CHAPTER 20 Anesthesia for Congenital Heart Surgery
This chapter describes the perioperative management of major forms of congenital heart disease (CHD) that require surgery with the use of cardiopulmonary bypass. Congenital cardiac lesions that are primarily addressed in the catheterization laboratory or without CPB are discussed in Chapter 21, Congenital Cardiac Anesthesia: Non-Bypass Procedures.
Congenital anomalies of the heart and cardiovascular system occur in 7 to 10 per 1000 live births. Congenital heart disease is the most common congenital disease, accounting for approximately 30% of all congenital diseases. CHD has become the principal cause of pediatric heart disease as the incidence of rheumatic heart disease has declined. Ten percent to 15% of children with CHD have associated congenital anomalies of the skeletal, genitourinary, or gastrointestinal system. The U.S. population of adults with CHD, surgically corrected or uncorrected, is estimated to exceed 1 million and is increasing steadily. As a result, it is not uncommon for adult patients with CHD to present for noncardiac surgery (see Chapter 21, Congenital Cardiac Anesthesia: Non-Bypass Procedures).
Congenital heart disease can be associated with specific complications. For example, infective endocarditis is a risk associated with most congenital cardiac anomalies. Sudden death occasionally occurs in patients who undergo surgical correction of CHD, presumably reflecting the effects of chronic abnormal hemodynamic loads, myocardial scarring and fibrosis, damage to the cardiac conduction system, or underlying (and presently occult) abnormal molecular and ion channel defects. Cardiac dysrhythmias are not usually a prominent presenting feature of CHD but can be more common as patients age and pathophysiologic sequelae of abnormal cardiac structure, function, and surgery accrue (see later and Chapter 21, Congenital Cardiac Anesthesia: Non-Bypass Procedures). Table 20-1 summarizes the pathophysiology and clinical picture associated with a wide variety of congenital heart defects. Table 20-2 summarizes the surgical repair options for each type of lesion.
TABLE 20-1 Pathophysiology and Clinical Picture of Congenital Heart Defects
Lesion Type | Pathophysiology | Clinical Signs and Symptoms |
Shunt Lesion without Outflow Tract Obstruction | ||
Shunt Lesions with Right Ventricular Outflow Tract Obstruction | ||
Transposition Physiology (Intercirculatory mixing) | ||
Single Ventricle Physiology | ||
One-Ventricle Lesions | ||
Two-Ventricle Lesions | ||
Left Ventricular Obstructive Lesions | ||
Mitral Stenosis | ||
Aortic Stenosis | ||
Coarctation | ||
Mixing of Systemic and Pulmonary Venous Blood with Series Circulation | ||
CHF
• Systemic and pulmonary vascular congestion; pulmonary vascular congestion is severe if pulmonary venous obstruction
PAPVR TAPVR |
CHF, Congestive heart failure; PAPVR, partial anomalous pulmonary venous return; TAPVR, total anomalous pulmonary venous return.
TABLE 20-2 Classification of Congenital Heart Lesions and Associated Repairs
Lesion Type | Repair |
Shunt Lesions | |
Left-to-Right | |
Complete repair | |
Complete repair | |
Complete repair | |
Complete repair | |
Complete repair | |
Right-to-Left | |
Complete repair | |
Complete repair | |
Complete repair | |
No repair | |
Transposition Physiology | |
Complete repair | |
Single-Ventricle Physiology | |
One-Ventricle Lesions | |
Staging to Fontan | |
Staging to Fontan | |
Staging to Fontan | |
Two-Ventricle Lesions | |
Complete repair | |
Complete repair | |
Complete repair | |
Left Ventricular Obstructive Lesions | |
Mitral Stenosis | |
Complete repair | |
Aortic Stenosis | |
Complete repair | |
Coarctation | |
Repair with likely residual lesions | |
Mixing of Systemic and Pulmonary Venous Blood with Series Circulation | |
Complete repair |
Pathophysiology of congenital heart disease
Shunting
Total pulmonary blood flow () is the sum of effective pulmonary blood flow and recirculated pulmonary blood flow. Total systemic blood flow (
) is the sum of effective systemic blood flow and recirculated systemic blood flow. Total pulmonary blood flow and total systemic blood flow do not have to be equal. Therefore, it is best to think of recirculated flow (physiologic shunt flow) as the extra, noneffective flow superimposed on the nutritive effective blood flow. These concepts are illustrated in Figures 20-1 to 20-3.
