CHAPTER 72 Anatomy and Synaptic Connectivity of the Basal Ganglia
Our knowledge of the functional anatomy of the basal ganglia has increased greatly over the past decades as a result of tremendous developments in the field. The use of sensitive tracing methods combined with high-resolution immunocytochemical methods has revealed complex features of the microcircuitry and macrocircuitry of the basal ganglia. The results of these studies led us to reconsider various aspects of the pathophysiology of basal ganglia disorders and the potential role of the basal ganglia in motor and nonmotor functions. In-depth knowledge of the basal ganglia circuitry is a prerequisite for a deeper understanding of the neural systems and functional network changes that underlie the beneficial effects of surgical therapies for movement disorders. This chapter provides an overview of the functional anatomy of the primate basal ganglia with an emphasis on recent findings that led us to reconsider the complex functions of the basal ganglia in normal and diseased states. Because of space constraints, this review does not aim at covering the whole literature on basal ganglia anatomy. Readers are referred to previous comprehensive reviews and compendiums for a survey of the early literature and a more general overview of this field.1–41
Functional Circuitry of the Basal Ganglia
The basal ganglia structures comprise the caudate nucleus and putamen, which make up the dorsal striatum and the nucleus accumbens and olfactory tubercle that form the ventral striatum. In rodents, the dorsal striatum is made up of a single mass of gray matter called the caudate-putamen complex. Other basal ganglia nuclei include the pallidum, which in primates consists of two parts, the internal and external segments of the globus pallidus, commonly referred to as GPi and GPe, respectively. In rodents, the homologue of the GPi is the entopeduncular nucleus and that of the GPe is the globus pallidus. The complex made up of the putamen and globus pallidus is called the lenticular nucleus. The subthalamic nucleus (STN), a small almond-shaped nucleus located laterally just below the thalamus at the junction between the diencephalon and midbrain, is another key basal ganglia structure often referred to as the “pacemaker” or “driving force” of the basal ganglia.10,20,21,30,31 The fact that the STN is the prime target of surgical therapy for Parkinson’s disease (PD) heavily supports its critical role in regulating basal ganglia function in normal and pathologic conditions.42–52 Finally, another major component of the basal ganglia network is the substantia nigra, located at the basis of the mesencephalon. The substantia nigra is divided into two major subnuclei; the substantia nigra pars compacta (SNc) consists of dopaminergic neurons, whereas the substantia nigra pars reticulata (SNr) is made up of GABAergic projection neurons. Other neighboring cellular groups related to the dopaminergic SNc include the ventral tegmental area (VTA) along the midline and the more caudal retrorubral field (RRF) lying along the ventrolateral edge of the upper brainstem. The basic circuit of the basal ganglia involves information originating from the entire cortical mantle and thalamus sent to the striatum, known as the main entrance of the basal ganglia. Once this information has been processed, it is sent to frontal cortical regions or the brainstem via functionally segregated basal ganglia thalamocortical channels of information that flow through the GPi and SNr, commonly known as the basal ganglia output nuclei (Fig. 72-1).
The Striatum: An Entrance to the Basal Ganglia Circuitry
The striatum consists of dorsal and ventral components based on their respective location along the dorsoventral extent of the telencephalon. These striatal regions are functionally different and process segregated information from the cerebral cortex; the main cortical input to the dorsal striatum originates from associative and sensorimotor areas, whereas the ventral striatum is predominantly innervated by limbic cortical regions.1
The striatal neuropil is further compartmentalized into two distinct territories called the patch (or striosomes) and the extrastriosomal matrix. These two striatal compartments receive distinct afferent projections and display a significant degree of neurochemical heterogeneity.2,53 Despite a pretty clear understanding of the anatomic organization of these compartments, their functional significance long remained poorly understood. However, there is recent evidence that imbalanced activity between the patch and matrix compartments may underlie some aspects of repetitive motor behavior known as stereotypies.54–57 The preferential recruitment of patch (or striosome) neurons after chronic exposure to psychostimulants probably represents a neural end point of the transmission from action-outcome associative behavior to conditioned habitual responding.57 The differential regulation of the Ras/Rap/extracellular signal–related kinase (ERK) mitogen-activated protein (MAP) kinase signal transduction cascades between the striosomal and extrastriosomal matrix compartments predicts the severity of the motor side effects induced by chronic dopaminergic antiparkinsonian therapy,58 and preferential loss of striatal patch projection neurons occurs in X-linked progressive dystonia-parkinsonism.59,60 Differential changes in GABAA receptor subunit expression between the patch and matrix compartments may underlie the variation in clinical symptomatology related to changes in mood in patients with Huntington’s disease.61
Cellular Organization of the Striatum
The striatum is largely made up of the so-called GABAergic medium spiny projection neurons, which can be divided into two major phenotypes based on their peptide and relative dopamine receptor expression (see later). There are about 2.8 × 106 medium spiny neurons in the rat striatum, which accounts for 95% to 97% of the total striatal neuronal population.62 These neurons have a small to medium-sized cell body from which emerge smooth proximal dendrites that give rise to a profuse and heavily spiny distal dendritic tree. Two main subtypes of striatal projection neurons have been identified: the “direct pathway” neurons preferentially express D1 dopamine receptors, substance P, and dynorphin and project directly to the GPi and SNr, whereas “indirect pathway” neurons express preferentially D2 dopamine receptors and enkephalin and project mainly to the GPe. Although both neuronal populations look morphologically similar, a recent rodent study has indicated that the dendritic length of D1 projection neurons is significantly greater than that of D2 neurons, an anatomic feature correlated with increased excitability of D2 neurons in adult mice.63–66
The abundance and plasticity of dendritic spines are other important morphologic criteria that could contribute to the physiologic role of medium spiny neurons. On average, single striatal projection neurons of either pathway are covered by about 5000 spines distributed quite homogeneously at about 1 spine/µm of distal dendritic length across species.67–70 The fact that in PD there is severe loss of striatal spines suggests an important regulatory role of dopamine and glutamate in striatal spine plasticity in normal and pathologic conditions.67–70 Although recent data from transgenic mice indicate that the loss of striatal spines in dopamine-depleted animals is specific to D2-containing neurons of the indirect pathway,68 these findings could not be confirmed in 1-methyl-4-phenyl-1,2,3,6-tetrahydropyridine (MPTP)-treated monkeys and humans with parkinsons, both of whom display more homogeneous spine loss between direct and indirect pathway striatofugal neurons.33,70
Medium spiny neurons give rise to a rich plexus of intrinsic axonal arborization within the vicinity of the parent cell bodies and contact neighboring projection neurons.71 Although this local connectivity has long been considered the main substrate for lateral inhibition in the striatum,71 electrophysiologic data have demonstrated that these connections are sparse and distal and consequently are poorly influential on the activity of striatal projection neurons.72,73 However, these connections are specifically organized and unidirectional between pairs of D1– or D2-containing neurons or from D2– to D1-positive projection neurons,74 with connections from D1– to D2-positive neurons being rare. The strength of these intrinsic connections is significantly reduced in dopamine-depleted parkinsonian conditions.74
The striatum also comprises four main populations of interneurons that represent about 2% to 3% of the total striatal neuronal population in rats, whereas in monkeys they account for as much as 23% of all striatal neurons.15,16,75,76 The cholinergic interneurons, which probably correspond to the “tonically active” neurons physiologically identified in the rat and monkey striatum,76–79 display a significant degree of colocalization with calretinin in primates.80 These neurons receive strong synaptic input from GABAergic axon collaterals of substance P–containing striatofugal neurons in rats81 and play a key role in reward-related learning and motivated behavior.77,78,82–89 In rats, they are connected to one another through GABAergic interneurons, thus providing a mechanism for their widespread recurrent inhibition via nicotinic excitation.90 The cholinergic interneurons are key mediators of dopamine-dependent striatal plasticity and learning.24,91–95
The GABA/parvalbumin interneurons are known as the “fast-spiking interneurons.” They form axosomatic synapses on projection neurons, are electrotonically coupled through gap junctions, and control spike timing in projection neurons, thereby providing the substrate for fast-forward intrastriatal inhibition of projection neurons in response to cortical activation (but see the article by Berke96).15,16,76
The GABA/nitric oxide synthase/neuropeptide Y/somatostatin interneurons are categorized physiologically as “persistent and low-threshold spike” neurons.15,16,76 These cells induce large inhibitory currents in projection neurons and release nitric oxide to modulate plasticity at glutamatergic synapses.16 In addition, because somatostatin actions entrain projection neurons into the rhythms generated by some interneurons, they are capable of modifying the processing and output of the striatum.97
The medium-sized GABA/calretinin interneurons represent the largest population of striatal interneurons in humans,80 display physiologic characteristics similar to the persistent and low-threshold spike neurons, and exert powerful monosynaptic inhibition of medium spiny projection neurons.76
Extrinsic Connectivity of the Striatum
Corticostriatal Projections
The cerebral cortex is the main source of glutamatergic input to the striatum. The corticostriatal pathway originates from all cortical areas and displays a highly topographic pattern of distribution in the striatum that imposes functionally segregated maps on it (see Fig. 72-1).98 The anatomic organization of the corticostriatal system has been extensively studied in rats and monkeys and more recently in humans via functional imaging approaches.99–119 These findings led to a basic scheme of functional connectivity between the cerebral cortex and striatum. The somatosensory, motor, and premotor cortices innervate the postcommissural region of the putamen somatotopically in a band-like pattern; the associative cortical areas from the frontal, parietal, and temporal lobes project to the caudate nucleus and the precommissural putamen; and the limbic cortices, the amygdala, and the hippocampus terminate preferentially in the ventral striatum (see Fig. 72-1).1,98 Functionally related associative or sensorimotor cortical inputs from areas connected through corticocortical projections either overlap or remain segregated in the striatum.116–119
In rats, corticostriatal neurons are divided into two distinct subsets: the superficial “intratelencephalic neurons,” which project solely to the striatum and the cerebral cortex, preferentially target “direct pathway” neurons, whereas the deep “pyramidal tract” neurons, which send their main axonal projections to the brainstem and spinal cord with collaterals to the striatum, preferentially target “indirect pathway” neurons.120,121 However, the physiologic significance of these anatomic observations remains unclear because electrophysiologic data suggest that intratelencephalic neurons are the main source of functional excitatory input to both populations of striatal projection neurons.122 There is also significant controversy regarding the existence of pyramidal tract corticostriatal neurons in primates.123–125
The dendritic spines of medium spiny neurons and GABA/parvalbumin-containing (PV) interneurons are the main targets of corticostriatal afferents,126–127 thereby laying the foundation for feedforward inhibition of striatal projection neurons in response to corticostriatal input.15,16,126–128 The cortical drive of feedforward inhibition from GABA/PV interneurons contributes to the imbalance of activity between the two populations of striatal projection neurons in the rat model of PD.129 However, this concept has recently been challenged by electrophysiologic evidence of the lack of correlation between cortical information flowing to GABA/PV neurons versus projection neurons.96
Thalamostriatal Projections
Thalamostriatal Projections from the Caudal Intralaminar Nuclei
The intralaminar thalamic nuclei are a major source of excitatory afferents to the striatum. In primates, the centromedian (CM) and parafascicular (PF) nuclei give rise to projections that largely terminate in different functional regions of the striatum. The medial part of the CM nucleus projects to the sensorimotor postcommissural sensorimotor putamen, whereas the PF nucleus predominantly innervates the associative caudate nucleus and the limbic ventral striatum.14,37 The dorsolateral PF nucleus selectively projects to the precommissural putamen. CM/PF neurons send only sparse projections to the cerebral cortex.130 At the synaptic level, CM and PF input preferentially targets the dendrites of striatal projection neurons.131–133 CM neurons also innervate striatal interneurons immunoreactive for choline acetyltransferase, parvalbumin, and somatostatin, but not calretinin.134 In line with these electron microscopic data, projections from the CM/PF complex tightly regulate the electrophysiologic activity and release of neurotransmitters from cholinergic interneurons135–137 and are required for the sensory responses of the “tonically acive neurons “(probably cholinergic) that are acquired through sensorimotor learning in monkeys.138–140
Thalamostriatal Projections from Other Thalamic Nuclei
The CM/PF complex is not the only source of thalamostriatal projections. Albeit sparse, most thalamic nuclei also contribute to a topographically and functionally organized striatal innervation.14,37,141–145 However, the synaptology of striatal projections from the CM/PF nuclei is strikingly different from that of other thalamic nuclei; that is, CM/PF terminals form synapses predominantly with the dendritic shafts of medium spiny neurons and interneurons, whereas projections from other thalamic nuclei, including the rostral intralaminar, midline, and relay thalamic nuclei, almost exclusively target dendritic spines.14,37,144,145 The degree of axon collateralization to the striatum and cortex of projections from the CM/PF complex versus other thalamic nuclei is significantly different. In contrast to CM/PF projections, which are directed mainly toward the striatum with minimal innervation of the frontal cortical areas, the relay and rostral intralaminar nuclei project predominantly to the cerebral cortex with modest striatal innervation.14,37,131,133,144,146 In general, thalamostriatal projections from single CM/PF neurons are much more focused and give rise to a significantly larger number of terminals than do individual corticostriatal axons.37,125,130 The functional significance of such differences in the pattern of striatal innervation between CM/PF and cortical input remains poorly understood.
