Chapter 11 Anatomy of Nerve Root Compression, Nerve Root Tethering, and Spinal Instability
The majority of the population will experience spine-related pain at some time in their lives. The greatest component of this pain is low back pain, typically occurring in patients 35 to 55 years of age. Fortunately, the majority of acute back pain is self-limited, with over 90% of patients recovering within 6 weeks. Unfortunately, back pain has a high recurrence rate, with symptoms returning within the year in two thirds of patients. Sciatica-type pain is also common. The majority of sciatic pain is also self-limiting. Certain aspects of lifestyle, such as a lack of physical activity, obesity, and smoking, predispose patients to recurrent episodes of back pain and sciatica.1 Determining the precise causes of these types of pain presents a challenge to spine care physicians. Understanding the pathology of normal spinal degeneration will aid in the diagnosis and treatment of spine-related pain.
Understanding Motion Segments
The spine is composed of three anatomic sections, the cervical, lumbar, and thoracic spine. The majority of spine-related pain involves the lumbar spine because the lumbar spine bears the weight of the entire body. The lumbar spine is the primary focus of this chapter; however, the concepts described may be generalized to a great extent to the cervical and thoracic spine. As discussed in Chapter 5, vertebrae are linked through facet joints on the posterior spinal column. The facet joints are formed between the superior articular processes of one vertebra and the inferior articular processes of the vertebra directly above.
Causes of Back Pain
Subaxial spine pain is often caused by either muscular spasm or a failure of the joints and discs that comprise the complex anatomy of the spine. When examining the causes of isolated back pain in patients who present to a primary care physician, one study found that 4% had a compression fracture, 3% spondylolisthesis, 0.7% a tumor or metastasis of another tumor, 0.3% ankylosing spondylitis, and 0.01% an infection. Therefore, most patients who present with the complaint of low back pain will leave their primary care physician’s office without a definitive diagnosis.2,3 For most of these patients, some form of spinal degenerative change is the likely cause.
Spinal Degeneration
In spinal degeneration, also termed spondylosis, disc degeneration seems to occur first. Changes to the biologic structure of the disc lead to the mechanical failure of that disc. Normally, anulus cells synthesize mostly collagen type I in response to deformation, whereas nucleus cells respond to hydrostatic pressure by synthesizing proteoglycans and fine collagen type II fibrils. Cell density declines during growth and is extremely low in the adult, especially in the nucleus. In adult discs, blood vessels are normally restricted to the outermost layers of the anulus. Metabolite transport is by diffusion, which is important for small molecules, and by bulk fluid flow, which is important for large molecules. Low oxygen tension in the center of a disc leads to anaerobic metabolism, resulting in a high concentration of lactic acid and low pH.4 Chronic lack of oxygen causes nucleus cells to become quiescent, whereas a chronic lack of glucose can kill them. Deficiencies in metabolite transport appear to limit both the density and metabolic activity of disc cells. As a result, discs have only a limited ability to recover from any metabolic or mechanical injury.5 Disc cells synthesize their matrix and break down existing matrix by producing and activating degradative enzymes, including matrix metalloproteinases and “a disintegrin and metalloproteinase” (ADAMs). The proteoglycan content of the disc is primarily responsible for the disc’s ability to act as a compressive buffer. It is maximal in the young adult and declines later in life,6 presumably because of proteolysis. Disc cells appear to adapt the properties of their matrix to suit their environment. With increasing age, the overall proteoglycan and water content of the disc decreases, especially in the nucleus.6 There is a corresponding increase in collagen content, a tendency for fine type II collagen fibrils in the inner anulus to be replaced by type I fibers as the anulus encroaches on the nucleus, and a tendency for type I fibers throughout the disc to become coarser. Loss of proteoglycan fragments from the disc is a slow process owing to the entrapment of the nucleus by the fibrous anulus and the cartilage end plates of the vertebrae.7 Reduced matrix turnover in older discs enables collagen molecules and fibrils to become increasingly cross-linked with each other, and existing cross-links become more stable.5 In addition, reactions between collagen and glucose lead to so-called nonenzymatic glycation, causing even more cross-linking and imparting a yellow color to the aging disc. With increasing age, the hydrostatic nucleus becomes smaller and the proteoglycan content of the nucleus decreases. As such, its ability to hold water and withstand compressive loads declines. The anulus becomes stiffer and ultimately weaker.