Single-Ventricle Physiology
Table 20-3 lists a number of single-ventricle physiology lesions. All patients with single-ventricle physiology who have severe hypoplasia of one ventricle will ultimately undergo the staged surgeries that comprise the single-ventricle pathway and result in Fontan physiology (described later). Patients with single-ventricle physiology and two well-formed ventricles are usually able to undergo a two-ventricle repair. In some cases, the two-ventricle repair will be complete. In others, significant residual lesions (VSD, aortopulmonary collaterals) will remain. In patients with single-ventricle physiology, the arterial oxygen saturation (Sao2) is determined by the relative volumes and saturations of pulmonary venous and systemic venous blood flows that have mixed and reach the aorta (see Fig. 20-1).
Aortic Blood Flow from: | Pulmonary Artery Blood Flow from: | |
HLHS | PDA | RV |
Severe neonatal aortic stenosis | PDA | RV |
IAA | LV (proximal)PDA (distal) | RV |
PA with IVS | LV | PDA |
Tetralogy of Fallot with pulmonary atresia | LV | PDA, MAPCAs |
Tricuspid atresia, NRGA, with pulmonary atresia (type 1A) | LV | PDA, MAPCAs |
Tricuspid atresia, NRGA, with restrictive VSD and pulmonary stenosis (type 1B) | LV | LV thru VSD to RV |
Tricuspid atresia, NRGA, with non-restrictive VSD and no pulmonary stenosis (type 1C) | LV | LV thru VSD to RV |
Truncus arteriosus | LV and RV | Aorta |
DILV, NRGA | LV | LV thru BVF |
BVF, Bulboventricular foramen; DILV, double-inlet left ventricle; HLHS, hypoplastic left heart syndrome; IAA, interrupted aortic arch; LV, left ventricle; MAPCAs, multiple aortopulmonary collateral arteries; NRGA, normally related great arteries; PA with IVS, pulmonary atresia with intact ventricular septum; PDA, patent ductus arteriosus; RV, right ventricle; VSD, ventricular septal defect.
Intercirculatory Mixing
Intercirculatory mixing is the unique situation that exists in transposition of the great arteries (TGA) (see Fig. 20-2). In TGA, there are two parallel circulations because of the existence of atrioventricular concordance (right atrium to right ventricle [RA-RV], and left atrium to left ventricle [LA-LV]) and ventriculoarterial discordance (right atrium to aorta [RV-Ao], and left ventricle to pulmonary artery [LV-PA]). This produces a parallel rather than a normal series circulation. In this arrangement, parallel recirculation of pulmonary venous blood in the pulmonary circuit and systemic venous blood in the systemic circuit occurs. Therefore, the physiologic shunt or the percentage of venous blood from one system that recirculates in the arterial outflow of the same system is 100% for both circuits.
Thus, this lesion is incompatible with life unless there are one or more communications (atrial septal defect [ASD], patent foramen ovale [PFO], VSD, PDA) between the parallel circuits to allow intercirculatory mixing. In the presence of mixing, arterial saturation (Sao2) is determined by the relative volumes and saturations of the recirculated systemic and effective systemic venous blood flows reaching the aorta (see Fig. 20-3).
Fontan Physiology
Fontan physiology (see also later) is a series (i.e., “normal”) circulation in which one ventricle has sufficient diastolic, systolic, and atrioventricular valve function to support systemic circulation (Figs. 20-4 and 20-5). This ventricle must in turn be in unobstructed continuity with the aorta and pulmonary venous blood return; there must also be unobstructed delivery of systemic venous blood to the pulmonary circulation (total cavopulmonary continuity).