Vesicular Glutamate Transporters as Specific Markers of Thalamostriatal versus Corticostriatal Projections
The vesicular glutamate transporters 1 and 2 (vGluT1 and vGluT2) are selective markers of the corticostriatal and thalamostriatal glutamatergic terminals, respectively.14,37,145,147 More than 95% of vGluT1 terminals contact spine heads, whereas only 50% to 60% of vGluT2 terminals do so in monkeys, a pattern that does not change in parkinsonism.145 In rats, there is a significant difference in the microcircuitry of vGluT2 terminals between the patch and matrix striatal compartments such that most axodendritic vGluT2 synapses are found in the matrix, consistent with the idea that the CM/PF complex is the main source of this synaptic input.14,37,133
Functional Roles of the Thalamostriatal Systems
The role or roles of the thalamostriatal systems probably differ between projections that arise from the CM/PF nuclei and those arising from other thalamic nuclei. In nonhuman primates, CM/PF neurons most likely supply striatal neurons with information that has attentional value in that they act as detectors of behaviorally significant events occurring on the contralateral side.138,139 In line with these data, changes in CM/PF activity are induced in response to attention-demanding reaction time tasks in humans.148 Electrical stimulation of the CM nucleus induces complex excitatory and inhibitory electrophysiologic responses in striatal projection neurons and cholinergic interneurons.135–137 In contrast, stimulation of rostral intralaminar nuclei results in complex alterations in cognitive processing, most likely through regulation of cortical and striatal activity.149,150 The function of other thalamostriatal systems is unknown, but they probably act as a positive reinforcer of specific populations of striatal neurons involved in performing a selected cortically driven behavior.14,37,151
Centromedian/Parafascicular Degeneration in Parkinson’s Disease
Postmortem studies of patients’ brains affected with progressive supranuclear palsy, Huntington’s disease, or PD revealed as much as a 50% loss of cells in the CM/PF nuclei.152,153 In patients with PD, the parvalbumin neurons are affected mainly in the PF nucleus, whereas the nonparvalbumin/noncalbindin neurons are specifically targeted in the CM nucleus.153 Asymmetric changes in the shape of the thalamus between patients with PD and healthy controls were recently reported.154 It is not clear whether these thalamic pathologies are also induced in animal models of parkinsonism. In rodents, some studies showed significant loss of PF thalamostriatal neurons in 6-hydroxydopamine–treated rats and MPTP-treated mice, whereas others did not find any cell loss in the PF nucleus under similar conditions.14,37 In nonhuman primates, a significant reduction in the relative abundance of vGluT2-positive terminals forming axodendritic synapses in the putamen of MPTP-treated monkeys was reported, thus suggesting a possible loss of CM-striatal neurons in this animal model.145
The Mesostriatal Dopaminergic Systems
Three main groups of dopaminergic neurons are found in the ventral midbrain: the A8 (RRF), A9 (SNc), and A10 (VTA) regions. Each of these subnuclei consists of predominantly dopaminergic neurons among which are interspersed small populations of GABAergic interneurons, except in the VTA, which has a significant population of GABAergic projection neurons.9 Various neuropeptides, including neurotensin and cholecystokinin, have been identified in subsets of dopaminergic neurons in the medial SNc and VTA.155–158 Ventral tier SNc (SNc-v) neurons are more significantly enriched with dopamine transporter than other ventral midbrain dopaminergic neurons,159 which may account for the preferential vulnerability of SNc-v neurons in response to MPTP.160,161 In addition, the calcium-binding protein calbindin D28k (CB) is strongly expressed in neurons of the VTA and RRF, as well as in dorsal tier neurons of the SNc (SNc-d), but it is not found in SNc-v neurons.9,162 A neuroprotective role of CB in SNc-d and VTA neurons in PD has been suggested, whereas the absence of CB may contribute to the selective vulnerability of SNc-v neurons in parkinsonism.163–165 The pattern of nigrostriatal degeneration at both the striatal and nigral levels is, indeed, correlated with the expression level of CB. At the striatal level, the sensorimotor postcommissural putamen, the most sensitive striatal region to dopaminergic denervation in PD (see later), is devoid of CB-containing neurons.166 In the substantia nigra, the more sensitive SNc-v neurons express a low level of CB immunoreactivity, whereas the relatively spared SNc-d and VTA neurons are enriched in CB.159,167 Finally, SNc neurons targeted by a strong CB-containing innervation from the striatum are more resistant than nigral neurons in CB-poor pockets called nigrosomes.164 Together, these findings highlight the potential neuroprotective role of CB in the pathogenesis of PD.
Based on various tract-tracing studies in monkeys, the following pattern emerged for organization of the nigrostriatal dopaminergic system in primates: (1) the sensorimotor striatum (i.e., postcommissural putamen) receives its main dopaminergic innervation from neuronal columns in the SNc-v, (2) the limbic striatum (i.e., nucleus accumbens) is the main recipient of dopaminergic projections from VTA and SNc-d neurons, and (3) the associative striatum (i.e., caudate nucleus and precommissural putamen) is preferentially innervated by dopaminergic neurons in the densocellular part of the SNc-v.9,33 In rats, the pattern appears to be slightly different in that SNc-d neurons project predominantly to the dorsal striatum.168
Single-cell filling studies have identified two main types of nigrostriatal axons: thin, varicose and widespread fibers that arise from neurons in the SNc-d, VTA, and RRF and preferentially terminate in the matrix striatal compartment, as well as thick varicose fibers that arise from the SNc-v and terminate mostly in the patch striatal compartment.169 A recent study using a more sensitive viral tracing method challenged this concept of dual nigrostriatal fiber systems and instead suggested a much more extensive axonal arborization of individual SNc dopaminergic neurons that do not display any preferential innervation of the patch or matrix compartments.170
It is well established that the pattern of progressive dopaminergic cell loss in PD is not homogeneous but rather displays a complex topographic and regional organization. In PD patients and MPTP-treated monkeys, two main features of nigrostriatal denervation have been well characterized: (1) the dopaminergic projections to the sensorimotor striatum (postcommissural plus dorsolateral precommissural putamen) are affected before those to the associative (caudate nucleus) and limbic (nucleus accumbens) striatal regions,171,172 and (2) VTA projections to the ventral striatum display a much lower degree of degeneration than do other midbrain dopaminergic cell groups.173,174 A preferential dopaminergic denervation of patch over matrix has also been suggested in MPTP-treated monkeys, but these data remain controversial and may be dependent on the animal species used and the method of MPTP administration.165,175
Dopamine has long been known to be a critical neuromodulator of basal ganglia neuronal activity through both presynaptic and postsynaptic mechanisms. Five dopamine receptor subtypes are expressed in striatal projection neurons and interneurons, thus providing multiple targets through which dopamine can mediate its effects. The dopamine-mediated regulation of glutamatergic and cholinergic transmission is severely affected in parkinsonism, thereby contributing to the abnormal changes in basal ganglia network activity described in PD. The morphology and plasticity of dendritic spines are also tightly regulated by dopamine/glutamate interactions, and this provides a substrate for integration and processing of extrinsic information to the basal ganglia circuitry.6,9,33,70
Other important modulatory systems that are not discussed in this review include the serotoninergic system from the raphe nuclei and the noradrenergic ascending projections from the locus coeruleus. Although not as well studied as the dopaminergic system, there is evidence that these two neurotransmitters regulate physiologic activity in various basal ganglia nuclei and possibly contribute to midbrain dopaminergic neuron loss and the development of nonmotor deficits and motor side effects from long-term dopaminergic therapy in patients with PD.176–183
Intrastriatal Dopamine Neurons: A Potential Compensatory Mechanism in Parkinson’s Disease
Dopaminergic interneurons have been described in the striatum of dopamine-depleted rats and monkeys and in the caudate nucleus and putamen of humans with PD.184–188 These aspiny neurons are small and coexpress various markers of dopaminergic neurons—glutamic acid decarboxylase and, in a small subset, calretinin.184,187 They receive scarce synaptic input and show up preferentially in the precommissural putamen and caudate nucleus in MPTP-treated monkeys.187 Their density significantly increases after dopamine depletion and the administration of glial-derived neurotrophic factor in the striatum,189 thus suggesting a potential compensatory role for dopaminergic interneurons in PD.
Extrastriatal Dopaminergic Systems
Although the striatum is well recognized as the main target of midbrain dopaminergic neurons, there is significant evidence that extrastriatal dopamine acting directly at the level of the globus pallidus, STN, and SNr also plays an important role in regulating basal ganglia function in normal and pathologic conditions.9,33 In addition, the existence of a dopaminergic afferent system to the ventral motor thalamus has recently been suggested.189–192 Although the exact origin of this system remains controversial, it could play a critical role in regulating the activity of thalamocortical neurons in basal ganglia–receiving nuclei, thereby contributing to the fine-tuning of information flow through the basal ganglia–thalamocortical loops in normal and parkinsonian conditions. Because of space limitations, this topic cannot be discussed extensively in this chapter, but readers are referred to recent publications and reviews that discuss the anatomic and physiologic evidence for these projections.9,33
The Direct and Indirect Pathways of the Basal Ganglia
Although overly simplistic, the direct and indirect pathway model of the basal ganglia has driven the field of basic and clinical basal ganglia research for the past decades. Obviously, since its introduction, this model has been challenged, revised, and updated as a result of gain in new knowledge of the basal ganglia circuitry, but it remains the most reliable working model of normal and abnormal physiology of the basal ganglia. The “direct pathway” refers to the monosynaptic connection between the striatum and the basal ganglia output nuclei, the GPi and the SNr, whereas the “indirect pathway” refers to the polysynaptic pathway linking the striatum and GPi/SNr via the GPe and STN.193–195 GABAergic striatal neurons give rise to either of these pathways, but they can be segregated into two populations by their peptide content (substance P—direct; enkephalin—indirect) and their preferential expression of dopamine receptor subtypes (D1—direct; D2—indirect).195 A balance between the activity of the two pathways is essential for normal functioning of the basal ganglia. In PD, the loss of striatal dopamine results in increased activity of indirect striatofugal neurons and decreased output from direct striatofugal neurons. Because of the polarity of connections in the direct/indirect pathways, this results in increased GABAergic basal ganglia outflow to the thalamus, which in turn may reduce cortical excitability and decrease motor behavior (Fig. 72-2).
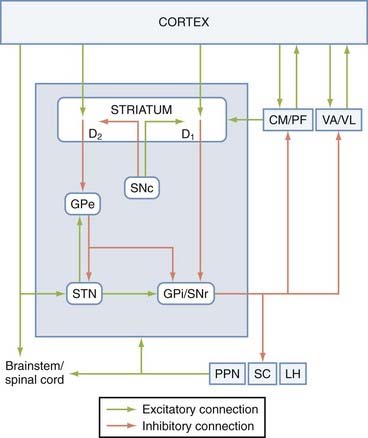
FIGURE 72-2 Direct and indirect pathway model of the basal ganglia. The blue box indicates tightly interconnected basal ganglia nuclei that receive extrinsic input from the cortical, thalamic, and brainstem regions. The extrastriatal substantia nigra pars compacta (SNc) dopaminergic projections to the globus pallidus pars externa (GPe), subthalamic nucleus (STN), and globus pallidus pars interna/substantia nigra pars reticulata (GPi/SNr) have been omitted from this diagram. The connections between the basal ganglia and the pedunculopontine nucleus/superior collicus/lateral habenula (PPN/SC/LH) are depicted in more detail in Figure 72-4. CM, centromedian nucleus of the thalamus; D1 and D2, dopamine D1-type and D2-type receptors; PF, parafascicular nucleus of the thalamus; VA/VL, ventral anterior and ventrolateral nuclei of the thalamus.
This traditional scheme of the basal ganglia circuitry has been challenged over the past decades because of some anatomic and molecular data suggesting that the two pathways may not be as segregated as previously thought. On the one hand, single-cell filling studies have demonstrated that most striatofugal neurons of the direct pathway give off collaterals to the GPe.8,196–198 Although the functional significance of these collateralized projections still remains poorly understood, they surely deserve consideration in the interpretation of functional changes in basal ganglia circuitry in normal and diseased states.
Another challenge to the model comes from molecular studies showing that D1 and D2 receptor mRNA may not be as segregated as originally thought.199–205 Significant controversy about dopamine receptor segregation was also raised in immunocytochemical studies; some reports have shown that D1 and D2 receptor protein immunoreactivity was largely segregated in two distinct populations of striatal spines,121,206 whereas others demonstrated a high level of D1 and D2 receptor immunoreactivity at the single-cell level in the rat striatum.207,208
More recently, bacterial artificial chromosome (BAC) transgenic mice have been developed.209,210 These BAC-D1 and BAC-D2 mice display complete segregation of D1 and D2 receptor mRNA, even when measured with the highly sensitive single-cell mRNA amplification method, an approach that revealed significant D1/D2 colocalization in normal rats.25,68,200,201,205 Whether this strict segregation of the two dopamine receptor subtypes underlies distinct functional dopamine-mediated physiologic effects or different mechanisms of synaptic plasticity between normal animals and these transgenic mice remains to be established. Another important fact to keep in mind while interpreting dopamine-mediated effects in individual striatofugal neurons is the possible coexpression of other D1 or D2 receptor family subtypes (i.e., D3, D4, and D5 receptors) in the two main populations of striatofugal neurons.211–216 To our knowledge, the relative expression level of D3, D4, and D5 receptors in striatofugal neurons of BAC-D1/enhanced green fluorescent protein (EGFP) or BAC-D2/EGFP transgenic mice remains unknown. We believe that such information is absolutely essential to clearly determine the chemical phenotype of striatofugal neurons in these animals and ensure that the functional data gathered from these mice can be translated to normal brains.
The Hyperdirect Corticosubthalamic System: Anatomic and Functional Significance
The STN is another major entry station for delivery of extrinsic cortical information to the basal ganglia circuitry. Information flowing along the corticosubthalamic tract is transmitted to the SNr and GPi at a faster pace than information transmitted along the direct and indirect corticostriatofugal systems.217 Basal ganglia output neurons do indeed respond with a fast excitation to electrical stimulation of the motor cortices. Excitotoxic STN lesions abolish these responses. In monkeys, the corticosubthalamic projection originates mainly from the motor cortices. Input from M1 flows along the dorsolateral sector of the nucleus, whereas projections from the supplementary motor area, premotor cortices, and the cingulate motor cortex overlap in the dorsomedial STN. There is a reversed somatotopy between the dorsolateral “M1 domain” and the dorsomedial “supplementary motor area/premotor cortices/cingulate motor cortex domain.”217–219 The ventrolateral half of the STN receives some input from the frontal and supplementary eye field areas, whereas the medial tip of the STN is involved in the processing of limbic-related information (Fig. 72-3). However, the exact sources and pattern of termination of cortical input to the ventral sectors of the STN have been less studied and remain poorly characterized.