Ultimately, aged discs fail to function properly and place additional strains on the facet joints and adjacent spinal motion segments. In the disc itself, the accumulated products of degeneration affect the metabolism of the remaining viable cells. This further hastens disc failure, resulting in changes that may be seen on MRI. These MRI changes include decreased water content, which is visible on T2-weighted images and is termed dark disc disease (Fig. 11-1). The end result of this cascade of failure is disc collapse.5
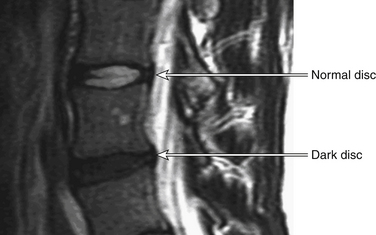
FIGURE 11-1 Sagittal MRI showing dark disc disease.
(From Lavelle WF, Carl AL, Lavelle ED, et al: Back pain. In Smith H, editor: Current therapy in pain, Philadelphia, 2009, Saunders Elsevier, pp 167–181.)
Isolated back pain may be due to a variety of forms of disc dysfunction. Pain may occur at any point of degeneration. Crock studied pain related to disc failure and coined the term internal disc derangement in 1970.8 The term was used to describe a large group of patients whose disabling back and leg pain worsened after an operation for suspected disc prolapse. Internal disc derangement was intended to describe a condition marked by alterations in the internal structure and metabolic functions of the disc thought to be attributable to injury or a series of injuries that may even have been subclinical.8 Despite Crock’s attempts to categorize disc failure, no direct and reliable relationship between measurable disc failure and pain has been developed.
As the disc fails, additional degenerative changes to the surrounding spinal structures may also occur. Disc failure is often the first of a series of failures in the spine. It has been hypothesized that disc failure causes the spinal ligaments to buckle and hypertrophy because of exposure to excessive forces, including new torsion forces.1,4 These abnormal forces may cause instability. Facet joint degenerative changes are believed to follow. When pain arises from the facet joints, patients often complain of greater discomfort with spine extension or hyperextension. Once muscles weaken, as is often seen with any form of spinal degeneration, any position can cause discomfort. As the degeneration progresses, further instability and joint hypertrophy may result. Similar to the degenerative changes seen in large appendicular joints such as the knee, significant radiographic degeneration may be seen in patients who have little or no back pain. These degenerative changes may, however, impinge on or stretch the neural elements, causing neuropathic pain.
Degenerative changes can also cause nerve root impingement in the neural foramen. The anteroposterior diameter of the foramen may be reduced by bulging disc material anteriorly and hypertrophic facets posteriorly. Foraminal height is reduced merely by the loss of intervertebral disc height. Facet subluxation can further decrease foraminal volume, making the exiting nerve roots in these patients even more susceptible to the compression caused be small amounts of disc bulging or facet hypertrophy.9
The areas of the degenerating spine may fail at different rates, leading to different clinical pictures of back pain, leg pain, or instability. If the anterior disc and ligaments fail at the same rate as the posterior structures, such as the facet joints, anterior subluxation of one vertebra is a possible result. This is termed spondylolisthesis. If failure occurs asymmetrically and there is a rotational or lateral translation, the deformity is termed olisthesis. Degenerative spondylolisthesis is most common at the L4-5 level5 and occurs 6 to 10 times more often here than at any other level. It is more common in women than men and in African Americans than whites.10 The increased motion caused by disc degeneration, combined with decreased shear resistance, allows for the anterior slip. Degenerative spondylolisthesis at the L4-5 level may result in a combination of central stenosis with lateral recess stenosis that compresses the traversing L5 nerve roots. Degenerative spondylolisthesis rarely exceeds 35% translation of the vertebrae.11
The posterior elements of the vertebra may also be disrupted by a stress fracture of an area of the spine called the pars interarticularis. The pars interarticularis is the lateral part of the posterior element that connects the superior and inferior facets (the term literally means “part between the articulations”). Repetitive flexion-extension and rotation lead to microtrauma at this junction and thereby fracture. Studies show that most patients with a spondylolysis or isthmic spondylolisthesis are unlikely to be at risk for increased back pain symptoms.12
Neuropathic Pain in Spinal Degeneration
The exact pathophysiology of the mechanisms of radicular and claudicant pain remains elusive. The sequences of neuropathologic changes that result from neurologic compression in the lumbar spinal canal have been investigated in animal studies. Delamarter et al.13 used a dog model in which they created varying degrees of stenosis and demonstrated deleterious effects on the neural elements by increasing the degree of the stenosis. They found that cortical evoked potentials were highly sensitive to this compression and were affected long before any clinical signs occurred. These authors also demonstrated venous congestion and arterial constriction around compressed nerve roots and dorsal root ganglia. The result was blockage of axoplasmic flow, with resulting edema, demyelination, and wallerian degeneration of motor and sensory fibers. Other authors have shown that sensory fibers are more susceptible to pressure and slower to recover than motor fibers,14 which may explain the presence of subjective sensory changes in the absence of objective physical findings. Arnoldi et al.15 suggested that increased venous pressure may explain the symptoms of neurogenic claudication. Others have suggested that narrowing of the spinal canal may lead to a reduction in blood supply to the cauda equina, resulting in ischemic changes from the diffusion of metabolites.16 These changes may stimulate the sinuvertebral nerve or lead to secretion of pain mediators, such as substance P, from the dorsal root ganglion. Perineural inflammation of unknown origin may also result in pain generation.