General Approach to Anesthetic Management
Preparation for Anesthesia
Sevoflurane, halothane, isoflurane, and fentanyl plus midazolam do not change the ratio of pulmonary-to-systemic blood flow () in children with atrial and ventricular septal defects when cautiously administered with 100% oxygen (Laird et al., 2002). Sevoflurane (1 minimum alveolar concentration [MAC]) and fentanyl plus midazolam have no significant effect on myocardial function in patients with a single ventricle (Ikemba et al., 2004). Halothane (1 and 1.5 MAC) depresses cardiac index and contractility more than comparable levels of sevoflurane, isoflurane, and fentanyl plus midazolam anesthesia (Rivenes et al., 2001). In addition, halothane anesthesia may result in more severe hypotension and emergent drug use than sevoflurane anesthesia in children with CHD (Russell et al., 2001).
Intravenous Induction
Many of these patients come to the operating room with functioning intravenous (IV) access. For those without it, effective premedication may facilitate IV placement and allow the attendant risks of mask induction in this population to be avoided. In some patients, oral midazolam (0.5 to 1.0 mg/kg) may suffice. Others have used oral combinations of meperidine (3 mg/kg) and pentobarbital (4 mg/kg) successfully in this group of patients (Nicolson et al., 1989). Ketamine (∼3 to 6 mg/kg) and midazolam (1 mg/kg) given orally in combination can be quite effective in terms of producing deep sedation and conditions favorable for IV placement and subsequent intravenous induction (Auden et al., 2000).
High-dosage synthetic narcotics in combination with pancuronium (0.1 mg/kg) are commonly used for intravenous induction in neonates and infants. The vagolytic and sympathomimetic effects of pancuronium counteract the vagotonic effect of synthetic opioids. In patients with a low aortic diastolic blood pressure and a high baseline heart rate, vecuronium (0.1 mg/kg) or cisatracurium (0.2 mg/kg) may be used without affecting heart rate. In older children with mild to moderately depressed systolic function, lower dosages of a synthetic opioid can be used in conjunction with etomidate (0.1 to 0.3 mg/kg) (Sarkar et al., 2005).
Ketamine (1 to 2 mg/kg) is a useful induction agent. For patients with both normal and elevated baseline pulmonary vascular resistance (PVR), ketamine causes minimal increases in pulmonary artery pressure as long as the airway and ventilation are supported (Morray et al., 1984). The tachycardia and increase in systemic vascular resistance (SVR) induced by ketamine may make it unfavorable for use in patients with systemic outflow tract obstructive lesions (Williams et al., 2007).
The myocardial depressive and vasodilatory effects of propofol and thiopental make them largely unsuitable as induction agents except in patients with simple shunt lesions in whom cardiovascular function is preserved (Williams et al., 1999b).
Specific lesions
Atrial Septal Defect
Atrial septal defect accounts for about one third of the congenital heart disease detected in adults, with the frequency in women two to three times that in men. See related video online at www.expertconsult.com.
Strictly speaking, an ASD is a communication between the left and right atrium resulting from a defect in the intraatrial septum, which consists of a central membranous portion and a thicker inferior and superior fatty limbus. The central membranous portion is formed by tissue of the septum primum, ultimately forming the fossa ovalis. This membrane lies posterior to the superior aspect of the fatty limbus.
ASDs are classified by their location (Fig. 20-6). There are four morphologic types: ostium secundum defects, ostium primum defects, inferior and superior sinus venosus defects, and coronary sinus (CS) defects. Although they are classified as ASDs, sinus venosus and CS defects are not truly defects in the intraatrial septum. Secundum ASDs account for 80% of all ASDs. Whereas a PFO results from incomplete fusion of an intact fossa ovalis membrane with the superior aspect of the fatty limbus, an ostium secundum ASD is the result of actual deficiencies in the membrane (septum primum) of the fossa ovalis. Isolated ostium primum ASDs, also known as partial atrioventricular canal defects, are discussed later (see Atrioventricular Canal Defects). The isolated ostium primum defect extends from the inferior intraatrial septum fatty limbus, to the crest of the intact ventricular septum.