An important role for the hyperdirect corticosubthalamic projection in the selection of motor programs has been proposed. According to this hypothesis, the cortical information flowing along the hyperdirect pathway is transmitted to a large and diffuse pool of GPi neurons in a nonspecific manner, thereby exciting a large population of basal ganglia output neurons not related to the selected motor act (i.e., the “surround neurons”). In contrast, the corollary signal transmitted along the corticostriatal system is much more focused and influences a restricted pool of GPi neurons (i.e., the “center neurons”). Via these projections, the cortical input flowing through the striatum and the STN generates a “center-surround” motor act selection model in the GPi that allows proper movements to be performed.217
Although this model generated significant interest, caution must be exercised in its interpretation in light of the anatomic and physiologic observations gathered from monkeys. First, the anatomic relationships between the STN and both pallidal segments are highly specific and topographic, with functionally related neurons in the GPe, GPi, and STN being connected,220,221 which is contrary to the assumption made by the model that the STN provides a diffuse projection to the GPi. Second, a majority of STN neurons increase their activity around the time of movement onset or after the movement during active step tracking movements in monkeys,222 thereby reducing the likelihood that the corticosubthalamic projection is involved in the preparation for movements as suggested by the center-surround hypothesis. However, recent reports have indicated that most STN neurons are active before self-paced movements in the parkinsonian human.223 Thus, the relative importance of the hyperdirect corticosubthalamic system in the basal ganglia circuitry remains a matter of debate that should be addressed in future studies.
Although the functional anatomy of the corticosubthalamic system largely relies on the processing of motor-related input from primary and secondary motor cortices, there is also evidence, gathered largely from rodent studies, that this system may be involved in processing limbic and cognitive information. The recent work of Baunez, Robbins, and colleagues led to the suggestion that the corticosubthalamic projection from the prefrontal cortex to the medial STN plays a role in preparatory processes, attention, perseveration, and other important cognitive or limbic functions in rats.224–233 However, the sources of nonmotor cortical afferents to the STN remain poorly characterized in nonhuman primates. In contrast, the recent use of diffusion-weighted magnetic resonance imaging methods has revealed connections between high-order associative areas of the frontal lobe and the STN in humans.234 It is also important to note that cognitive effects are sometimes induced by bilateral STN-DBS in PD patients.235–242 Although direct connections with the cerebral cortex remain to be established, it is worth noting that the ventromedial STN is tightly linked with the caudate nucleus and related associative regions of the GPe and GPi, thus providing another substrate for STN stimulation–mediated effects on complex cognitive functions.220,221,243,244 The caudal intralaminar nuclei and the brainstem pedunculopontine nucleus (PPN) are two additional sources of glutamatergic input to the STN that may contribute to the increased firing activity of STN neurons in parkinsonian conditions (see Fig. 72-3).221 In turn, the STN sends glutamatergic projections back to the cerebral cortex and PPN.221,243–245
The exact origin of corticosubthalamic neurons remains unknown. Although early data suggested that cortico-STN axons may be collaterals of the descending pyramidal tract in rats246 and cats,247 the recent filling of single pyramidal tract neurons in M1 led to the anterograde labeling of only a few scarcely distributed fibers in the monkey STN,125 thus suggesting that this projection may have a more complex origin than previously thought in primates.
The Pedunculopontine Nucleus as an Integrative Component of the Basal Ganglia
Cellular Organization and Connectivity of the Pedunclulopontine Nucleus
The PPN is made up of a chemically heterogeneous group of neurons in the upper brainstem that lies around the superior cerebellar peduncle. It is surrounded laterally by fibers of the medial lemniscus, medially by the decussation of the superior cerebellar peduncle, dorsally by the RRF, rostrally by the dorsomedial sector of the caudalmost tip of the substantia nigra, and caudally by the cuneiform nuclei. Two major neuronal groups have been identified: the PPN pars compacta (PPNc), which consists of densely packed cholinergic neurons in the caudolateral half of the nucleus, and the PPN pars diffusa (PPNd), which is more medially located and consists of sparsely distributed noncholinergic neurons along the dorsoventral extent of the superior cerebellar peduncle. In humans, the PPN comprises about 10,000 to 15,000 cholinergic neurons,248,249 which make up more than 90% of the PPNc.250 In nonhuman primates, the 40% of cholinergic neurons in the PPN express glutamate immunoreactivity.251 GABAergic, dopaminergic, noradrenergic, and various peptidergic neurons have also been identified within the boundaries of the PPN.252–257
It has long been known that the PPN is tightly linked with the basal ganglia. It receives major projections from the GPi and SNr (see later) and more modest input from the STN. Additional input to the PPN originates from the spinal cord, raphe nuclei, locus coeruleus, deep cerebellar nuclei, superior colliculus, and SNc.255–257 In turn, the PPN sends ascending projections to all basal ganglia nuclei, but most particularly to the SNc and the STN.258,259 Both glutamate and acetylcholine are used as neurotransmitters by these projections. In addition to feedback projections to the basal ganglia, the PPN is also an important source of descending projections to the pontine, medullary, and spinal structures. Through these connections, the PPN can thus be considered a possible relay station where information from the basal ganglia could bypass the thalamocortical loop and be transmitted directly to the reticular formation and spinal cord (see later).
The PPN also sends massive cholinergic and noncholinergic projections to the thalamus. This ascending PPN-thalamus system is critical for mediation of cortical desynchronization during waking and rapid eye movement sleep. Both cholinergic and glutamatergic PPN input to thalamostriatal neurons in the caudal intralaminar thalamic nuclei has been shown,260 thus suggesting that the PPN sends information to the basal ganglia not only directly but also indirectly via thalamostriatal neurons. Therefore, the PPN is strategically located to modulate neuronal activity in functional basal ganglia–thalamocortical and basal ganglia–thalamostriatal loops.253,257,260
Recent studies using diffusion tensor imaging have confirmed and extended our knowledge of PPN connectivity in humans. Despite the obvious limitations of this approach in differentiating afferent from efferent fiber pathways and the likelihood that small fiber tracts may not be detected with tractography, diffusion tensor imaging surely deserves strong interest because of its noninvasive nature and possible use in tracing neural connections in the human brain.261–264
The Pedunculopontine Nucleus as a Target for Functional Deep Brain Stimulation in Movement Disorders
There is experimental evidence that the PPN plays a role in the initiation and modulation of gait and other stereotyped movements in animals.255,265–267 Bilateral lesions of the PPN in normal monkeys induce bradykinesia.267–269 Postmortem studies have revealed as much as a 50% loss of cholinergic neurons in the PPN of parkinsonian patients.270,271 Blockade of GABAA receptors or low-frequency stimulation in the PPN reverses akinesia in MPTP-treated parkinsonian monkeys.272–274 This series of experimental data led to the hypothesis that DBS in the PPN area may be a suitable antiparkinsonian strategy for patients who suffer from gait freezing and poor balance, two late-onset symptoms of PD that are resistant to dopamine therapy.255,275–281 Although such procedures have been successfully applied to some patients,275–281 the exact brainstem site of stimulation remains controversial.282–283 Some authors have argued that the peripeduncular nucleus, a cell group located rostral and lateral to the PPN, has been the main target of DBS performed thus far in some PD patients.280–284
Basal Ganglia Output to the Thalamus and Brainstem
The GPi and SNr are the two main output stations of the basal ganglia. They integrate functionally segregated striatal input and send this information through massive GABAergic projections that profusely innervate thalamic, brainstem, and reticular targets. These projections, which often originate from collaterals of the same axons, are specific and functionally compartmentalized in their respective targets (Fig. 72-4). In the following account, the anatomic organization of GPi and SNr outflow is discussed, and the relevance of these projections in the transmission and processing of basal ganglia information through cortical and subcortical loops in normal and pathologic conditions is examined.
Efferent Projections of the Globus Pallidus Pars Interna
Two major types of projection neurons have been identified in the primate GPi: type I neurons send collateralized axonal projections to the thalamus and PPN, whereas type II neurons, located along the border of the GPi, project to the lateral habenula with rare collaterals to the anterior thalamic nuclei (see later).285 Each of these projection systems is discussed in more detail.
The Pallidothalamic Projection
The pallidothalamic projection travels via the ansa lenticularis and lenticular fasciculus to reach the ventral anterior/ventral lateral (VA/VL) nuclei,1,286,287 where it terminates in a topographic fashion. The exact origin of fibers that make up the two major pallidal outflow tracts remains controversial. On one hand, some studies have demonstrated that pallidothalamic fibers from the caudal sensorimotor portion of the GPi travel medially through the lenticular fasciculus to reach the thalamus whereas fibers coursing in the ansa lenticularis originate mostly from cells in the rostral GPi.288 This scheme is much simpler than that proposed in other studies in which it is suggested that fibers coursing through the ansa lenticularis frequently follow lengthy courses through the caudorostral extent of the GPi to reach the thalamus.285,289 This discrepancy may be the result of a significant degree of neuronal heterogeneity in the primate GPi.285 Such delineation is critical for effective surgical treatment of various movement disorders.288
Efferent projections from the sensorimotor GPi remain largely segregated from associative and limbic GPi outflow. In squirrel monkeys, the sensorimotor GPi output is directed toward the posterior VL (VLp) nucleus, whereas the associative and limbic GPi regions preferentially innervate the parvocellular VA (VApc) and the dorsal VL (VLd) nuclei. The ventromedial nucleus receives input from the limbic GPi only.98,286,287
The pallidal-, nigral-, and cerebellar-receiving territories are largely segregated in the primate thalamus, but they slightly overlap in rodents.290–292 In contrast to previous belief, pallidothalamic outflow to the cerebral cortex is not restricted to the supplementary and premotor cortices but also gains access to the primary motor cortex, as does information flowing along the cerebellothalamic tract.1,3–5,293–295 However, the sources of GPi or cerebellar projections to specific motor cortical areas are quantitatively different and largely segregated such that GPi outflow to thalamocortical neurons that innervate the supplementary and presupplementary motor areas is more massive than projections from the dentate nucleus to these areas.293 In the VL nucleus, the differential level of CB expression can be used as a landmark between GPi and cerebellar termination sites.286 About 10% to 20% of pallidothalamic neurons in the monkey GPi project to the contralateral VA/VL nuclei.296
Most pallidal neurons in the sensorimotor portion of the GPi that project to the VA/VL nuclei send axon collaterals to the CM nucleus (see Figs. 72-1 and 72-4), where they form synapses with thalamostriatal neurons projecting back to the sensorimotor territory of the striatum (i.e., the postcommissural putamen). Similarly, associative GPi outflow innervates the dorsolateral extension of the PF (PFdl) nucleus, which projects back to the precommissural associative region of the putamen, whereas the limbic GPi innervates the rostrodorsal PF nucleus, which preferentially innervates the nucleus accumbens. The CM/PF complex is therefore integrated into series of closed and open basal ganglia–thalamostriatal loops that run in parallel with the basal ganglia–thalamocortical system (see Fig. 72-1).14,37,297
The Pallidotegmental Projection
The main target of descending pallidotegmental fibers is the PPN. In monkeys, more than 80% of GPi neurons projecting to the PPN send axon collaterals to the VA/VL nuclei (Fig. 72-4). Because the PPN sends significant descending projections to lower brainstem regions and the spinal cord,253–256 the pallidotegmental projection may be a route by which cortical information processed in the basal ganglia can bypass the thalamocortical system to directly reach lower motor and autonomic centers. In contrast to the thalamus, where motor, associative, and limbic information is largely segregated, there is significantly more overlap between projections from different functional regions of the GPi in the PPN,298 thus suggesting a higher degree of functional convergence at the level of individual PPN neurons than in the thalamus. The medial pars diffusa of the PPN is the main site of termination of basal ganglia input from the GPi and SNr, which suggests that some of these PPN neurons may send projections back to the basal ganglia, thereby forming additional subcortical loops integrated within the basal ganglia circuitry.255,257,259,298
The Pallidohabenular Projection
As mentioned earlier, the pallidohabenular projection arises from a distinct population of neurons located along the borders of the GPi in nonhuman primates.1,285 Although originally seen as a rather minor projection, recent studies using sensitive tracing methods have demonstrated that the pallidohabenular projection is functionally organized and more massive than previously thought.284 In primates, the sensorimotor GPi preferentially innervates the centrolateral part of the lateral habenular nucleus, whereas the limbic and associative GPi regions project massively to the medial part of the nucleus (Smith and colleagues, unpublished data). Although the pallidohabenular projection is mainly GABAergic like other GPi output systems, cholinergic neurons also contribute to this projection in rats.299,300 In light of its close connections with various subcortical limbic regions, the lateral habenula is considered to be a functional interface between the limbic system and the basal ganglia.301–307
The lateral habenula is a major source of GABAergic inhibitory projections to midbrain dopaminergic neurons,301,308 although the anatomic and functional organization of this system has been studied mainly in rodents and remains to be carefully examined in primates (see Fig. 72-4). Recent studies have suggested that the pallidohabenular neurons convey reward-related signals to the lateral habenula, which then influences the striatum and other basal ganglia nuclei through regulation of the dopaminergic and serotoninergic systems (see Fig. 72-4).306 The lateral habenula also plays important roles in learning, memory, and attention.309,310 Because of its close relationships with the different monoaminergic systems, the lateral habenula is thought to be a potential target site for DBS in patients with severe depression.311
Efferent Projections of the Substantia Nigra Reticulata
The Nigrothalamic Projection
SNr and GPi input to the ventral thalamus is largely segregated from each other and from cerebellar afferents in primates.290 In monkeys, nigrothalamic cells form the largest population of nigrofugal neurons. Input from the medial part of the SNr terminates mostly in the medial magnocellular division of the VA (VAmc) and mediodorsal (MDmc) nuclei, which in turn innervate anterior regions of the frontal lobe, including the principal sulcus and the orbital cortex.1,11,312–317
Neurons in the lateral part of the SNr preferentially project to the lateral posterior region of the VAmc nucleus and to different parts of the MD nucleus, mostly related to posterior regions of the frontal lobe, including the frontal eye field areas of the premotor cortex (see Fig. 72-4). SNr outflow also gains access to thalamocortical neurons that project to the area TE in the inferotemporal cortex, thereby providing a substrate whereby the basal ganglia can influence higher order aspects of visual processing.316 Dysfunction in this system may therefore contribute to alterations in visual perception, including visual hallucinations in some basal ganglia disorders.