Most of the literature examining the causes of neurologic pain resulting from spinal pathology attributes compression as the principal cause.17–27 There are, however, instances where patients have persistent neuropathic pain, particularly radicular symptoms, in the absence of imaging studies displaying compressive pathology.
Motion of Neural Elements in the Spine: How Nerve Roots Can Be Stretched
Breig and Marions28 and Breig and Troup29 initially described movements of the nerve root sleeve in relation to a change in posture. They hypothesized that these patterns of movement might be related to changes in the length of the spinal canal during postural changes and motion.
To understand fully the impact of motion on neural elements, a basic understanding of the anatomic relationships of the nerve roots within the functional spinal unit (FSU) or motion segment is required. In the FSU, nerve roots are enclosed in a mobile osteoligamentous space and are exposed to dynamic stretch and compressive strains. This is most often observed in the situation in which nerve roots traverse a particularly long course through the central and lateral recess. Although compression is the mechanism most commonly associated with pain, inflammation as well as nerve root tethering are also possible causes.17,30 Tethering of the nerve root has been shown to be deleterious to nerves in clinical scenarios other than pathologic spinal degeneration, such as scoliosis, spina bifida occulta, and intrathecal spinal tumors.31 Stretch-induced nerve injury is also a well-known complication of lumbosacral spondylolisthesis reduction.32
As described earlier, lumbar nerve roots are enclosed in the lateral recess, a hollow, hemicylindrical recess that traverses mobile FSUs. The lateral recess is bordered laterally by the pedicle, posteriorly by the superior articular facet, and anteriorly by the dorsolateral surface of the vertebral body and the adjacent intervertebral disc (Fig. 11-2).
The unique and often underappreciated characteristic of this anatomic region is that lumbar nerve roots are dynamic neural structures with the ability to move alongside the deforming intervertebral disc and articulating adjacent facet joints. The lateral recess has been defined using a three-zone model,33,34 comprising the entrance zone, midzone, and exit zone. The entrance zone is located medial to and below the superior articular process, with the disc and facet joint forming the anterior and posterior walls, respectively. The midzone is the region through which the nerve root passes beneath the pars level of the lamina. Finally, the exit zone consists of the intervertebral foramen.
The lumbar nerve root may be compressed by or tethered to the surrounding structures primarily in two locations. The first is at the neck of the nerve root sheath as it exits the dural sac and the second is the lateral aspect of the foramen, where the nerve root is attached to both pedicles both rostrally and caudally by the foraminal ligaments (Fig. 11-3).
These ligaments limit nerve root excursion and increase in size and strength distally in the lumbar spine.35 The foraminal ligaments have been shown to play an organizational and protective function by equalizing stresses on neural structures during movements of the spine and extremities.35–38
Static lateral recess syndrome can be defined anatomically by the fixed, permanent entrapment of neural structures within the lateral recess.19,33,34 When a motion segment has erratic or excessive motion, such as that seen with instability associated with a spondylolisthesis, a dynamic lateral recess syndrome may occur. In the foramen, the exit zone of the lateral recess, the nerve root occupies 30% to 50% of the cross-sectional area. As such, there is ample room for the exiting nerve root. The dorsal root ganglion (DRG) is located just proximal to the origin of the spinal nerve. Its position relative to the foramen can vary considerably. The most common location of the DRG is directly beneath the foramen, except for the S1 DRG, which is usually located in the spinal canal.39 Therefore, if tethering occurs at two points close to the pedicle, nerve root stretch at the area of the DRG may occur. Also, because the DRG cells are the primary sensory neurons that send projections to peripheral and central targets, stretch deformation of the DRG may cause a variety of clinical responses, including pain.