Clinical Presentation
Some small ostium secundum ASDs can be primarily closed, whereas larger defects are patched, usually with pericardium. An alternative is nonoperative device closure of secundum ASDs in the cardiac catheterization laboratory (see Chapter 21, Congenital Cardiac Anesthesia: Non-Bypass Procedures). Primum ASDs generally require patch closure and suture closure of the anterior leaflet mitral cleft. Sinus venous defects without partially anomalous pulmonary venous return can be closed primarily with a patch. An alternative procedure is performed when the pulmonary vein or veins anomalously enter the SVC. The SVC is transected above the origin of the anomalous vein or veins, and the SVC orifice is directed across the defect into the LA with a pericardial patch. The distal end of the SVC is then anastomosed in an end-to-end fashion to the roof of the RA appendage, recreating SVC-to-RA continuity.
Management of Anesthesia
The goals of anesthetic management for patients with ASD before cardiopulmonary bypass (CPB) follow from the aforementioned principles and are outlined in Box 20-1. The goals for these patients after CPB are outlined in Box 20-2.
Box 20-1 Anesthesia Management Goals for ASD, VSD, AVC Defect, and PDA before Cardiopulmonary Bypass (CPB)
Box 20-2 Management Goals for ASD, VSD, and AVC Defect after Cardiopulmonary Bypass (CPB)
From Bizzarro M, Gross I: Inhaled nitric oxide for the postoperative management of pulmonary hypertension in infants and children with congenital heart disease, Cochrane Database Syst Rev (4):CD005055, 2005.
Ventricular Septal Defect
Ventricular septal defect is the most common congenital cardiac abnormality in infants and children. See related video online at www.expertconsult.com.
A large number of VSDs close spontaneously by the time a child reaches 2 years of age. VSDs can be classified by their location in the septum (Fig. 20-7).
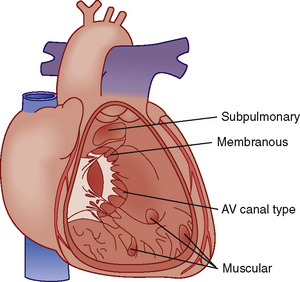
FIGURE 20-7 Locations of ventricular septal defects.
(Redrawn from Children’s Hospital Boston, Boston, Mass.)
Atrioventricular Canal Defects
Embryologically, four endocardial cushions contribute to the development of the lower ostium primum portion of the atrial septum and the upper, posterior inlet portion of the intact ventricular septum (IVS), where the AV valves insert. See related video online at www.expertconsult.com.
The endocardial cushions also contribute to the tissue that forms the septal leaflets of the mitral and tricuspid valves. Therefore, cushion defects, or atrioventricular canal (AVC) defects, can include abnormalities in all these structures. The terminology of these lesions can be confusing and is summarized as follows:
Tetralogy of Fallot
Tetralogy of Fallot is the most common cyanotic congenital heart defect. See related video online at www.expertconsult.com.
It is characterized by a VSD, an overriding aorta, a right ventricular hypertrophy, and pulmonic stenosis (infundibular or subvalvular, valvular, supravalvular, or a combination thereof) (Fig. 20-8). The critical pathophysiologic malformation is underdevelopment of the right ventricular infundibulum and displacement of the infundibular septum, which together result in right ventricular outflow tract (RVOT) stenosis. Patients with TOF have displacement of the infundibular septum in an anterior, superior, and leftward direction. The posterior wall of the right ventricular outflow tract is formed by the infundibular septum, and its abnormal displacement results in narrowing of the right ventricular outflow tract. In addition, displacement of the infundibular septum creates a large malalignment VSD, with the aorta overriding the intraventricular septum. Abnormalities in the septal and parietal attachments of the outflow tract further exacerbate the infundibular stenosis.