In rats, functionally segregated striatal neurons project to different lamellae of SNr neurons, which in turn send the information to different thalamic nuclei. The dendrites of individual SNr neurons largely conform to the geometry of striatonigral projections, which strongly supports the concept of a parallel architecture of striatonigral circuits.318–320 SNr neurons also project to the rostral and caudal intralaminar thalamic nuclei. In monkeys, SNr projections terminate in the PF nucleus, where they form GABAergic synapses with thalamostriatal neurons that project to the caudate nucleus.14,37,297
The Nigrotegmental Projection
The nigrotegmental projection has not been studied in detail in primates, but in rats it displays a dorsoventral topography and terminates preferentially on noncholinergic neurons in the medial two thirds of the PPNd.321,322 In monkeys, the cells that give rise to the nigrotegmental projection are found throughout the mediolateral extent of the SNr, and most send axon collaterals to the VA thalamic nucleus (see Fig. 72-4).1,312
The Nigrocollicular Projection
The nigrocollicular projection is massive and terminates in the intermediate layer of the superior colliculus, where nigral terminals form distinctive clusters that innervate neurons projecting to the spinal cord, medulla, and periabducens area (Fig. 72-4). This projection is critical for control of saccadic eye movements and orients the eyes toward a stimulus in response to auditory or visual stimuli.18,32,323–325 This is consistent with the fact that the SNr-receiving neurons of the intermediate layer of the superior colliculus are targeted by visual input from the cortex and project to brainstem regions that control eye movement. In turn, the superior colliculus sends a projection to dopaminergic neurons in the SNc in rats and primates.326–331 This projection is considered a prime source of sensory-related events to dopaminergic nigrostriatal neurons, thereby suggesting that the phasic responses of midbrain dopaminergic neurons in complex tasks may be related to “sensory prediction errors” instead of “reward prediction errors” (see Fig. 72-4).332–340
The Nigroreticular Projection
The SNr also sends projections to the medullary reticular formation. In rats, this projection arises from a population of neurons in the dorsolateral SNr and terminates in the parvicellular reticular formation. Identified nigroreticular neurons receive GABAergic input from the striatum and the globus pallidus. This projection probably plays a role in orofacial movements because the part of the SNr receiving neurons of the reticular formation is directly connected with orofacial motor nuclei.341–343
Benabid AL, Chabardes S, Mitrofanis J, et al. Deep brain stimulation of the subthalamic nucleus for the treatment of Parkinson’s disease. Lancet Neurol. 2009;8:67-81.
Fornai F, di Poggio AB, Pellegrini A, et al. Noradrenaline in Parkinson’s disease: from disease progression to current therapeutics. Curr Med Chem. 2007;14:2330-2334.
Graybiel AM. Habits, rituals, and the evaluative brain. Annu Rev Neurosci. 2008;31:359-387.
Haber SN, Calzavara R. The cortico-basal ganglia integrative network: the role of the thalamus. Brain Res Bull. 2009;78:69-74.
Kimura M, Minamimoto T, Matsumoto N, et al. Monitoring and switching of cortico-basal ganglia loop functions by the thalamo-striatal system. Neurosci Res. 2004;48:355-360.
Matsumoto M, Hikosaka O. Representation of negative motivational value in the primate lateral habenula. Nat Neurosci. 2009;12:77-84.
Matsumoto M, Hikosaka O. Lateral habenula as a source of negative reward signals in dopamine neurons. Nature. 2007;447:1111-1115.
May PJ, McHaffie JG, Stanford TR, et al. Tectonigral projections in the primate: a pathway for pre-attentive sensory input to midbrain dopaminergic neurons. Eur J Neurosci. 2009;29:575-587.
Mazloom M, Smith Y. Synaptic microcircuitry of tyrosine hydroxylase-containing neurons and terminals in the striatum of 1-methyl-4-phenyl-1,2,3,6-tetrahydropyridine–treated monkeys. J Comp Neurol. 2006;495:453-469.
McHaffie JG, Stanford TR, Stein BE, et al. Subcortical loops through the basal ganglia. Trends Neurosci. 2005;28:401-407.
Mena-Segovia J, Winn P, Bolam JP. Cholinergic modulation of midbrain dopaminergic systems. Brain Res Rev. 2008;58:265-271.
Middleton FA, Strick PL. Anatomical evidence for cerebellar and basal ganglia involvement in higher cognitive function. Science. 1994;266:458-461.
Nambu A, Tokuno H, Takada M. Functional significance of the cortico-subthalamo-pallidal ‘hyperdirect’ pathway. Neurosci Res. 2002;43:111-117.
Pahapill PA, Lozano AM. The pedunculopontine nucleus and Parkinson’s disease. Brain. 2000;123:1767-1783.
Parent A, Hazrati LN. Functional anatomy of the basal ganglia. I. The cortico-basal ganglia-thalamo-cortical loop. Brain Res Rev. 1995;20:91-127.
Redgrave P, Gurney K, Reynolds J. What is reinforced by phasic dopamine signals? Brain Res Rev. 2008;58:322-339.
Rommelfanger KS, Weinshenker D. Norepinephrine: the redheaded stepchild of Parkinson’s disease. Biochem Pharmacol. 2007;74:177-190.
Sanchez-Gonzalez MA, Garcia-Cabezas MA, Rico B, et al. The primate thalamus is a key target for brain dopamine. J Neurosci. 2005;25:6076-6083.
Schultz W. Multiple dopamine functions at different time courses. Annu Rev Neurosci. 2007;30:259-288.
Smith Y, Kieval JZ. Anatomy of the dopamine system in the basal ganglia. Trends Neurosci. 2000;23:S28-S33.
Smith Y, Raju D, Nanda B, et al. The thalamostriatal systems: anatomical and functional organization in normal and parkinsonian states. Brain Res Bull. 2009;78:60-68.
Stefani A, Lozano AM, Peppe A, et al. Bilateral deep brain stimulation of the pedunculopontine and subthalamic nuclei in severe Parkinson’s disease. Brain. 2007;130:1596-1607.
Surmeier DJ, Ding J, Day M, et al. D1 and D2 dopamine-receptor modulation of striatal glutamatergic signaling in striatal medium spiny neurons. Trends Neurosci. 2007;30:228-235.
Villalba RM, Lee H, Smith Y. Dopaminergic denervation and spine loss in the striatum of MPTP-treated monkeys. Exp Neurol. 2009;215:220-227.
Wichmann T, Delong MR. Deep brain stimulation for neurologic and neuropsychiatric disorders. Neuron. 2006;52:197-204.
1 Parent A, Hazrati LN. Functional anatomy of the basal ganglia. I. The cortico-basal ganglia-thalamo-cortical loop. Brain Res Rev. 1995;20:91-127.
2 Gerfen CR, Wilson CJ. The basal ganglia. In: Björklund A, Hökfelt T, Swanson L, editors. Handbook of Chemical Neuroanatomy, Integrated Systems of the CNS, Part III. Amsterdam: Elsevier; 1996:369.
3 Middleton FA, Strick PL. New concepts about the organization of basal ganglia output. Adv Neurol. 1997;74:57-68.
4 Middleton FA, Strick PL. Basal ganglia output and cognition: evidence from anatomical, behavioral, and clinical studies. Brain Cogn. 2000;42:183-200.
5 Middleton FA, Strick PL. Basal ganglia and cerebellar loops: motor and cognitive circuits. Brain Res Rev. 2000;31:236-250.
6 Nicola SM, Surmeier J, Malenka RC. Dopaminergic modulation of neuronal excitability in the striatum and nucleus accumbens. Annu Rev Neurosci. 2000;23:185-215.
7 Gerfen CR. Dopamine-mediated gene regulation in models of Parkinson’s disease. Ann Neurol. 2000;47:S42-S50.
8 Parent A, Sato F, Wu Y, et al. Organization of the basal ganglia: the importance of axonal collateralization. Trends Neurosci. 2000;23:S20-S27.
9 Smith Y, Kieval JZ. Anatomy of the dopamine system in the basal ganglia. Trends Neurosci. 2000;23:S28-S33.
10 Bevan MD, Magill PJ, Terman D, et al. Move to the rhythm: oscillations in the subthalamic nucleus–external globus pallidus network. Trends Neurosci. 2002;25:525-531.
11 Afifi AK. The basal ganglia: a neural network with more than motor function. Semin Pediatr Neurol. 2003;10:3-10.
12 Graybiel AM. Network-level neuroplasticity in cortico-basal ganglia pathways. Parkinsonism Relat Disord. 2004;10:293-296.
13 Kelly RM, Strick PL. Macro-architecture of basal ganglia loops with the cerebral cortex: use of rabies virus to reveal multisynaptic circuits. Prog Brain Res. 2004;143:449-459.
14 Smith Y, Raju DV, Pare JF, et al. The thalamostriatal system: a highly specific network of the basal ganglia circuitry. Trends Neurosci. 2004;27:520-527.
15 Tepper JM, Bolam JP. Functional diversity and specificity of neostriatal interneurons. Curr Opin Neurobiol. 2004;14:685-692.
16 Tepper JM, Koós T, Wilson CJ. GABAergic microcircuits in the neostriatum. Trends Neurosci. 2004;27:662-669.
17 Tepper JM, Abercrombie ED, Bolam JP. Basal ganglia macrocircuits. Prog Brain Res. 2007;160:3-7.
18 McHaffie JG, Stanford TR, et al. Subcortical loops through the basal ganglia. Trends Neurosci. 2005;28:401-407.
19 Boyes J, Bolam JP. Localization of GABA receptors in the basal ganglia. Prog Brain Res. 2007;160:229-243.
20 Hammond C, Bergman H, Brown P. Pathological synchronization in Parkinson’s disease: networks, models and treatments. Trends Neurosci. 2007;30:357-364.
21 Wichmann T, Delong MR. Anatomy and physiology of the basal ganglia: relevance to Parkinson’s disease and related disorders. Handb Clin Neurol. 2007;83:1-18.
22 Graybiel AM. The basal ganglia: learning new tricks and loving it. Curr Opin Neurobiol. 2005;15:638-644.
23 Graybiel AM. Habits, rituals, and the evaluative brain. Annu Rev Neurosci. 2008;31:359-387.
24 Pisani A, Bernardi G, Ding J, et al. Re-emergence of striatal cholinergic interneurons in movement disorders. Trends Neurosci. 2007;30:545-553.
25 Surmeier DJ, Ding J, Day M, et al. D1 and D2 dopamine-receptor modulation of striatal glutamatergic signaling in striatal medium spiny neurons. Trends Neurosci. 2007;30:228-235.
26 Wilson CJ. GABAergic inhibition in the neostriatum. Prog Brain Res. 2007;160:91-110.
27 Haber SN. The primate basal ganglia: parallel and integrative networks. J Chem Neuroanat. 2003;26:317-330.
28 Haber S. Parallel and integrative processing through the basal ganglia reward circuit: lessons from addiction. Biol Psychiatry. 2008;64:173-174.
29 Braak H, Del Tredici K. Cortico-basal ganglia-cortical circuitry in Parkinson’s disease reconsidered. Exp Neurol. 2008;212:226-229.
30 Israel Z, Bergman H. Pathophysiology of the basal ganglia and movement disorders: from animal models to human clinical applications. Neurosci Biobehav Rev. 2008;32:367-377.
31 Obeso JA, Rodriguez-Oroz MC, Benitez-Temino B, et al. Functional organization of the basal ganglia: therapeutic implications for Parkinson’s disease. Mov Disord. 2008;23(suppl 3):S548-S559.
32 Redgrave P, Gurney K, et al. What is reinforced by phasic dopamine signals? Brain Res Rev. 2008;58:322-339.
33 Smith Y, Villalba R. Striatal and extrastriatal dopamine in the basal ganglia: an overview of its anatomical organization in normal and parkinsonian brains. Mov Disord. 2008;23(suppl 3):S534-547.
34 Tepper JM, Wilson CJ, Koos T. Feedforward and feedback inhibition in neostriatal GABAergic spiny neurons. Brain Res Rev. 2008;58:272-281.
35 Yelnik J. Modeling the organization of the basal ganglia. Rev Neurol (Paris). 2008;164:969-976.
36 Nambu A. Seven problems on the basal ganglia. Curr Opin Neurobiol. 2008;18:595-604.
37 Smith Y, Raju D, et al. The thalamostriatal systems: anatomical and functional organization in normal and parkinsonian states. Brain Res Bull. 2009;78:60-68.
38 Haber SN, Gdowski MJ. The basal ganglia. In: Paxinos G, Mai JK, editors. The Human Nervous System. 2nd ed. Amsterdam: Academic Press; 2004:677-738.
39 Schultz W. Behavioral theories and the neurophysiology of reward. Annu Rev Psychol. 2006;57:87-115.
40 Schultz W. Multiple dopamine functions at different time courses. Annu Rev Neurosci. 2007;30:259-288.
41 Schultz W. Behavioral dopamine signals. Trends Neurosci. 2007;30:203-210.
42 Baláz M, Rektor I, Pulkrábek J. Participation of the subthalamic nucleus in executive functions: an intracerebral recording study. Mov Disord. 2008;23:553-557.
43 Benabid AL, Chabardes S, Mitrofanis J, et al. Deep brain stimulation of the subthalamic nucleus for the treatment of Parkinson’s disease. Lancet Neurol. 2009;8:67-81.
44 Benarroch EE. Subthalamic nucleus and its connections: anatomic substrate for the network effects of deep brain stimulation. Neurology. 2008;70:1991-1995.