It has also been shown that the more caudal lumbosacral nerve roots traverse a longer path to their extraspinal destination. Thus, the L4, L5, and S1 nerve roots traverse two or more mobile segments. Theoretically, this exposes them to a greater risk of either compression or stretch injury. This may partially explain the higher incidence of pain related to these nerve roots.19,33,34,37
Ventral Olisthesis and Loss of Disc Height
In a ventral olisthesis (translational/rotational deformity) of L4 on L5, the lateral recess of L4 moves forward, along with its neural contents. The L5 nerve root is subject to strain (change in length). The tethering effect of the foraminal ligaments combined with the anterior motion of the lateral recess causes the aforementioned strain. Further, the trefoil shape of the spinal canal is associated with reduced height of the lateral recess, which may increase the strain as well as compression seen at the L5 nerve root (Fig. 11-4).
Sagittal plane deformity such as focal kyphosis may also play a role in stretch of the lumbar nerve root. In a simulated ventral olisthesis of L4 on L5, a significant and deleterious differential strain is seen on the nerve root (Fig. 11-5). The maximum strain appears to occur at the L5 nerve root. Sagittal plane deformities are also known causes of axial back pain, requiring greater efforts by the paraspinal muscles to maintain an erect posture.
Lateral Olisthesis
Lateral olisthesis of L4 on L5 exerts a strain on the extraforaminal portion of the L4 root. This occurs because the entire lateral recess and its neural contents move with the olisthetic segment. Lateral olisthesis also stretches the subjacent L5 root on the side opposite to the olisthesis direction.27 The L5 pedicle on the ipsilateral side of the olisthesis acts as a fulcrum on the intraspinal portion of the subjacent part of the L5 nerve root. An investigation of degenerative scoliotic curves, in which lateral translation is associated with rotation, did not show that neural canal dimension was reduced with this particular deformity.39
Neural, Biomechanical, and Physiologic Considerations of Nerve Stretch
In the previous section we described the pathophysiology of nerve stretch and compression. To understand the development of axonal pathology in response to stretch, the relationship between the applied mechanical forces and the structural and functional response of the axon must be understood.27,40–46 The literature shows that strain rate is a time-dependent viscoelastic behavior that differs with variation in the histologic composition and diameter of the nerve root.46–49 The material properties of nerve roots are influenced by their relative proportions of protein and collagen. Spinal nerve roots contain approximately 20% of the amount of collagen in peripheral nerves and six times more collagen than the spinal cord.43,50,51 Conversely, the DRG is a mechanically and physiologically delicate structure. It has been shown that nerve roots are much less resilient than peripheral nerves, with a strength of only 10% and a stiffness of 20% of those of peripheral nerves.43 This may suggest that stretch through nerve roots in part occurs through dural or epineural tissues. It is thus apparent that the relative “mechanical friability” of nerve roots can be explained by these variations in histology and collagen composition.
Although stretch-induced neural injury of both the central and peripheral nervous system is well described in the literature, little is known regarding the biomechanical-physiologic responses to stretch at the nerve root level in humans.17,25,52–54 Animal models have provided some indirect evidence for the mechanisms of clinically observed pain syndromes. Such studies, however, should be interpreted with caution because the majority of animal models do not precisely reproduce the extent of damage, the biologic milieu, and the time course of axonal injury seen in humans.20,25,36,43–49,55,56
The amount of quantitative data available on the mechanical properties of human spinal nerve roots exposed to the low strain rates that occur at unstable FSUs is limited. Kwan et al. reported that human spinal nerve roots had a tensile strength of 0.17 ± 0.59 MPa and an ultimate strain of 15.0% ± 3.5%, at a strain rate 0.17 mm/sec.36 Sunderland and Bradley reported the ranges of maximum tensile stress and load to be 3.9 to 29.4 MPa and 0.2 to 3.3 kg, respectively, in human S3 nerve roots stretched at a rate of 1.27 mm/sec.57
Nerve roots have characteristic viscoelastic material properties that are strain rate dependent and exhibit higher tensile stress at higher strain rates.47–49 In vivo studies of rat L5 dorsal nerve roots subjected to a predetermined strain range (<10%, 10% to 20%, >20%) at a specified displacement rate (0.01 mm/sec and 15 mm/sec) demonstrated a threshold rate of complete nerve conduction loss at strain increases of 16% and 9% for the quasistatic 0.01 mm/sec and dynamic 15 mm/sec strain rates, respectively.47–49 These studies suggest that the modulating effects of excessive loading events (magnitude, rate of application, and duration) on electrophysiologic and possible pain responses may determine the extent of injury.