Hypoxic or Hypercyanotic Episodes (“Tet Spells”)
Treatment
Without surgery, mortality exceeds 50% by 3 years of age. Currently, most patients with TOF have an elective full correction between the ages of 2 and 10 months. In some centers, surgery is delayed as long as possible within this time interval, with the precise timing of repair dictated by the onset of cyanotic episodes. Definitive repair for TOF is being accomplished in neonates in some centers if favorable anatomy is present. Surgery is aimed at relieving the outflow obstruction by resection of hypertrophied, obstructing muscle bundles and augmentation and enlargement of the RVOT with a pericardial patch. In a somewhat older approach, unless the pulmonic annulus was near normal size, and the pulmonary valve only mildly stenotic, enlargement of the RVOT frequently included extension of the patch across the pulmonary valve annulus and into the main pulmonary artery (transannular patch). Because a transannular patch creates pulmonic insufficiency, which has been associated with a negative impact on long-term RV function and outcome, more recent data suggest it is best avoided when possible; as a result, the current approach for many is to enlarge the infundibular area with a patch (and resect interfering muscles bundles) and repair the pulmonary stenosis to the extent possible, thereby relieving most if not all of the outflow obstruction (and resultant RV hypertension) without creating significant pulmonary regurgitation. If stenosis of the pulmonary artery extends to the bifurcation of the pulmonary artery, a pericardial patch can be placed beyond the bifurcation of the pulmonary arteries (either de novo or as a continuation of the transannular patch). Finally, the VSD is closed. In neonates, this is usually done through the right ventriculotomy created for resection of RVOT obstruction, and with placement of the infundibular, pulmonary artery, or transannular patch. In infants and older children, the VSD can be closed via a trans–tricuspid valve approach, thereby avoiding the likely deleterious consequences of a right ventriculotomy. The overall goals of the surgery are to reduce RV pressure (ideally to below one half to three fourths of the systemic pressure), to avoid inducing RV volume overload (pulmonary regurgitation), and to successfully close the VSD (Apitz et al., 2009; Jonas, 2009; Khairy et al., 2009).
Management of Anesthesia
The anesthetic management goals for patients with tetralogy of Fallot are summarized in Box 20-3. Preoperatively, it is important to avoid dehydration by maintaining oral feedings in infants and young children or by providing intravenous fluids before the patient’s arrival in the operating room. Crying associated with intramuscular administration of drugs used for preoperative medication can lead to hypercyanotic spells in those prone to do so. β-Adrenergic antagonists (now used infrequently) should be continued until the induction of anesthesia in patients receiving these drugs, for prophylaxis against hypercyanotic attacks.
Box 20-3 Goals of Anesthesia Management for Tetralogy of Fallot
Ebstein’s Anomaly
Ebstein’s anomaly is an abnormality of the tricuspid valve in which the septal and often the posterior valve leaflets are displaced downward into the right ventricle (Fig. 20-9). See related video online at www.expertconsult.com.
In addition, the anterior tricuspid valve leaflet is abnormal. It is generally elongated and sail-like, with chordal attachments to the RV free wall. There may be RV outflow obstruction from the anterior leaflet. The result is a dilated right atrium with atrialization of the proximal RV and reduced effective RV cavity size and function. The tricuspid valve is usually regurgitant but may also be stenotic. Most patients with Ebstein’s anomaly have an interatrial communication (ASD, PFO), through which there may be right-to-left shunting of blood, the magnitude of which depends on the severity of the tricuspid valve abnormality and degree of RV dysfunction.