45 DeLong MR, Wichmann T. Deep brain stimulation for Parkinson’s disease. Ann Neurol. 2001;49:142-143.
46 Limousin P, Martinez-Torres I. Deep brain stimulation for Parkinson’s disease. Neurotherapeutics. 2008;5:309-319.
47 Lozano AM, Snyder BJ. Deep brain stimulation for parkinsonian gait disorders. J Neurol. 2008;255(suppl 4):30-31.
48 Temel Y. Subthalamic nucleus stimulation in Parkinson’s disease: the other side of the medallion. Exp Neurol. 2008;211:321-323.
49 Tommasi G, Krack P, Fraix V, et al. Pyramidal tract side effects induced by deep brain stimulation of the subthalamic nucleus. J Neurol Neurosurg Psychiatry. 2008;79:813-819.
50 Videnovic A, Metman LV. Deep brain stimulation for Parkinson’s disease: prevalence of adverse events and need for standardized reporting. Mov Disord. 2008;23:343-349.
51 Wichmann T, Delong MR. Deep brain stimulation for neurologic and neuropsychiatric disorders. Neuron. 2006;52:197-204.
52 Yu H, Neimat JS. The treatment of movement disorders by deep brain stimulation. Neurotherapeutics. 2008;5:26-36.
53 Graybiel AM. Neurotransmitters and neuromodulators in the basal ganglia. Trends Neurosci. 1990;13:244-254.
54 Saka E, Graybiel AM. Pathophysiology of Tourette’s syndrome: striatal pathways revisited. Brain Dev. 2003;25(suppl 1):S15-S19.
55 Saka E, Goodrich C, Harlan P. Repetitive behaviors in monkeys are linked to specific striatal activation patterns. J Neurosci. 2004;24:7557-7565.
56 Canales JJ, Graybiel AM. A measure of striatal function predicts motor stereotypy. Nat Neurosci. 2000;3:377-383.
57 Canales JJ. Stimulant-induced adaptations in neostriatal matrix and striosome systems: transiting from instrumental responding to habitual behavior in drug addiction. Neurobiol Learn Mem. 2005;83:93-103.
58 Crittenden JR, Cantuti-Castelvetri I, Daka E, et al. Dysregulation of CalDAG-GEFI and CalDAG-GEFII predicts the severity of motor side-effects induced by anti-parkinsonian therapy. Proc Natl Acad Sci U S A. 2009;106:2892-2896.
59 Goto S, Lee LV, Munoz EL, et al. Functional anatomy of the basal ganglia in X-linked recessive dystonia-parkinsonism. Ann Neurol. 2005;58:7-17.
60 Sato K, Sumi-Ichinose C, Kaji R, et al. Differential involvement of striosome and matrix dopamine systems in a transgenic model of dopa-responsive dystonia. Proc Natl Acad Sci U S A. 2008;105:12551-12556.
61 Tippett LJ, Waldvogel HJ, Thomas SJ, et al. Striosomes and mood dysfunction in Huntington’s disease. Brain. 2007;130:206-221.
62 Oorschot DE. Total number of neurons in the neostriatal, pallidal, subthalamic, and substantia nigral nuclei of the rat basal ganglia: a stereological study using the cavalieri and optical dissector methods. J Comp Neurol. 1996;366:580-599.
63 Gertler TS, Chan CS, Surmeier DJ. Dichotomous anatomical properties of adult striatal medium spiny neurons. J Neurosci. 2008;28:10814-10824.
64 Kreitzer AC, Malenka RC. Endocannabinoid-mediated rescue of striatal LTD and motor deficits in Parkinson’s disease models. Nature. 2007;445:643-647.
65 Cepeda C, André VM, Yamazaki I, et al. Differential electrophysiological properties of dopamine D1 and D2 receptor–containing striatal medium-sized spiny neurons. Eur J Neurosci. 2008;27:671-682.
66 Day M, Wokosin D, Plotkin JL, et al. Differential excitability and modulation of striatal medium spiny neuron dendrites. J Neurosci. 2008;28:11603-11614.
67 Ingham CA, Hood SH, Aubuthnott GW. Spine density on neostriatal neurones changes with 6-hydroxydopamine lesions and with age. Brain Res. 1989;503:334-338.
68 Day M, Wang Z, Ding J, et al. Selective elimination of glutamatergic synapses on striatopallidal neurons in Parkinson disease models. Nat Neurosci. 2006;9:251-259.
69 Wickens JR, Arbuthnott GW, Shindou T. Simulation of GABA function in the basal ganglia: computational models of GABAergic mechanisms in basal ganglia function. Prog Brain Res. 2007;160:313-329.
70 Villalba RM, Lee H, Smith Y. Dopaminergic denervation and spine loss in the striatum of MPTP-treated monkeys. Exp Neurol. 2009;215:220-227.
71 Wilson CJ, Groves PM. Fine structure and synaptic connections of the common spiny neuron of the rat neostriatum: a study employing intracellular inject of horseradish peroxidase. J Comp Neurol. 1980;194:599-615.
72 Jaeger D, Kita H, Wilson CJ. Surround inhibition among projection neurons is weak or nonexistent in the rat neostriatum. J Neurophysiol. 1994;72:2555-2558.
73 Koos T, Tepper JM, Wilson CJ. Comparison of IPSCs evoked by spiny and fast-spiking neurons in the neostriatum. J Neurosci. 2004;24:7916-7922.
74 Taverna S, Ilijic E, Surmeier DJ. Recurrent collateral connections of striatal medium spiny neurons are disrupted in models of Parkinson’s disease. J Neurosci. 2008;28:5504-5512.
75 Graveland GA, DiFiglia M. The frequency and distribution of medium-sized neurons with indented nuclei in the primate and rodent neostriatum. Brain Res. 1985;327:307-311.
76 Kawaguchi Y, Wilson CJ, Augood SJ, et al. Striatal interneurones: chemical, physiological and morphological characterization. Trends Neurosci. 1995;18:527-535.
77 Aosaki T, Graybiel AM, Kimura M. Effect of the nigrostriatal dopamine system on acquired neural responses in the striatum of behaving monkeys. Science. 1994;265:412-415.
78 Aosaki T, Tsubokawa H, Ishida A, et al. Responses of tonically active neurons in the primate’s striatum undergo systematic changes during behavioral sensorimotor conditioning. J Neurosci. 1994;14:3969-3984.
79 Bennett BD, Wilson CJ. Spontaneous activity of neostriatal cholinergic interneurons in vitro. J Neurosci. 1999;19:5586-5596.
80 Cicchetti F, Beach TG, Parent A. Chemical phenotype of calretinin interneurons in the human striatum. Synapse. 1998;30:284-297.
81 Smith AD, Bolam JP. The neural network of the basal ganglia as revealed by the study of synaptic connections of identified neurones. Trends Neurosci. 1990;13:259-265.
82 Graybiel AM, Aosaki T, Flaherty AW. The basal ganglia and adaptive motor control. Science. 1994;265:1826-1831.
83 Kimura M, Yamada H, Matsumoto N. Tonically active neurons in the striatum encode motivational contexts of action. Brain Dev. 2003;25(suppl 1):S20-23.
84 Kimura M, Matsumoto N, Okahashi K. Goal-directed, serial and synchronous activation of neurons in the primate striatum. Neuroreport. 2003;14:799-802.
85 Yamada H, Matsumoto N, Kimura M. Tonically active neurons in the primate caudate nucleus and putamen differentially encode instructed motivational outcomes of action. J Neurosci. 2004;24:3500-3510.
86 Apicella P. Leading tonically active neurons of the striatum from reward detection to context recognition. Trends Neurosci. 2007;30:299-306.
87 Tan CO, Bullock D. A dopamine-acetylcholine cascade: simulating learned and lesion-induced behavior of striatal cholinergic interneurons. J Neurophysiol. 2008;100:2409-2421.
88 Joshua M, Adler A, Mitelman R. Midbrain dopaminergic neurons and striatal cholinergic interneurons encode the difference between reward and aversive events at different epochs of probabilistic classical conditioning trials. J Neurosci. 2008;28:11673-11684.
89 Joshua M, Adler A, Rosin B. Encoding of probabilistic rewarding and aversive events by pallidal and nigral neurons. J Neurophysiol. 2009;101:758-772.
90 Sullivan MA, Chen H, Morikawa H. Recurrent inhibitory network among striatal cholinergic interneurons. J Neurosci. 2008;28:8682-8690.
91 Wang Z, Kai L, Day M, et al. Dopaminergic control of corticostriatal long-term synaptic depression in medium spiny neurons is mediated by cholinergic interneurons. Neuron. 2006;50:443-452.
92 Shen W, Tian X, Day M, et al. Cholinergic modulation of Kir2 channels selectively elevates dendritic excitability in striatopallidal neurons. Nat Neurosci. 2007;10:1458-1466.
93 Ding J, Guzman JN, Tkatch T, et al. RGS4-dependent attenuation of M4 autoreceptor function in striatal cholinergic interneurons following dopamine depletion. Nat Neurosci. 2006;9:832-842.
94 Maurice N, Mercer J, Chan CS, et al. D2 dopamine receptor–mediated modulation of voltage-dependent Na+ channels reduces autonomous activity in striatal cholinergic interneurons. J Neurosci. 2004;24:10289-10301.
95 Cabrera-Vera TM, Hernandez S, Earls LR, et al. RGS9-2 modulates D2 dopamine receptor–mediated Ca2+ channel inhibition in rat striatal cholinergic interneurons. Proc Natl Acad Sci U S A. 2004;101:16339-16344.
96 Berke JD. Uncoordinated firing rate changes of striatal fast-spiking interneurons during behavioral task performance. J Neurosci. 2008;28:10075-10080.
97 Galarraga E, Vilchis C, Tkatch T, et al. Somatostatinergic modulation of firing pattern and calcium-activated potassium currents in medium spiny neostriatal neurons. Neuroscience. 2007;146:537-554.
98 Alexander GE, DeLong MR, Strick PL. Parallel organization of functionally segregated circuits linking basal ganglia and cortex. Annu Rev Neurosci. 1986;9:357-381.
99 Gerfen CR. The neostriatal mosaic: compartmentalization of corticostriatal input and striatonigral output systems. Nature. 1984;311:461-464.
100 McGeorge AJ, Faull RL. The organization of the projection from the cerebral cortex to the striatum in the rat. Neuroscience. 1989;29:503-537.
101 Reep RL, Cheatwood JL, Corwin JV. The associative striatum: organization of cortical projections to the dorsocentral striatum in rats. J Comp Neurol. 2003;467:271-292.
102 Alloway KD, Lou L, Nwabueze-Ogbo F, et al. Topography of cortical projections to the dorsolateral neostriatum in rats: multiple overlapping sensorimotor pathways. J Comp Neurol. 2006;499:33-48.
103 McHaffie JG, Thomson CM, Stein BE. Corticotectal and corticostriatal projections from the frontal eye fields of the cat: an anatomical examination using WGA-HRP. Somatosens Mot Res. 2001;18:117-130.
104 Gerardin E, Lehericy S, Pochon JB, et al. Foot, hand, face and eye representation in the human striatum. Cereb Cortex. 2003;13:162-169.
105 Lehericy S, Ducros M, Krainik A, et al. 3-D diffusion tensor axonal tracking shows distinct SMA and pre-SMA projections to the human striatum. Cereb Cortex. 2004;14:1302-1309.
106 Lehericy S, Ducros M, Van de Moortele PF, et al. Diffusion tensor fiber tracking shows distinct corticostriatal circuits in humans. Ann Neurol. 2004;55:522-529.
107 Wiesendanger E, Clarke S, Kraftsik R, et al. Topography of cortico-striatal connections in man: anatomical evidence for parallel organization. Eur J Neurosci. 2004;20:1915-1922.
108 Postuma RB, Dagher A. Basal ganglia functional connectivity based on a meta-analysis of 126 positron emission tomography and functional magnetic resonance imaging publications. Cereb Cortex. 2006;16:1508-1521.
109 Dominey PF, Inui T, Hoen M. Neural network processing of natural language: II. Towards a unified model of corticostriatal function in learning sentence comprehension and non-linguistic sequencing. Brain Lang. 2009;109:80-92.
110 Nagano-Saito A, Leyton M, Monchi O. Dopamine depletion impairs frontostriatal functional connectivity during a set-shifting task. J Neurosci. 2008;28:3697-3706.
111 Seger CA. How do the basal ganglia contribute to categorization? Their roles in generalization, response selection, and learning via feedback. Neurosci Biobehav Rev. 2008;32:265-278.
112 Nakano K, Kayahara T, Tsutsumi T. Neural circuits and functional organization of the striatum. J Neurol. 2000;247(suppl 5):V1-V15.
113 Nakano K. Neural circuits and topographic organization of the basal ganglia and related regions. Brain Dev. 2000;22(suppl 1):S5-S16.
114 Haber SN, Kim KS, Mailly P, et al. Reward-related cortical inputs define a large striatal region in primates that interface with associative cortical connections, providing a substrate for incentive-based learning. J Neurosci. 2006;26:8368-8376.
115 Calzavara R, Mailly P, Haber SN. Relationship between the corticostriatal terminals from areas 9 and 46, and those from area 8A, dorsal and rostral premotor cortex and area 24c: an anatomical substrate for cognition to action. Eur J Neurosci. 2007;26:2005-2024.
116 Yeterian EH, Van Hoesen GW. Cortico-striate projections in the rhesus monkey: the organization of certain cortico-caudate connections. Brain Res. 1978;139:43-63.
117 Selemon LD, Goldman-Rakic PS. Longitudinal topography and interdigitation of corticostriatal projections in the rhesus monkey. J Neurosci. 1985;5:776-794.
118 Parthasarathy HB, Schall JD, Graybiel AM. Distributed but convergent ordering of corticostriatal projections: analysis of the frontal eye field and the supplementary eye field in the macaque monkey. J Neurosci. 1992;12:4468-4488.
119 Flaherty AW, Graybiel AM. Two input systems for body representations in the primate striatal matrix: experimental evidence in the squirrel monkey. J Neurosci. 1993;13:1120-1137.
