-abstract 1335)
21 Luce DA. Determining in vivo biomechanical properties of the cornea with an ocular response analyzer. J Cataract Refract Surg. 2005;31(1):156-162.
22 Shah S, Laiquizzaman M, Bhojwani R, et al. Assessment of the biomechanical properties of the cornea with the Ocular Response Analyzer in normal and keratoconic eyes. Invest Ophthalmol Vis Sci. 2007;48:3026-3031.
23 Touboul D, Roberts C, Kérautret J, et al. Correlations between corneal hysteresis, intraocular pressure, and corneal central pachymetry. J Cataract Refract Surg. 2008;34:616-622.
24 Kirwan C, O’Malley D, O’Keefe M. Corneal hysteresis and corneal resistance factor in keratoectasia: findings using the Reichert Ocular Response Analyzer. Ophthalmologica. 2008;222:334-337.
25 Grabner G, Eilmsteiner R, Steindl C, et al. Dynamic corneal imaging. J Cataract Refract Surg. 2005;31(1):163-174.
26 Jaycock PD, Lobo L, Ibrahim J, et al. Interferometric technique to measure biomechanical changes in the cornea induced by refractive surgery. J Cataract Refract Surg. 2005;31(1):175-184.
27 Ford M, Dupps WJ, Huprikar N, et al. OCT elastography by pressure-induced optical feature flow. Proc SPIE. 2006. 6138OP (E-pub March 7, 2006)
28 Klyce SD, Dohlman CH, Tolpin DW. In vivo determination of corneal swelling pressure. Exp Eye Res. 1971;11(2):220-229.
29 Mishima S, Hedbys BO. Physiology of the cornea. Int Ophthalmol Clin. 1968;8(3):527-560.
30 Smolek MK. Interlamellar cohesive strength in the vertical meridian of human eye bank corneas. Invest Ophthalmol Vis Sci. 1954;34(10):2962-2969.
31 Bryant MRFD, Campos M, McDonnell PJ. Finite element analysis of corneal topographic changes after excimer laser phototherapeutic keratectomy. Invest Ophthalmol Vis Sci. 1993;31(Suppl):804.
32 Litwin KL, Moreira H, Ohadi C, et al. Changes in corneal curvature at different excimer laser ablative depths. Am J Ophthalmol. 1991;111(3):382-384.
33 Gilbert ML, Roth AS, Friedlander MH. Corneal flattening by shallow circular trephination in human eye bank eyes. Refract Corneal Surg. 1990;6(2):113-116.
34 Sinha Roy A, Dupps WJ. Effects of altered corneal stiffness on native and post-LASIK corneal biomechanical behavior: a whole-eye finite element analysis. J Refract Surg. 2009;25:875-887.
35 Fagerholm P, Fitzsimmons TD, Orndahl M, et al. Phototherapeutic keratectomy: long-term results in 166 eyes. Refract Corneal Surg. 1993;9(Suppl. 2):S76-S81.
36 Lindstrom RL, Hardten DR, Houtman DM, et al. Six-month results of hyperopic and astigmatic LASIK in eyes with primary and secondary hyperopia. Trans Am Ophthalmol Soc. 1999;97:241-255. discussion 55–60
37 Ortiz D, Piñero D, Shabayek MH, et al. Corneal biomechanical properties in normal, post-laser in situ keratomileusis, and keratoconic eyes. J Cataract Refract Surg. 2007;33:1371-1375.
38 Guell JL, Velasco F, Roberts C, et al. Corneal flap thickness and topography changes induced by flap creation during laser in situ keratomileusis. J Cataract Refract Surg. 2005;31(1):115-119.
39 Pallikaris IG, Kymionis GD, Panagopoulou SI, et al. Induced optical aberrations following formation of a laser in situ keratomileusis flap. J Cataract Refract Surg. 2002;28(10):1737-1741.
40 Krueger RR, Dupps WJ. Biomechanical effects of femtosecond and microkeratome-based flap creation: prospective, contralateral examination of 2 subjects. J Refract Surg. 2007;23:800-807.
41 Dupps WJ, Wilson SE. Biomechanics and wound healing in the cornea. Exp Eye Res. 2006;83(4):709-720. (Epub 20 May 2006)
42 Porter J, MacRae S, Yoon G, et al. Separate effects of the microkeratome incision and laser ablation on the eye’s wave aberration. Am J Ophthalmol. 2003;136(2):327-337.
43 Waheed S, Chalita MR, Xu M, et al. Flap-induced and laser-induced ocular aberrations in a two-step LASIK procedure. J Refract Surg. 2005;21(4):46-52.