Treatment
Treatment of neonatal Ebstein’s anomaly is controversial and varies with the specific subtype of abnormality. Approaches range from tricuspid valve repair (for patients whose valve is amenable to repair and in whom the RV is likely to be able to support normal cardiac output), staging to a Fontan procedure (for those with significantly reduced RV size and function), and transplantation. In older patients, treatment is based on the prevention of associated complications, including antibiotic prophylaxis against infective endocarditis and administration of diuretics and digoxin for the management of CHF. Patients with supraventricular dysrhythmias are treated pharmacologically or with catheter ablation if an accessory pathway is present. Repair or replacement of the tricuspid valve in conjunction with closure of the interatrial communication is recommended for older patients who have severe symptoms despite medical therapy. Complications of surgery to correct Ebstein’s anomaly include third-degree atrioventricular heart block, persistence of supraventricular dysrhythmias, residual tricuspid regurgitation after valve repair, and prosthetic valve dysfunction when the tricuspid valve is replaced (da Silva et al., 2007; Knott-Craig et al., 2007; Bove et al., 2009).
Management of Anesthesia
Delayed onset of pharmacologic effects can be expected after IV administration of an anesthetic, which may result in part from pooling and dilution in an enlarged right atrium. The major hazards during anesthesia in patients with Ebstein’s anomaly include depression of RV function and reduced forward flow into the pulmonary artery, accentuation of arterial hypoxemia because of increases in the magnitude of the right-to-left intracardiac shunt, and the development of supraventricular tachydysrhythmias (Lerner et al., 2003). Increased right atrial pressures may indicate the presence of right ventricular failure. Both ventilatory and pharmacologic measures should focus on minimizing mechanical and metabolic effects of ventilation on RV afterload and on maintaining RV contractility. In the presence of a PFO, an increase in right atrial pressure above the pressure in the left atrium can lead to a right-to-left intracardiac shunt through the foramen ovale. Unexplained arterial hypoxemia or paradoxical air embolism during the perioperative period may result from shunting of blood or air through a previously closed foramen ovale.
Tricuspid Atresia
Tricuspid atresia (TA) involves a lesion with single-ventricle physiology. See related video online at www.expertconsult.com.
These patients are staged to a Fontan procedure. Patients with TA have complete obstruction to RV inflow and variable obstruction to RV outflow. A communication (ASD or PFO) results in an obligatory right-to-left shunt at the atrial level with complete mixing of systemic and pulmonary venous blood in the left atrium. When the ASD or PFO is restrictive, there is a large right atrial to left atrial pressure gradient, which results in poor decompression of the RA and systemic venous congestion. Pulmonary blood flow can be provided by a downstream shunt from intracardiac (VSD) and extracardiac (PDA, multiple aortopulmonary collateral arteries) sources. Anatomic classification is based on the presence or absence of transposition of the great arteries, the extent of pulmonary stenosis or atresia, and the size of the VSD. Approximately 70% of all patients with TA are type 1, and 50% percent of all TA patients are type 1B (Fig. 20-10, and see Fig. 20-2 and Table 20-3).
Treatment
Initial palliation depends on the anatomic variant. Infants with TA, adequate ASD, adequate VSD, and an “appropriate” amount of pulmonic stenosis can have a balanced circulation and require little or no intervention until later in infancy. Prostaglandin E1 (PGE1) is frequently necessary to augment PBF, and a balloon atrial septostomy may be necessary to allow adequate mixing and right atrial decompression. A Blalock-Taussig (BT) shunt may be required to provide a stable source of augmented pulmonary blood flow (e.g., TA with no VSD or with VSD but also with severe pulmonary stenosis or atresia). A staged approach to a Fontan procedure as described previously (see Figs. 20-4 and 20-5; Fig. 20-19) is the usual definitive treatment approach for TA.
Total Anomalous Pulmonary Venous Return
Anatomy
Total anomalous pulmonary venous return (TAPVR) results in blood return from all of the pulmonary veins into the systemic venous system rather than directly into the left atrium. See related video online at www.expertconsult.com.