120 Reiner A, Jiao Y, Del Mar N, et al. Differential morphology of pyramidal tract–type and intratelencephalically projecting–type corticostriatal neurons and their intrastriatal terminals in rats. J Comp Neurol. 2003;457:420-440.
121 Lei W, Jiao Y, Del Mar N, et al. Evidence for differential cortical input to direct pathway versus indirect pathway striatal projection neurons in rats. J Neurosci. 2004;24:8289-8299.
122 Ballion B, Mallet N, Bezard E, et al. Intratelencephalic corticostriatal neurons equally excite striatonigral and striatopallidal neurons and their discharge activity is selectively reduced in experimental parkinsonism. Eur J Neurosci. 2008;27:2313-2321.
123 Jones EG, Coulter JD, Burton H, et al. Cells of origin and terminal distribution of corticostriatal fibers arising in the sensory-motor cortex of monkeys. J Comp Neurol. 1997;173:53-80.
124 Turner RS, DeLong MR. Corticostriatal activity in primary motor cortex of the macaque. J Neurosci. 2000;20:7096-7108.
125 Parent M, Parent A. Single-axon tracing study of corticostriatal projections arising from primary motor cortex in primates. J Comp Neurol. 2006;496:202-213.
126 Kemp JM, Powell TP. The termination of fibres from the cerebral cortex and thalamus upon dendritic spines in the caudate nucleus: a study with the Golgi method. Philos Trans R Soc Lond B Biol Sci. 1971;262:429-439.
127 Lapper SR, Smith Y, Sadikot AF, et al. Cortical input to parvalbumin-immunoreactive neurones in the putamen of the squirrel monkey. Brain Res. 1992;580:215-224.
128 Mallet N, Le Moine C, Charpier S, et al. Feedforward inhibition of projection neurons by fast-spiking GABA interneurons in the rat striatum in vivo. J Neurosci. 2005;25:3857-3869.
129 Mallet N, Ballion B, Le Moine C, et al. Cortical inputs and GABA interneurons imbalance projection neurons in the striatum of parkinsonian rats. J Neurosci. 2006;26:3875-3884.
130 Parent M, Parent A. Single-axon tracing and three-dimensional reconstruction of centre median–parafascicular thalamic neurons in primates. J Comp Neurol. 2005;481:127-144.
131 Sadikot AF, Parent A, Francois C. Efferent connections of the centromedian and parafascicular thalamic nuclei in the squirrel monkey: a PHA-L study of subcortical projections. J Comp Neurol. 1992;315:137-159.
132 Sidibe M, Smith Y. Differential synaptic innervation of striatofugal neurones projecting to the internal or external segments of the globus pallidus by thalamic afferents in the squirrel monkey. J Comp Neurol. 1996;365:445-465.
133 Raju DV, Shah DJ, Wright TM, et al. Differential synaptology of vGluT2-containing thalamostriatal afferents between the patch and matrix compartments in rats. J Comp Neurol. 2006;499:231-243.
134 Sidibe M, Smith Y. Thalamic inputs to striatal interneurons in monkeys: synaptic organization and co-localization of calcium binding proteins. Neuroscience. 1999;89:1189-1208.
135 Wilson CJ, Chang HT, Kitai ST. Origins of post synaptic potentials evoked in spiny neostriatal projection neurons by thalamic stimulation in the rat. Exp Brain Res. 1983;51:217-226.
136 Zackheim J, Abercrombie ED. Thalamic regulation of striatal acetylcholine efflux is both direct and indirect and qualitatively altered in the dopamine-depleted striatum. Neuroscience. 2005;131:423-436.
137 Nanda B, Galvan A, Smith Y, et al. Effects of stimulation of the centromedian nucleus of the thalamus on the activity of striatal cells in awake rhesus monkeys. Eur J Neurosci. 2009;29:588-598.
138 Minamimoto T, Kimura M. Participation of the thalamic CM-Pf complex in attentional orienting. J Neurophysiol. 2002;87:3090-3101.
139 Minamimoto T, Hori Y, Kimura M. Complementary process to response bias in the centromedian nucleus of the thalamus. Science. 2005;308:1798-1801.
140 Kimura M, Minamimoto T, Matsumoto N, et al. Monitoring and switching of cortico-basal ganglia loop functions by the thalamo-striatal system. Neurosci Res. 2004;48:355-360.
141 Groenewegen HJ, Berendse HW. The specificity of the ‘nonspecific’ midline and intralaminar thalamic nuclei. Trends Neurosci. 1994;17:52-57.
142 McFarland NR, Haber SN. Convergent inputs from thalamic motor nuclei and frontal cortical areas to the dorsal striatum in the primate. J Neurosci. 2000;20:3798-3813.
143 Haber SN, Calzavara R. The cortico-basal ganglia integrative network: the role of the thalamus. Brain Res Bull. 2009;78:69-74.
144 Lacey CJ, Bolam JP, Magill PJ. Novel and distinct operational principles of intralaminar thalamic neurons and their striatal projections. J Neurosci. 2007;27:4374-4384.
145 Raju DV, Ahern TH, Shah DJ, et al. Differential synaptic plasticity of the corticostriatal and thalamostriatal systems in an MPTP-treated monkey model of parkinsonism. Eur J Neurosci. 2008;27:1647-1658.
146 Sadikot AF, Parent A, Smith Y, et al. Efferent connections of the centromedian and parafascicular thalamic nuclei in the squirrel monkey: a light and electron microscopic study of the thalamostriatal projection in relation to striatal heterogeneity. J Comp Neurol. 1992;320:228-242.
147 Raju DV, Smith Y. Differential localization of vesicular glutamate transporters 1 and 2 in the rat striatum. In: Bolam JPet al, editor. The Basal Ganglia VIII. New York: Plenum Press; 2005:601-610.
148 Kinomura S, Larsson J, Gulyas B, et al. Activation by attention of the human reticular formation and thalamic intralaminar nuclei. Science. 1996;271:512-515.
149 Schiff ND, Giacino JT, Kalmar KA, et al. Behavioural improvements with thalamic stimulation after severe traumatic brain injury. Nature. 2007;448:600-603.
150 Mair RG, Hembrook JR. Memory enhancement with event-related stimulation of the rostral intralaminar thalamic nuclei. J Neurosci. 2008;28:14293-14300.
151 Haber S, McFarland NR. The place of the thalamus in frontal cortical–basal ganglia circuits. Neuroscientist. 2001;7:315-324.
152 Henderson JM, Carpenter K, Cartwright H, et al. Loss of thalamic intralaminar nuclei in progressive supranuclear palsy and Parkinson’s disease: clinical and therapeutic implications. Brain. 2000;123:1410-1421.
153 Henderson JM, Carpenter K, Cartwright H, et al. Degeneration of the centré median–parafascicular complex in Parkinson’s disease. Ann Neurol. 2000;47:345-352.
154 McKeown MJ, Uthama A, Abugharbieh R, et al. Shape (but not volume) changes in the thalami in Parkinson disease. BMC Neurol. 2008;8:8.
155 Seroogy K, Ceccatelli S, Schalling M, et al. A subpopulation of dopaminergic neurons in rat ventral mesencephalon contains both neurotensin and cholecystokinin. Brain Res. 1988;455:88-98.
156 Seroogy KB, Dangaran K, Lim S, et al. Ventral mesencephalic neurons containing both cholecystokinin- and tyrosine hydroxylase–like immunoreactivities project to forebrain regions. J Comp Neurol. 1989;279:397-414.
157 Seutin V. Dopaminergic neurones: much more than dopamine? Br J Pharmacol. 2005;146:167-169.
158 Binder EB, Kinkead B, Owens MJ, et al. Neurotensin and dopamine interactions. Pharmacol Rev. 2001;53:453-486.
159 Haber SN, Ryoo H, Cox C, et al. Subsets of midbrain dopaminergic neurons in monkeys are distinguished by different levels of mRNA for the dopamine transporter: comparison with the mRNA for the D2 receptor, tyrosine hydroxylase and calbindin immunoreactivity. J Comp Neurol. 1995;362:400-410.
160 Sanghera MK, Manaye K, McMahon A, et al. Dopamine transporter mRNA levels are high in midbrain neurons vulnerable to MPTP. Neuroreport. 1997;8:3327-3331.
161 Bezard E, Gross CE, Fournier MC, et al. Absence of MPTP-induced neuronal death in mice lacking the dopamine transporter. Exp Neurol. 1999;155:268-273.
162 Gerfen CR, Baimbridge KG, Miller JJ. The neostriatal mosaic: compartmental distribution of calcium-binding protein and parvalbumin in the basal ganglia of the rat and monkey. Proc Natl Acad Sci U S A. 1985;82:8780-8784.
163 Yamada T, McGeer PL, Baimbridge KG, et al. Relative sparing in Parkinson’s disease of substantia nigra dopamine neurons containing calbindin-D28K. Brain Res. 1990;526:303-307.
164 Damier P, Hirsch EC, Agid Y, et al. The substantia nigra of the human brain. II. Patterns of loss of dopamine-containing neurons in Parkinson’s disease. Brain. 1999;122:1437-1448.
165 Iravani MM, Syed E, Jackson MJ, et al. A modified MPTP treatment regime produces reproducible partial nigrostriatal lesions in common marmosets. Eur J Neurosci. 2005;21:841-854.
166 Karachi C, Francois C, Parain K, et al. Three-dimensional cartography of functional territories in the human striatopallidal complex by using calbindin immunoreactivity. J Comp Neurol. 2002;450:122-134.
167 Iacopino A, Christakos S, German D, et al. Calbindin-D28K–containing neurons in animal models of neurodegeneration: possible protection from excitotoxicity. Brain Res Mol Brain Res. 1992;13:251-261.
168 Gerfen CR, Herkenham M, Thibault J. The neostriatal mosaic: II. Patch- and matrix-directed mesostriatal dopaminergic and non-dopaminergic systems. J Neurosci. 1987;7:3915-3934.
169 Prensa L, Parent A. The nigrostriatal pathway in the rat: a single-axon study of the relationship between dorsal and ventral tier nigral neurons and the striosome/matrix striatal compartments. J Neurosci. 2001;21:7247-7260.
170 Matsuda W, Furuta T, Nakamura KC, et al. Single nigrostriatal dopaminergic neurons form widely spread and highly dense axonal arborizations in the neostriatum. J Neurosci. 2009;29:444-453.
171 Kish SJ, Shannak K, Hornykiewicz O. Uneven pattern of dopamine loss in the striatum of patients with idiopathic Parkinson’s disease. Pathophysiologic and clinical implications. N Engl J Med. 1998;318:876-880.
172 Brooks DJ, Ibanez V, Sawle GV, et al. Differing patterns of striatal 18F-dopa uptake in Parkinson’s disease, multiple system atrophy, and progressive supranuclear palsy. Ann Neurol. 1990;28:547-555.
173 Damier P, Hirsch EC, Agid Y, et al. The substantia nigra of the human brain. I. Nigrosomes and the nigral matrix, a compartmental organization based on calbindin D(28K) immunohistochemistry. Brain. 1999;122:1421-1436.
174 Gibb WR, Lees AJ. Anatomy, pigmentation, ventral and dorsal subpopulations of the substantia nigra, and differential cell death in Parkinson’s disease. J Neurol Neurosurg Psychiatry. 1991;54:388-396.
175 Moratalla R, Quinn B, DeLanney LE, et al. Differential vulnerability of primate caudate-putamen and striosome-matrix dopamine systems to the neurotoxic effects of 1-methyl-4-phenyl-1,2,3,6-tetrahydropyridine. Proc Natl Acad Sci U S A. 1992;89:3859-3863.
176 Fornai F, Ruffoli R, Soldani P, et al. The “parkinsonian heart”: from novel vistas to advanced therapeutic approaches in Parkinson’s disease. Curr Med Chem. 2007;14:2421-2428.
177 Fornai F, di Poggio AB, Pellegrini A, et al. Noradrenaline in Parkinson’s disease: from disease progression to current therapeutics. Curr Med Chem. 2007;14:2330-2334.
178 Rommelfanger KS, Edwards GL, Freeman KG, et al. Norepinephrine loss produces more profound motor deficits than MPTP treatment in mice. Proc Natl Acad Sci U S A. 2007;104:13804-13809.
179 Rommelfanger KS, Weinshenker D. Norepinephrine: the redheaded stepchild of Parkinson’s disease. Biochem Pharmacol. 2007;74:177-190.
180 Carta M, Carlsson T, Munoz A, et al. Serotonin-dopamine interaction in the induction and maintenance of L-DOPA–induced dyskinesias. Prog Brain Res. 2008;172:465-478.
181 Carta AR, Frau L, Pontis S, et al. Direct and indirect striatal efferent pathways are differentially influenced by low and high dyskinetic drugs: behavioural and biochemical evidence. Parkinsonism Relat Disord. 2008;14(suppl 2):S165-S168.
182 Fox SH, Chuang R, Brotchie JM. Parkinson’s disease—opportunities for novel therapeutics to reduce the problems of levodopa therapy. Prog Brain Res. 2008;172:479-494.
183 Betarbet R, Turner R, Chockkan V, et al. Dopaminergic neurons intrinsic to the primate striatum. J Neurosci. 1997;17:6761-6768.
184 Cossette M, Levesque D, Parent A. Neurochemical characterization of dopaminergic neurons in human striatum. Parkinsonism Relat Disord. 2005;11:277-286.
185 Cossette M, Lecomte F, Parent A. Morphology and distribution of dopaminergic neurons intrinsic to the human striatum. J Chem Neuroanat. 2005;29:1-11.
186 Mazloom M, Smith Y. Synaptic microcircuitry of tyrosine hydroxylase–containing neurons and terminals in the striatum of 1-methyl-4-phenyl-1,2,3,6-tetrahydropyridine–treated monkeys. J Comp Neurol. 2006;495:453-469.
187 Tande D, Hoglinger G, Debeir T, et al. New striatal dopamine neurons in MPTP-treated macaques result from a phenotypic shift and not neurogenesis. Brain. 2006;129:1194-1200.