44 Dada T, Vajpayee RB, Gupta V, et al. Microkeratome-induced reduction of astigmatism after penetrating keratoplasty. Am J Ophthalmol. 2001;131(4):507-508.
45 Mularoni A, Laffi GL, Bassein L, et al. Two-step LASIK with topography-guided ablation to correct astigmatism after penetrating keratoplasty. J Refract Surg. 2006;22(1):67-74.
46 Slade SG. Thin-flap laser-assisted in situ keratomileusis. Curr Opin Ophthalmol. 2008;86:215-218.
47 Friedenwald JS. Contribution to the theory and practice of tonometry. Am J Ophthalmol. 1937;20:985-1024.
48 Munger R, Dohadwala AA, Hodge WG, et al. Changes in measured intraocular pressure after hyperopic photorefractive keratectomy. J Cataract Refract Surg. 2001;27(8):1254-1262.
49 Liu J, Roberts CJ. Influence of corneal biomechanical properties on intraocular pressure measurement: quantitative analysis. J Cataract Refract Surg. 2005;31(1):146-155.
50 Pepose JS, Feigenbaum SK, Qazi MA, et al. Changes in corneal biomechanics and intraocular pressure following LASIK using static, dynamic, and contour tonometry. Am J Ophthalmol. 2007;143(1):39-47.
51 Brandt JD, Beiser JA, Kass MA, et al. Central corneal thickness in the Ocular Hypertension Treatment Study (OHTS). Ophthalmology. 2001;108(10):1779-1788.
52 Kaufmann C, Bachmann LM, Thiel MA. Intraocular pressure measurements using dynamic contour tonometry after laser in situ keratomileusis. Invest Ophthalmol Vis Sci. 2003;44(9):3790-3794.
53 Randleman JB, Russell B, Ward MA, et al. Risk factors and prognosis for corneal ectasia after LASIK. Ophthalmology. 2003;110(2):267-275.
54 Seiler T, Quurke AW. Iatrogenic keratectasia after LASIK in a case of forme fruste keratoconus. J Cataract Refract Surg. 1998;24(7):1007-1009.
55 Vinciguerra P, Munoz MI, Camesasca FI, et al. Long-term follow-up of ultrathin corneas after surface retreatment with phototherapeutic keratectomy. J Cataract Refract Surg. 2005;31(1):82-87.
56 Grzybowski DM, Roberts CJ, Mahmoud AM, et al. Model for nonectatic increase in posterior corneal elevation after ablative procedures. J Cataract Refract Surg. 2005;31(1):72-81.
57 Nawa Y, Masuda K, Ueda T, et al. Evaluation of apparent ectasia of the posterior surface of the cornea after keratorefractive surgery. J Cataract Refract Surg. 2005;31(3):571-573.
58 Kerautret J, Colin J, Touboul D, et al. Biomechanical characteristics of the ectatic cornea. J Cataract Refract Surg. 2008;34:510-513.
59 Hallahan KM, Sinha Roy A, Ambrosio RJr, et al. Evaluation of standard and derived ocular response analyzer (ORA) measures in keratoconus. Invest Ophthalmol Vis Sci. 2008. ARVO E-abstract
60 Wollensak G, Spoerl E, Seiler T. Stress-strain measurements of human and porcine corneas after riboflavin-ultraviolet-A-induced cross-linking. J Cataract Refract Surg. 2003;29:1780-1785.
61 Hanna KD, Jouve FE, Waring GO. Preliminary computer simulation of the effects of radial keratotomy. Arch Ophthalmol. 1989;107(6):911-918.
62 Hanna KD, Jouve FE, Waring GO, et al. Computer simulation of arcuate and radial incisions involving the corneoscleral limbus. Eye. 1989;3(Part 2):227-239.
63 Katsube N, Wang R, Okuma E, et al. Biomechanical response of the cornea to phototherapeutic keratectomy when treated as a fluid-filled porous material. J Refract Surg. 2002;18(5):S593-S597.
64 Alastrue V, Calvo B, Pena E, et al. Biomechanical modeling of refractive corneal surgery. J Biomech Eng. 2006;128(1):150-160.
65 Deenadayalu C, Mobasher B, Rajan SD, et al. Refractive change induced by the LASIK flap in a biomechanical finite element model. J Refract Surg. 2006;22(3):286-292.
66 Pandolfi A, Manganiello F. A model for the human cornea: constitutive formulation and numerical analysis. Biomech Model Mechanobiol. 2006;5:237-246.