The individual pulmonary veins usually drain into a common pulmonary venous confluence that has no direct connection to the LA; this confluence connects to the systemic venous circulation via one of four anatomic variants (Fig. 20-11):
Surgical Therapy
Definitive repair involves anastomosis of the pulmonary venous confluence to the posterior LA, ligation of the vertical vein, and closure of the ASD (Boger et al., 1999; Wang et al., 2004; Lacour-Gayet, 2006; Siles and Lapierre, 2008).
Post-CPB Management
Pulmonary hypertension (frequently at systemic or even suprasystemic levels of PA pressure) and limited systemic cardiac output are the major challenges after CPB. For patients’ reactive pulmonary vasculature, blunting of stress-induced increases in PVR with narcotics is indicated. In addition, ventilatory interventions that reduce PVR and the use of selective pulmonary vasodilators such as iNO are frequently necessary; indeed, this group of patients with CHD are among those most likely to respond to iNO after CPB with a clinically significant decrease in PVR and improvement in cardiac function (Adatia and Wessel, 1994; Curran et al., 1995; Bizzarro and Gross, 2005; Roberts et al., 1993).
Residual RV and PA hypertension can be caused by one or more of the following: (1) pulmonary venous obstruction, a technical (surgical) problem arising from the failure to construct a nonrestrictive anastomosis of the pulmonary venous confluence to the LA; (2) LA (and hence pulmonary venous) hypertension due to the small, noncompliant LA and LV and post-CPB LV dysfunction; and (3) labile increases in PVR due to reactive pulmonary vasoconstriction (capillary and precapillary). In the presence of postoperative pulmonary venous obstruction, efforts to increase pulmonary blood flow may worsen pulmonary edema. Surgical revision may be necessary (Lacour-Gayet, 2006).
Transposition of the Great Arteries
Anatomy
In patients with TGA, there is discordance of the ventriculoarterial connections and concordance of the atrioventricular connections. See related video online at www.expertconsult.com.
In other words, a right-sided RA connects via a right-sided tricuspid valve and RV to a right-sided and anterior aorta. A left-sided LA connects via a left-sided mitral valve and LV to a left-sided and posterior pulmonary artery (Fig. 20-12). The coronary arteries in dextro- (D-)TGA arise from the aortic sinuses that face the pulmonary artery. In normally related vessels, these sinuses are located on the anterior portion of the aorta, whereas in D-TGA they are located posteriorly. In the majority of D-TGA patients (70%), the right sinus is the origin of the right coronary artery, whereas the left sinus is the origin of the left main coronary artery. In the remainder of cases, there is considerable variability in this coronary anatomy. The exact coronary anatomy must be delineated; variants can contribute significantly to operative difficulty and the success of surgical repair.
Surgical Therapy
Mustard and Senning Procedures
Both the Mustard and the Senning procedures are atrial switch procedures, which surgically create discordant atrioventricular connections in the presence of the preexisting discordant ventriculoarterial connections. As a result of atrial baffling, systemic venous blood is directed to the LV, which remains in continuity with the pulmonary artery, and pulmonary venous blood is baffled to the RV, which is in continuity with the aorta. This arrangement results in physiologic but not anatomic correction of D-TGA. After these procedures, the right ventricle remains the systemic ventricle and the tricuspid valve remains the systemic AV valve (Fig. 20-13).
Both systemic and pulmonary venous obstruction by baffle material may occur as the result of either procedure. Because of the extensive atrial suture lines and at times atrial distention, dysrhythmias occur frequently after these procedures: about 60% to 70% of patients have some form of dysrhythmia, 30% of which are serious (bradycardia, sick-sinus syndrome, atrial flutter). Long-term exposure of the right ventricle and tricuspid valve to systemic pressure can result in progressive and severe right ventricular dysfunction (Horer et al., 2008).