188 Palfi S, Leventhal L, Chu Y, et al. Lentivirally delivered glial cell line–derived neurotrophic factor increases the number of striatal dopaminergic neurons in primate models of nigrostriatal degeneration. J Neurosci. 2002;22:4942-4954.
189 Freeman A, Ciliax B, Bakay R, et al. Nigrostriatal collaterals to thalamus degenerate in parkinsonian animal models. Ann Neurol. 2001;50:321-329.
190 Sánchez-González MA, García-Cabezas MA, Rico B, et al. The primate thalamus is a key target for brain dopamine. J Neurosci. 2005;25:6076-6083.
191 García-Cabezas MA, Rico B, Sánchez-González MA, et al. Distribution of the dopamine innervation in the macaque and human thalamus. Neuroimage. 2007;34:965-984.
192 García-Cabezas MA, Martínez-Sánchez P, Sánchez-González MA, et al. Dopamine innervation in the thalamus: monkey versus rat. Cereb Cortex. 2009;19:424-434.
193 Albin RL, Young AB, Penney JB. The functional anatomy of basal ganglia disorders. Trends Neurosci. 1989;12:366-375.
194 Bergman H, Wichmann T, DeLong MR. Reversal of experimental parkinsonism by lesions of the subthalamic nucleus. Science. 1990;249:1436-1438.
195 Gerfen CR, Engber TM, Mahan LC, et al. D1 and D2 dopamine receptor–regulated gene expression of striatonigral and striatopallidal neurons. Science. 1990;250:1429-1432.
196 Kawaguchi Y, Wilson CJ, Emson PC. Projection subtypes of rat neostriatal matrix cells revealed by intracellular injection of biocytin. J Neurosci. 1990;10:3421-3438.
197 Parent A, Charara A, Pinault D. Single striatofugal axons arborizing in both pallidal segments and in the substantia nigra in primates. Brain Res. 1995;698:280-284.
198 Levesque M, Parent A. The striatofugal fiber system in primates: a reevaluation of its organization based on single-axon tracing studies. Proc Natl Acad Sci U S A. 2005;102:11888-11893.
199 Le Moine C, Normand E, Bloch B. Phenotypical characterization of the rat striatal neurons expressing the D1 dopamine receptor gene. Proc Natl Acad Sci U S A. 1991;88:4205-4209.
200 Surmeier DJ, Eberwine J, Wilson CJ, et al. Dopamine receptor subtypes colocalize in rat striatonigral neurons. Proc Natl Acad Sci U S A. 1992;89:10178-10182.
201 Surmeier DJ, Reiner A, Levine MS, et al. Are neostriatal dopamine receptors co-localized? Trends Neurosci. 1993;16:299-305.
202 Gerfen CR, Keefe KA. Neostriatal dopamine receptors. Trends Neurosci. 1994;17:2-3.
203 Le Moine C, Bloch B. D1 and D2 dopamine receptor gene expression in the rat striatum: sensitive cRNA probes demonstrate prominent segregation of D1 and D2 mRNAs in distinct neuronal populations of the dorsal and ventral striatum. J Comp Neurol. 1995;355:418-426.
204 Svenningsson P, Le Moine C, Aubert I, et al. Cellular distribution of adenosine A2A receptor mRNA in the primate striatum. J Comp Neurol. 1998;399:229-240.
205 Surmeier DJ, Song WJ, Yan Z. Coordinated expression of dopamine receptors in neostriatal medium spiny neurons. J Neurosci. 1996;16:6579-6591.
206 Hersch SM, Ciliax BJ, Gutekunst CA, et al. Electron microscopic analysis of D1 and D2 dopamine receptor proteins in the dorsal striatum and their synaptic relationships with motor corticostriatal afferents. J Neurosci. 1995;15:5222-5237.
207 Aizman O, Brismar H, Uhlen P, et al. Anatomical and physiological evidence for D1 and D2 dopamine receptor colocalization in neostriatal neurons. Nat Neurosci. 2000;3:226-230.
208 Deng YP, Lei WL, Reiner A. Differential perikaryal localization in rats of D1 and D2 dopamine receptors on striatal projection neuron types identified by retrograde labeling. J Chem Neuroanat. 2006;32:101.
209 Heintz N. BAC to the future: the use of BAC transgenic mice for neuroscience research. Nat Rev Neurosci. 2001;2:861-870.
210 Gong S, Zheng C, Doughty ML, et al. A gene expression atlas of the central nervous system based on bacterial artificial chromosomes. Nature. 2003;425:917-925.
211 Le Moine C, Bloch B. Expression of the D3 dopamine receptor in peptidergic neurons of the nucleus accumbens: comparison with the D1 and D2 dopamine receptors. Neuroscience. 1996;73:131-143.
212 Gurevich EV, Joyce JN. Distribution of dopamine D3 receptor expressing neurons in the human forebrain: comparison with D2 receptor expressing neurons. Neuropsychopharmacology. 1999;20:60-80.
213 Khan ZU, Gutierrez A, Martin R, et al. Dopamine D5 receptors of rat and human brain. Neuroscience. 2000;100:689-699.
214 Rivera A, Alberti I, Martin AB, et al. Molecular phenotype of rat striatal neurons expressing the dopamine D5 receptor subtype. Eur J Neurosci. 2002;16:2049-2058.
215 Noain D, Avale ME, Wedemeyer C, et al. Identification of brain neurons expressing the dopamine D4 receptor gene using BAC transgenic mice. Eur J Neurosci. 2006;24:2429-2438.
216 Araki KY, Sims JR, Bhide PG. Dopamine receptor mRNA and protein expression in the mouse corpus striatum and cerebral cortex during pre- and postnatal development. Brain Res. 2007;1156:31-45.
217 Nambu A, Tokuno H, Takada M. Functional significance of the cortico-subthalamo-pallidal ‘hyperdirect’ pathway. Neurosci Res. 2002;43:111-117.
218 Nambu A, Takada M, Inase M, et al. Dual somatotopical representations in the primate subthalamic nucleus: evidence for ordered but reversed body-map transformations from the primary motor cortex and the supplementary motor area. J Neurosci. 1996;16:2671-2683.
219 Strafella AP, Vanderwerf Y, Sadikot AF. Transcranial magnetic stimulation of the human motor cortex influences the neuronal activity of subthalamic nucleus. Eur J Neurosci. 2004;20:2245-2249.
220 Shink E, Bevan MD, Bolam JP, et al. The subthalamic nucleus and the external pallidum: two tightly interconnected structures that control the output of the basal ganglia in the monkey. Neuroscience. 1996;73:335-357.
221 Smith Y, Bevan MD, Shink E, et al. Microcircuitry of the direct and indirect pathways of the basal ganglia. Neuroscience. 1998;86:353-387.
222 Wichmann T, Bergman H, DeLong MR. The primate subthalamic nucleus. III. Changes in motor behavior and neuronal activity in the internal pallidum induced by subthalamic inactivation in the MPTP model of parkinsonism. J Neurophysiol. 1994;72:521-530.
223 Paradiso G, Cunic D, Saint-Cyr JA, et al. Involvement of human thalamus in the preparation of self-paced movement. Brain. 2004;127:2717-2731.
224 Baunez C, Humby T, Eagle DM, et al. Effects of STN lesions on simple vs choice reaction time tasks in the rat: preserved motor readiness, but impaired response selection. Eur J Neurosci. 2001;13:1609-1616.
225 Chudasama Y, Baunez C, Robbins TW. Functional disconnection of the medial prefrontal cortex and subthalamic nucleus in attentional performance: evidence for corticosubthalamic interaction. J Neurosci. 2003;23:5477-5485.
226 Winstanley CA, Baunez C, Theobald DE, et al. Lesions to the subthalamic nucleus decrease impulsive choice but impair autoshaping in rats: the importance of the basal ganglia in Pavlovian conditioning and impulse control. Eur J Neurosci. 2005;21:3107-3116.
227 Blandini F, Fancellu R, Armentero MT, et al. Unilateral lesion of the subthalamic nucleus enhances cortical fos expression associated with focally evoked seizures in the rat. Brain Res. 2006;1101:145-150.
228 Eagle DM, Baunez C, Hutcheson DM, et al. Stop-signal reaction-time task performance: role of prefrontal cortex and subthalamic nucleus. Cereb Cortex. 2008;18:178-188.
229 Le Jeune F, Peron J, Biseul I, et al. Subthalamic nucleus stimulation affects orbitofrontal cortex in facial emotion recognition: a PET study. Brain. 2008;131:1599-1608.
230 Tzagournissakis M, Dermon CR, Savaki HE. Functional metabolic mapping of the rat brain during unilateral electrical stimulation of the subthalamic nucleus. J Cereb Blood Flow Metab. 1994;14:132-144.
231 Maurice N, Deniau JM, Glowinski J. Relationships between the prefrontal cortex and the basal ganglia in the rat: physiology of the corticosubthalamic circuits. J Neurosci. 1998;18:9539-9546.
232 Kolomiets BP, Deniau JM, Mailly P. Segregation and convergence of information flow through the cortico-subthalamic pathways. J Neurosci. 2001;21:5764-5772.
233 Kolomiets BP, Deniau JM, Glowinski J. Basal ganglia and processing of cortical information: functional interactions between trans-striatal and trans-subthalamic circuits in the substantia nigra pars reticulata. Neuroscience. 2003;117:931-938.
234 Aron AR, Behrens TE, Smith S, et al. Triangulating a cognitive control network using diffusion-weighted magnetic resonance imaging (MRI) and functional MRI. J Neurosci. 2007;27:3743-3752.
235 Gerschlager W, Alesch F, Cunnington R. Bilateral subthalamic nucleus stimulation improves frontal cortex function in Parkinson’s disease. An electrophysiological study of the contingent negative variation. Brain. 1999;122:2365-2373.
236 Schroeder U, Kuehler A, Haslinger B, et al. Subthalamic nucleus stimulation affects striato-anterior cingulate cortex circuit in a response conflict task: a PET study. Brain. 2002;125:1995-2004.
237 Hershey T, Revilla FJ, Wernle A, et al. Stimulation of STN impairs aspects of cognitive control in PD. Neurology. 2004;62:1110-1114.
238 Haegelen C, Verin M, Broche BA. Does subthalamic nucleus stimulation affect the frontal limbic areas? A single-photon emission computed tomography study using a manual anatomical segmentation method. Surg Radiol Anat. 2005;27:389-394.
239 Funkiewiez A, Ardouin C, Caputo E. Long term effects of bilateral subthalamic nucleus stimulation on cognitive function, mood, and behaviour in Parkinson’s disease. J Neurol Neurosurg Psychiatry. 2004;75:834-839.
240 Funkiewiez A, Ardouin C, Cools R. Effects of levodopa and subthalamic nucleus stimulation on cognitive and affective functioning in Parkinson’s disease. Mov Disord. 2006;21:1656-1662.
241 Campbell MC, Karimi M, Weaver PM, et al. Neural correlates of STN DBS–induced cognitive variability in Parkinson disease. Neuropsychologia. 2008;46:3162-3169.
242 Kalbe E, Voges J, Weber T. Frontal FDG-PET activity correlates with cognitive outcome after STN-DBS in Parkinson disease. Neurology. 2009;72:42-49.
243 Parent A, Smith Y. Organization of efferent projections of the subthalamic nucleus in the squirrel monkey as revealed by retrograde labeling methods. Brain Res. 1987;436:296-310.
244 Smith Y, Hazrati LN, Parent A. Efferent projections of the subthalamic nucleus in the squirrel monkey as studied by the PHA-L anterograde tracing method. J Comp Neurol. 1990;294:306-323.
245 Degos B, Deniau JM, Le Cam J, et al. Evidence for a direct subthalamo-cortical loop circuit in the rat. Eur J Neurosci. 2008;27:2599-2610.
246 Kitai ST, Deniau JM. Cortical inputs to the subthalamus: intracellular analysis. Brain Res. 1981;214:411-415.
247 Giuffrida R, Li Volsi G, Maugeri G, et al. Influences of pyramidal tract on the subthalamic nucleus in the cat. Neurosci Lett. 1985;54:231-235.
248 Garcia-Rill E, Biedermann JA, Chambers T, et al. Mesopontine neurons in schizophrenia. Neuroscience. 1995;66:321-335.
249 Garcia-Rill E, Reese NB, Skinner RD. Arousal and locomotion: from schizophrenia to narcolepsy. Prog Brain Res. 1996;107:417-434.
250 Mesulam MM, Geula C, Bothwell MA, et al. Human reticular formation: cholinergic neurons of the pedunculopontine and laterodorsal tegmental nuclei and some cytochemical comparisons to forebrain cholinergic neurons. J Comp Neurol. 1989;283:611-633.
251 Lavoie B, Parent A. Pedunculopontine nucleus in the squirrel monkey: distribution of cholinergic and monoaminergic neurons in the mesopontine tegmentum with evidence for the presence of glutamate in cholinergic neurons. J Comp Neurol. 1994;344:190-209.
252 Inglis WL, Winn P. The pedunculopontine tegmental nucleus: where the striatum meets the reticular formation. Prog Neurobiol. 1995;47:1-29.
253 Rye DB. Contributions of the pedunculopontine region to normal and altered REM sleep. Sleep. 1997;20:757-788.
254 Winn P, Brown VJ, Inglis WL. On the relationships between the striatum and the pedunculopontine tegmental nucleus. Crit Rev Neurobiol. 1997;11:241-261.
255 Pahapill PA, Lozano AM. The pedunculopontine nucleus and Parkinson’s disease. Brain. 2000;123:1767-1783.
256 Winn P. Experimental studies of pedunculopontine functions: are they motor, sensory or integrative? Parkinsonism Relat Disord. 2008;14(suppl 2):S194-198.
257 Mena-Segovia J, Bolam JP, Magill PJ. Pedunculopontine nucleus and basal ganglia: distant relatives or part of the same family? Trends Neurosci. 2004;27:585-588.