Basic Science of Chronic Spinal Pain and Stretch-Induced Nerve Root Injury
The actual mechanism by which neural tissue injury causes or contributes to chronic pain syndromes remains speculative. It has previously been suggested that injuries adjacent to the DRG produce pathologic reactions that manifest in differing severities of symptoms and animal behavioral responses.18,20,26,30,32,34,44,55,58–63
Stretch injury to peripheral nerves has been shown to induce local and central changes at the DRG and dorsal horn levels.17,32,54–56 Nerve root injury, on the other hand, may produce more robust, centrally mediated responses than a peripheral nerve injury. It has been suggested that a partial dorsal rhizotomy may activate injury signals in the dorsal root that are primarily transmitted to the central terminals of the spinal cord.18,21,27,39,44,55,58,59 These injury signals are manifested by sensitization of specific nociceptors or a variety of dorsal horn neurons with a short duration of pain persistence.18,47–49
The DRG itself, with its central and peripheral components, may represent the primary focus of stretch deformation during the pathomechanical behavior of a failing FSU. The mechanical deformation of DRG cells is well known to induce alterations in membrane properties that manifest as ectopic discharges and increased excitability. These, in turn, trigger chronic changes in excitability or synaptic plasticity of dorsal horn neurons.20,30,34,40,58,60,61
Although biomechanical and electrophysiologic data suggest a possible role of stretch deformation in painful neural element injury, the precise physiologic mechanism of this remains unclear. Nerve root injury, however, is hypothesized to induce central nervous system sensitization through a mechanism that is modulated by synaptic, neuroimmune, and neuroinflammatory events.24,26,46,58,62 These events mimic synaptic plasticity and remodeling similar to that observed in learning and memory.26
Role of Nerve Root Vascularization and Perfusion
Vascular hypoperfusion is another proposed mechanism for the physiologic and structural changes of neural tissues in response to stretch. Ischemic changes occur in response to an elongation of 15%.49 Histologic changes are also observed between 4% and 50%. Conduction disturbances have been reported at degrees of elongation ranging from 6% to 100%.49
The DRG has an abundant intrinsic vascular supply. The volume of blood flow in the DRG is approximately twice that of the nerve root and is similar to that of the gray matter of the spinal cord. Although the effect of compression on the DRG and nerve root blood flow is well documented in the literature, little is known about the effects of quasistatic or dynamic stretch on the nerve root or DRG venous pressure dynamics, blood flow, or blood-neural barrier function.24
Arnoldi C.C., Brodsky A.E., Cauchoix J., et al. Lumbar spinal stenosis and nerve root entrapment syndromes: definition and classification. Clin Orthop Relat Res. 1976;115:4-5.
Bain A.C., Raghupathi R., Meaney D.F. Dynamic stretch correlates to both morphological abnormalities and electrophysiological impairment in a model of traumatic axonal injury. J Neurotrauma. 2001;18:499-511.
Chung R.S., Staal J.A., McCormack G.H., et al. Mild axonal stretch injury in vitro induces a progressive series of neurofilament alterations ultimately leading to delayed axotomy. J Neurotrauma. 2005;22:1081-1091.
Delamarter R.B., Bohlman H.H., Dodge L.D., et al. Experimental lumbar spinal stenosis: analysis of the cortical evoked potentials, microvasculature, and histopathology. J Bone Joint Surg [Am]. 1990;72:110-120.
Kawakami M., Weinstein J.N., Chatani K., et al. Experimental lumbar radiculopathy: behavioral and histologic changes in a model of radicular pain after spinal nerve root irritation with chromic gut ligatures in the rat. Spine (Phila Pa 1976). 1994;19:1795-1802.
Kitab S.A., Miele V.J., Lavelle W.F., et al. Pathoanatomic basis for stretch-induced lumbar nerve root injury with a review of the literature. Neurosurgery. 2009;65:161-168.
Wall E.J., Massie J.B., Kwan M.K., et al. Experimental stretch neuropathy: changes in nerve conduction under tension. J Bone Joint Surg [Br]. 1992;74:126-129.