Anatomic Repair or the Arterial (Jatene) Switch Procedure
The arterial switch operation (ASO) surgically reverses the discordant ventriculoarterial connections so that after repair, the right ventricle is connected to the pulmonary artery and the left ventricle is connected to the aorta. This is now the most common procedure performed for D-TGA (DeBord et al., 2007; Gottlieb et al., 2008; Quinn et al., 2008). In brief, the pulmonary artery and the aorta are transected distal to their respective valves. The coronary arteries are excised from the ascending aorta with 3 to 4 mm of surrounding tissue and these sites repaired either with pericardium or synthetic material. The coronary arteries are reimplanted into the proximal pulmonary artery (neoaorta), the distal pulmonary artery is brought anterior (LeCompte maneuver) and reanastomosed to the proximal aorta (right ventricular outflow), and the distal aorta is reanastomosed to the proximal pulmonary artery (left ventricular outflow) (Fig. 20-14). As mentioned earlier, variants in coronary anatomy can complicate this scenario. Although most patients with D-TGA have coronary anatomy that is suitable for coronary reimplantation, some variants, such as a single right coronary artery, are at risk for postoperative myocardial ischemia and death because reimplantation can result in distortion of the coronary ostia or narrowing of the artery itself. Inverted, intramural, or parallel coronary arteries can also be a problem.
Rastelli Procedure
The ASO is generally not performed on patients with significant anatomic and fixed LVOT obstruction (subpulmonic stenosis; patients with dynamic LVOT obstruction are likely to have no gradient across the LV outflow tract after the ASO). Correction of some types and severities of anatomic LVOT obstruction is possible and these patients are also potential candidates for ASO. For patients with D-TGA, VSD, and severe anatomic LVOT obstruction (subpulmonary stenosis), a Rastelli procedure is performed (Fig. 20-15).
Post-CPB Management
In some TGA patients, the LV may have limited ability to support the systemic circulation after the ASO. This may occur as the result of myocardial ischemia, inadequate LV mass, poor protection of the LV during aortic cross-clamping, or a combination thereof. Echocardiography can be useful in identifying both global and regional LV systolic dysfunction. It also detects mitral regurgitation, which may occur secondary to papillary muscle dysfunction or to dilation of the mitral valve annulus. Inotropic support of the LV and afterload reduction may be necessary to terminate CPB. Initial inotropic support is accomplished with dopamine (3 to 10 mcg/kg per minute). In rare instances when LV failure (that, again, has been proven not to be the result of a coronary problem) is severe, epinephrine (0.05 to 0.5 mcg/kg per minute) may have to be added. Milrinone (0.25 to 1.0 mcg/kg per minute after a 50-mcg/kg loading dose) can be a useful agent based on its inodilator properties (Hoffman et al., 2002, 2003). These patients are at particular risk for LV dysfunction in the immediate postoperative period secondary to afterload mismatch (insufficient contractility for the degree of systemic afterload). Although the vasodilation that accompanies milrinone administration in infants is substantially less than that seen in adult patients, it is nonetheless advisable to administer the loading dose of milrinone over 10 to 15 minutes or longer.
Truncus Arteriosus
Anatomy
A single great vessel arising from the base of the heart and giving rise to the pulmonary, coronary, and systemic arteries characterizes truncus arteriosus (Figs. 20-16 and 20-17). See related video online at www.expertconsult.com.
There is a single semilunar valve, which is usually abnormal, and invariably a large (nonrestrictive) conoventricular septal VSD is present. The truncus straddles this large VSD (see Fig. 20-16). Truncus arteriosus is classified based on the origin of the pulmonary arteries. In type 1, a short pulmonary trunk originates from the truncus and gives rise to both pulmonary arteries. In type 2, both pulmonary arteries arise from a common orifice of the truncus, whereas in type 3, the right and left pulmonary arteries arise separately from the lateral aspect of the truncus. The VSD is usually of the infundibular or perimembranous infundibular type. The truncal valve is tricuspid in most patients, but multiple cusps (four to seven) are common, and the valve itself may be regurgitant or stenotic. Extracardiac anomalies are seen in approximately 30% of patients.
Anesthetic Management
These patients are managed according to the principles described later (see Hypoplastic Left Heart Syndrome and Single-Ventricle Physiology). This is a non–ductal-dependent lesion.