1. Lavelle W.F., Carl A.L., Lavelle E.D., et al. Back pain. In: Smith H., editor. Current therapy in pain. Philadelphia: Saunders Elsevier; 2009:167-181.
2. Koes B.W., van Tulder M.W., Thomas S. Diagnosis and treatment of low back pain. BMJ. 2006;332:1430-1434.
3. Deyo R.A., Rainville J., Kent D.L. What can the history and physical examination tell us about low back pain? JAMA. 1992;268:760-765.
4. Wisneski R.J., Garfin S.R., Rothman R.H. Lumbar disc disease. In: Herkowitz H., Garfin S.R., Balderson R.A., et al, editors. The spine. ed 4. Philadelphia: WB Saunders; 1999:671-746.
5. Adams M.A., Roughley P.J. What is intervertebral disc degeneration, and what causes it? Spine (Phila Pa 1976). 31, 2006. 2151–1261
6. Antoniou J., Steffen T., Nelson F., et al. The human lumbar intervertebral disc: evidence for changes in the biosynthesis and denaturation of the extracellular matrix with growth, maturation, ageing, and degeneration. J Clin Invest. 1996;98:996-1003.
7. Buckwalter J.A. Aging and degeneration of the human intervertebral disc. Spine (Phila Pa. 1995;1976(20):1307-1314.
8. Fardon D.F., Milette P.C. Nomenclature and classification of lumbar disc pathology. Spine (Phila Pa 1976). 2001;26:461-462.
9. Hilibrand A.S., Rand N. Degenerative lumbar stenosis: diagnosis and management. J Am Acad Orthop Surg. 1999;7:239-249.
10. Rosenberg N.J. Degenerative spondylolisthesis: predisposing factors. J Bone Joint Surg [Am]. 1975;57:467-474.
11. Cauchoix J., Benoist M., Chassaing V. Degenerative spondylolisthesis. Clin Orthop Relat Res. 1976;115:122-129.
12. Beutler W.J., Fredrickson B.E., Murtland A., et al. The natural history of spondylolysis and spondylolisthesis: 45-year follow-up evaluation. Spine (Phila Pa 1976). 2003;28:1027-1035.
13. Delamarter R.B., Bohlman H.H., Dodge L.D., et al. Experimental lumbar spinal stenosis: analysis of the cortical evoked potentials, microvasculature, and histopathology. J Bone Joint Surg [Am]. 1990;72:110-120.
14. Garfin S.R., Rydevik B.L., Lipson S.J. Spinal stenosis: pathophysiology. In: Rothman R.H., Simeone F.A., editors. The spine. vol 1, ed 3. Philadelphia: WB Saunders; 1992:791-826.
15. Arnoldi C.C., Brodsky A.E., Cauchoix J., et al. Lumbar spinal stenosis and nerve root entrapment syndromes: definition and classification. Clin Orthop Relat Res. 1976;115:4-5.
16. Parke W.W., Watanabe R. The intrinsic vasculature of the lumbosacral spinal nerve roots. Spine (Phila Pa 1976). 1985;10:508-515.
17. DeLeo J.A., Winkelstein B.A. Physiology of chronic spinal pain syndromes: from animal models to biomechanics. Spine (Phila Pa 1976). 2002;27:2526-2537.
18. Hanai F., Matsui N., Hongo N. Changes in responses of wide dynamic range neurons in the spinal dorsal horn after dorsal root or dorsal root ganglion compression. Spine (Phila Pa 1976). 1996;21:1408-1414. discussion 1414–1405
19. Hasegawa T., An H.S., Haughton V.M., et al. Lumbar foraminal stenosis: critical heights of the intervertebral discs and foramina. A cryomicrotome study in cadavera. J Bone Joint Surg [Am]. 1995;77:32-38.
20. Hunt J.L., Winkelstein B.A., Rutkowski M.D., et al. Repeated injury to the lumbar nerve roots produces enhanced mechanical allodynia and persistent spinal neuroinflammation. Spine (Phila Pa 1976). 2001;26:2073-2079.
21. Kawakami M., Weinstein J.N., Chatani K., et al. Experimental lumbar radiculopathy: behavioral and histologic changes in a model of radicular pain after spinal nerve root irritation with chromic gut ligatures in the rat. Spine (Phila Pa 1976). 1994;19:1795-1802.
22. Kim K.W., Chung J.W., Park J.B., et al. The course of the nerve root in the neural foramen and its relationship with foraminal entrapment or impingement in adult patients with lumbar isthmic spondylolisthesis and radicular pain. J Spinal Disord Tech. 2004;17:220-225.
23. Kraan G.A., Delwel E.J., Hoogland P.V., et al. Extraforaminal ligament attachments of human lumbar nerves. Spine (Phila Pa 1976). 2005;30:601-605.
24. Louis R. Vertebroradicular and vertebromedullar dynamics. Anat Clin. 1981;3:1-11.
25. Maxwell W.L., Povlishock J.T., Graham D.L. A mechanistic analysis of nondisruptive axonal injury: a review. J Neurotrauma. 1997;14:419-440.
26. Rutkowski M.D., Winkelstein B.A., Hickey W.F., et al. Lumbar nerve root injury induces central nervous system neuroimmune activation and neuroinflammation in the rat: relationship to painful radiculopathy. Spine (Phila Pa 1976). 2002;27:1604-1613.
27. Tabo E., Jinks S.L., Eisele J.H.Jr., et al. Behavioral manifestations of neuropathic pain and mechanical allodynia, and changes in spinal dorsal horn neurons, following L4-L6 dorsal root constriction in rats. Pain. 1999;80:503-520.
28. Breig A., Marions O. Biomechanics of the lumbosacral nerve roots. Acta Radiol. 1963;1:1141-1160.
29. Breig A., Troup J.D. Biomechanical considerations in the straight-leg-raising test: cadaveric and clinical studies of the effects of medial hip rotation. Spine (Phila Pa 1976). 1979;4:242-250.
30. Kawakami M., Weinstein J.N., Spratt K.F., et al. Experimental lumbar radiculopathy: immunohistochemical and quantitative demonstrations of pain induced by lumbar nerve root irritation of the rat. Spine (Phila Pa 1976). 1994;19:1780-1794.
31. Elsberg C.A. Congenital abnormalities of the spine and spinal cord. In: Hoeber P.B., editor. Surgical disease of the spinal cord, membranes and nerve roots: symptoms, diagnosis, and treatment. New York: Harper and Brothers; 1941:163-184.
32. Obata K., Yamanaka H., Kobayashi K., et al. The effect of site and type of nerve injury on the expression of brain-derived neurotrophic factor in the dorsal root ganglion and on neuropathic pain behavior. Neuroscience. 2006;137:961-970.
33. Ciric I., Mikhael M.A., Tarkington J.A., et al. The lateral recess syndrome: a variant of spinal stenosis. J Neurosurg. 1980;53:433-443.
34. Liu C.N., Michaelis M., Amir R., et al. Spinal nerve injury enhances subthreshold membrane potential oscillations in DRG neurons: relation to neuropathic pain. J Neurophysiol. 2000;84:205-215.
35. Grimes P.F., Massie J.B., Garfin S.R. Anatomic and biomechanical analysis of the lower lumbar foraminal ligaments. Spine (Phila Pa 1976). 2000;25:2009-2014.
36. Kwan M.K., Rydevik B., Myers R.R. Biomechanical and histological assessment of human lumbosacral spinal nerve roots. Trans Orthop Res Soc. 1989;14:348.
37. Spencer D.L., Irwin G.S., Miller J.A. Anatomy and significance of fixation of the lumbosacral nerve roots in sciatica. Spine (Phila Pa 1976). 1983;8:672-679.
38. Wiltse L.L., Fonseca A.S., Amster J., et al. Relationship of the dura, Hofmann’s ligaments, Batson’s plexus, and a fibrovascular membrane lying on the posterior surface of the vertebral bodies and attaching to the deep layer of the posterior longitudinal ligament: an anatomical, radiologic, and clinical study. Spine (Phila Pa 1976). 1993;18:1030-1043.
39. Bain A.C., Raghupathi R., Meaney D.F. Dynamic stretch correlates to both morphological abnormalities and electrophysiological impairment in a model of traumatic axonal injury. J Neurotrauma. 2001;18:499-511.
40. Abdulla F.A., Smith P.A. Axotomy- and autotomy-induced changes in the excitability of rat dorsal root ganglion neurons. J Neurophysiol. 2001;85:630-643.
41. Hasue M., Kunogi J., Konno S., et al. Classification by position of dorsal root ganglia in the lumbosacral region. Spine (Phila Pa 1976). 1989;14:1261-1264.
42. Ploumis A., Transfeldt E.E., Gilbert T.J.Jr., et al. Degenerative lumbar scoliosis: radiographic correlation of lateral rotatory olisthesis with neural canal dimensions. Spine (Phila Pa 1976). 2006;31:2353-2358.
43. Beel J.A., Stodieck L.S., Luttges M.W. Structural properties of spinal nerve roots: biomechanics. Exp Neurol. 1986;91:30-40.
44. Chung R.S., Staal J.A., McCormack G.H., et al. Mild axonal stretch injury in vitro induces a progressive series of neurofilament alterations ultimately leading to delayed axotomy. J Neurotrauma. 2005;22:1081-1091.
45. Song X.J., Hu S.J., Greenquist K.W., et al. Mechanical and thermal hyperalgesia and ectopic neuronal discharge after chronic compression of dorsal root ganglia. J Neurophysiol. 1999;82:3347-3358.
46. Winkelstein B.A., Weinstein J.N., DeLeo J.A. The role of mechanical deformation in lumbar radiculopathy: an in vivo model. Spine (Phila Pa 1976). 2002;27:27-33.
47. Singh A., Lu Y., Chen C., Cavanaugh J.M. Mechanical properties of spinal nerve roots subjected to tension at different strain rates. J Biomech. 2006;39(9):1669-1676.
48. Singh A., Lu Y., Chen C., et al. A new model of traumatic axonal injury to determine the effects of strain and displacement rates. Stapp Car Crash J. 2006;50:601-623.
49. Smith D.H., Wolf J.A., Lusardi T.A., et al. High tolerance and delayed elastic response of cultured axons to dynamic stretch injury. J Neurosci. 1999;19:4263-4269.
50. Lee C.K., Rauschning W., Glenn W. Lateral lumbar spinal canal stenosis: classification, pathologic anatomy and surgical decompression. Spine (Phila Pa 1976). 1988;13:313-320.
51. Stodieck L.S., Beel J.A., Luttges M.W. Structural properties of spinal nerve roots: protein composition. Exp Neurol. 1986;91:41-51.
52. Gilbert K.K., Brismée J.M., Collins D.L., et al. 2006 Young Investigator Award Winner: Lumbosacral nerve root displacement and strain: part 2. A comparison of 2 straight leg raise conditions in unembalmed cadavers. Spine (Phila Pa 1976). 2007;32:1521-1525.
53. Henderson F.C., Geddes J.F., Vaccaro A.R., et al. Stretch-associated injury in cervical spondylotic myelopathy: new concept and review. Neurosurgery. 2005;56:1101-1113. discussion 1101–1113
54. Wall E.J., Massie J.B., Kwan M.K., et al. Experimental stretch neuropathy: changes in nerve conduction under tension. J Bone Joint Surg [Br]. 1992;74:126-129.
55. Matsuda H., Tsai C.L., Tseng C.Y., et al. Neurophysiologic changes after preganglionic and postganglionic nerve-root constriction: an experimental study in the rat. Spine (Phila Pa 1976). 2007;32:950-958.
56. Millesi H., Zoch G., Reihsner R. Mechanical properties of peripheral nerves. Clin Orthop Relat Res. 1995;314:76-83.
57. Sunderland S., Bradley K. Stress-strain phenomenon in human spinal nerve roots. Brain. 1961;84:120-124.
58. Hashizume H., DeLeo J.A., Colburn R.W., et al. Spinal glial activation and cytokine expression after lumbar root injury in the rat. Spine (Phila Pa 1976). 2000;25:1206-1217.
59. Jou I.M. The effects from lumbar nerve root transection in rats on spinal somatosensory and motor-evoked potentials. Spine (Phila Pa 1976). 2004;29:147-155.
60. Kirita T., Takebayashi T., Mizuno S., et al. Electrophysiologic changes in dorsal root ganglion neurons and behavioral changes in a lumbar radiculopathy model. Spine (Phila Pa 1976). 2007;32:E65-E72.
61. Nakamura S.I., Myers R.R. Injury to dorsal root ganglia alters innervation of spinal cord dorsal horn lamina involved in nociception. Spine (Phila Pa 1976). 2000;25:537-542.
62. Winkelstein B.A., Rutkowski M.D., Sweitzer S.M., et al. Nerve injury proximal or distal to the DRG induces similar spinal glial activation and selective cytokine expression but differential behavioral responses to pharmacologic treatment. J Comp Neurol. 2001;439:127-139.
63. Kitab S.A., Miele V.J., Lavelle W.F., et al. Pathoanatomic basis for stretch-induced lumbar nerve root injury with a review of the literature. Neurosurgery. 2009;65:161-168.