258 Charara A, Smith Y, Parent A. Glutamatergic inputs from the pedunculopontine nucleus to midbrain dopaminergic neurons in primates: Phaseolus vulgaris–leucoagglutionin anterograde labeling combined with postembedding glutamate and GABA immunohistochemistry. J Comp Neurol. 1996;364:254-266.
259 Mena-Segovia J, Winn P, Bolam JP. Cholinergic modulation of midbrain dopaminergic systems. Brain Res Rev. 2008;58:265-271.
260 Sidibe M, Pare JF, Raju DV, et al. Anatomical and functional relationships between intralaminar thalamic nuclei and basal ganglia in monkeys. In: Nicholson LFB, Faull RLM, editors. The Basal Ganglia VII. New York: Kluwer; 2002:409-420.
261 Muthusamy KA, Aravamuthan BR, Kringelbach ML, et al. Connectivity of the human pedunculopontine nucleus region and diffusion tensor imaging in surgical targeting. J Neurosurg. 2007;107:814-820.
262 Aravamuthan BR, Muthusamy KA, Stein JF, et al. Topography of cortical and subcortical connections of the human pedunculopontine and subthalamic nuclei. Neuroimage. 2007;37:694-705.
263 Aravamuthan BR, Stein JF, Aziz TZ. The anatomy and localization of the pedunculopontine nucleus determined using probabilistic diffusion tractography. Br J Neurosurg. 2008;22(suppl 1):S25-32.
264 Aravamuthan BR, McNab JA, Miller KL, et al. Cortical and subcortical connections within the pedunculopontine nucleus of the primate Macaca mulatta determined using probabilistic diffusion tractography. J Clin Neurosci. 2009;16:413-420.
265 Garcia-Rill E, Houser CR, Skinner RD, et al. Locomotion-inducing sites in the vicinity of the pedunculopontine nucleus. Brain Res Bull. 1987;18:731-738.
266 Piallat B, Chabardes S, Torres N, et al. Gait is associated with an increase in tonic firing of the sub-cuneiform nucleus neurons. Neuroscience. 2009;158:1201-1205.
267 Weinberger M, Hamani C, Hutchison WD, et al. Pedunculopontine nucleus microelectrode recordings in movement disorder patients. Exp Brain Res. 2008;188:165-174.
268 Kojima J, Yamaji Y, Matsumura M, et al. Excitotoxic lesions of the pedunculopontine tegmental nucleus produce contralateral hemiparkinsonism in the monkey. Neurosci Lett. 1997;226:111-114.
269 Munro-Davies L, Winter J, Aziz TZ, et al. Kainate acid lesions of the pedunculopontine region in the normal behaving primate. Mov Disord. 2001;16:150-151.
270 Hirsch EC, Graybiel AM, Duyckaerts C, et al. Neuronal loss in the pedunculopontine tegmental nucleus in Parkinson disease and in progressive supranuclear palsy. Proc Natl Acad Sci U S A. 1987;84:5976-5980.
271 Jellinger KA. Neuropathology of movement disorders. Neurosurg Clin N Am. 1998;9:237-262.
272 Jenkinson N, Nandi D, Muthusamy K, et al. Anatomy, physiology, and pathophysiology of the pedunculopontine nucleus. Mov Disord. 2009;24:319-328.
273 Nandi D, Aziz TZ, Giladi N, et al. Reversal of akinesia in experimental parkinsonism by GABA antagonist microinjections in the pedunculopontine nucleus. Brain. 2002;125:2418-2430.
274 Jenkinson N, Nandi D, Miall RC, et al. Pedunculopontine nucleus stimulation improves akinesia in a parkinsonian monkey. Neuroreport. 2004;15:2621-2624.
275 Pierantozzi M, Palmieri MG, Galati S. Pedunculopontine nucleus deep brain stimulation changes spinal cord excitability in Parkinson’s disease patients. J Neural Transm. 2008;115:731-735.
276 Ferraye MU, Debu B, Pollak P. Deep brain stimulation effect on freezing of gait. Mov Disord. 2008;23(suppl 2):S489-494.
277 Lozano AM, Snyder BJ. Deep brain stimulation for parkinsonian gait disorders. J Neurol. 2008;255(suppl 4):30-31.
278 Nandi D, Liu X, Winter JL, et al. Deep brain stimulation of the pedunculopontine region in the normal non-human primate. J Clin Neurosci. 2002;9:170-174.
279 Nandi D, Jenkinson N, Stein J, et al. The pedunculopontinhe nucleus in Parkinson’s disease: primate studies. Br J Neurosurg. 2008;22(suppl 1):S4-8.
280 Mazzone P, Lozano A, Stanzione P, et al. Implantation of human pedunculopontine nucleus: a safe and clinically relevant target in Parkinson’s disease. Neuroreport. 2005;16:1877-1881.
281 Stefani A, Lozano AM, Peppe A, et al. Bilateral deep brain stimulation of the pedunculopontine and subthalamic nuclei in severe Parkinson’s disease. Brain. 2007;130:1596-1607.
282 Yelnik J. PPN or PPD, what is the target for deep brain stimulation in Parkinson’s disease? Brain. 2007;130:e79.
283 Zrinzo L, Zrinzo LV, Hariz M. The peripeduncular nucleus: a novel target for deep brain stimulation? Neuroreport. 2007;18:1301-1302.
284 Zrinzo L, Zrinzo LV. Surgical anatomy of the pedunculopontine and peripeduncular nuclei. Br J Neurosurg. 2008;22(suppl 1):S19-24.
285 Parent M, Levesque M, Parent A. Two types of projection neurons in the internal pallidum of primates: single-axon tracing and three-dimensional reconstruction. J Comp Neurol. 2001;439:162-175.
286 Percheron G, Francois C, Talbi B, et al. The primate motor thalamus. Brain Res Brain Res Rev. 1996;22:93-181.
287 Sidibe M, Bevan MD, Bolam JP, et al. Efferent connections of the internal globus pallidus in the squirrel monkey: I. Topography and synaptic organization of the pallidothalamic projection. J Comp Neurol. 1997;382:323-347.
288 Baron MS, Sidibe M, DeLong MR, et al. Course of motor and associative pallidothalamic projections in monkeys. J Comp Neurol. 2001;429:490-501.
289 Kuo JS, Carpenter MB. Organization of pallidothalamic projections in the rhesus monkey. J Comp Neurol. 1973;151:201-236.
290 Ilinsky IA, Tourtellotte WG, Kultas-Ilinsky K. Anatomical distinctions between the two basal ganglia afferent territories in the primate motor thalamus. Stereotact Funct Neurosurg. 1993;60:62-69.
291 Sakai ST, Stepniewska I, Qi HX, et al. Pallidal and cerebellar afferents to pre-supplementary motor area thalamocortical neurons in the owl monkey: a multiple labeling study. J Comp Neurol. 2000;417:164-180.
292 Gallay MN, Jeanmonod D, Liu J. Human pallidothalamic and cerebellothalamic tracts: anatomical basis for functional stereotactic neurosurgery. Brain Struct Funct. 2008;212:443-463.
293 Akkal D, Dum RP, Strick PL. Supplementary motor area and presupplementary motor area: targets of basal ganglia and cerebellar output. J Neurosci. 2007;27:10659-10673.
294 Schell GR, Strick PL. The origin of thalamic inputs to the arcuate premotor and supplementary motor areas. J Neurosci. 1984;4:539-560.
295 Strick PL. How do the basal ganglia and cerebellum gain access to the cortical motor areas? Behav Brain Res. 1985;18:107-123.
296 Hazrati LN, Parent A. Contralateral pallidothalamic and pallidotegmental projections in primates: an anterograde and retrograde labeling study. Brain Res. 1991;567:212-223.
297 Sidibe M, Pare JF, Smith Y. Nigral and pallidal inputs to functionally segregated thalamostriatal neurons in the centromedian/parafascicular nuclear compex in monkey. J Comp Neurol. 2002;447:286-299.
298 Shink E, Sidibe M, Smith Y. Efferent connections of the internal globus pallidus in the squirrel monkey: II. Topography and synaptic organization of pallidal efferents to the pedunculopontine nucleus. J Comp Neurol. 1997;382:348-363.
299 Moriizumi T, Hattori T. Choline acetyltransferase–immunoreactive neurons in the rat entopeduncular nucleus. Neuroscience. 1992;46:721-728.
300 Kha HT, Finkelstein DI, Pow DV, et al. Study of projections from the entopeduncular nucleus to the thalamus of the rat. J Comp Neurol. 2000;426:366-377.
301 Matsumoto M, Hikosaka O. Lateral habenula as a source of negative reward signals in dopamine neurons. Nature. 2007;447:1111-1115.
302 Matsumoto M, Hikosaka O. Negative motivational control of saccadic eye movement by the lateral habenula. Prog Brain Res. 2008;171:399-402.
303 Matsumoto M, Hikosaka O. Representation of negative motivational value in the primate lateral habenula. Nat Neurosci. 2009;12:77-84.
304 Hikosaka O, Sesack SR, Lecourtier L, et al. Habenula: crossroad between the basal ganglia and the limbic system. J Neurosci. 2008;28:11825-11829.
305 Hikosaka O. [Decision-making and learning by cortico-basal ganglia network.]. Brain Nerve. 2008;60:799-813.
306 Hong S, Hikosaka O. The globus pallidus sends reward-related signals to the lateral habenula. Neuron. 2008;60:720-729.
307 Geisler S, Trimble M. The lateral habenula: no longer neglected. CNS Spectr. 2008;13:484-489.
308 Ji H, Shepard PD. Lateral habenula stimulation inhibits rat midbrain dopamine neurons through a GABA(A) receptor–mediated mechanism. J Neurosci. 2007;27:6923-6930.
309 Klemm WR. Habenular and interpeduncularis nuclei: shared components in multiple-function networks. Med Sci Monit. 2004;10:RA261-273.
310 Lecourtier L, Kelly PH. A conductor hidden in the orchestra? Role of the habenular complex in monoamine transmission and cognition. Neurosci Biobehav Rev. 2007;31:658-672.
311 Sartorius A, Henn FA. Deep brain stimulation of the lateral habenula in treatment resistant major depression. Med Hypotheses. 2007;69:1305-1308.
312 Parent A, Mackey A, Smith Y, et al. The output organization of the substantia nigra in primate as revealed by a retrograde double labeling method. Brain Res Bull. 1983;10:527-529.
313 Kultas-Ilinsky K, Ilinsky IA. Fine structure of the magnocellular subdivision of the ventral anterior thalamic nucleus (VAmc) of Macaca mulatta: II. Organization of nigrothalamic afferents as revealed with EM autoradiography. J Comp Neurol. 1990;294:479-489.
314 François C, Tande D, Yelnik J, et al. Distribution and morphology of nigral axons projecting to the thalamus in primates. J Comp Neurol. 2002;447:249-260.
315 Middleton FA, Strick PL. Anatomical evidence for cerebellar and basal ganglia involvement in higher cognitive function. Science. 1994;266:458-461.
316 Middleton FA, Strick PL. The temporal lobe is a target of output from the basal ganglia. Proc Natl Acad Sci U S A. 1996;93:8683-8687.
317 Middleton FA, Strick PL. Basal-ganglia ‘projections’ to the prefrontal cortex of the primate. Cereb Cortex. 2002;12:926-935.
318 Deniau JM, Menetrey A, Charpier S. The lamellar organization of the rat substantia nigra pars reticulata: segregated patterns of striatal afferents and relationship to the topography of corticostriatal projections. Neuroscience. 1996;73:761-781.
319 Deniau JM, Mailly P, Maurice N, et al. The pars reticulata of the substantia nigra: a window to basal ganglia output. Prog Brain Res. 2007;160:151-172.
320 Mailly P, Charpier S, Menetrey A, et al. Three-dimensional organization of the recurrent axon collateral network of the substantia nigra pars reticulata neurons in the rat. J Neurosci. 2003;23:5247-5257.
321 Grofova I, Zhou M. Nigral innervation of cholinergic and glutamatergic cells in the rat mesopontine tegmentum: light and electron microscopic anterograde tracing and immunohistochemical studies. J Comp Neurol. 1998;395:359-379.
322 Spann BM, Grofova I. Nigropedunculopontine projection in the rat: an anterograde tracing study with Phaseolus vulgaris-leucoagglutinin (PHA-L). J Comp Neurol. 1991;311:375-388.
323 Hikosaka O. Basal ganglia mechanisms of reward-oriented eye movement. Ann N Y Acad Sci. 2007;1104:229-249.
324 Hikosaka O, Takikawa Y, Kawagoe R. Role of the basal ganglia in the control of purposive saccadic eye movements. Physiol Rev. 2000;80:953-978.
325 Sato M, Hikosaka O. Role of primate substantia nigra pars reticulata in reward-oriented saccadic eye movement. J Neurosci. 2002;22:2363-2373.
326 Coizet V, Comoli E, Westby GW, et al. Phasic activation of substantia nigra and the ventral tegmental area by chemical stimulation of the superior colliculus: an electrophysiological investigation in the rat. Eur J Neurosci. 2003;17:28-40.
327 Coizet V, Overton PG, Redgrave P. Collateralization of the tectonigral projection with other major output pathways of superior colliculus in the rat. J Comp Neurol. 2007;500:1034-1049.
328 Comoli E, Coizet V, Boyes J, et al. A direct projection from superior colliculus to substantia nigra for detecting salient visual events. Nat Neurosci. 2003;6:974-980.
329 Dommett E, Coizet V, Blaha CD, et al. How visual stimuli activate dopaminergic neurons at short latency. Science. 2005;307:1476-1479.
330 May PJ, McHaffie JG, Stanford TR, et al. Tectonigral projections in the primate: a pathway for pre-attentive sensory input to midbrain dopaminergic neurons. Eur J Neurosci. 2009;29:575-587.
331 McHaffie JG, Jiang H, May PJ, et al. A direct projection from superior colliculus to substantia nigra pars compacta in the cat. Neuroscience. 2006;138:221-234.
332 Redgrave P, Prescott TJ, Gurney K. Is the short-latency dopamine response too short to signal reward error? Trends Neurosci. 1999;22:146-151.
333 Redgrave P, Gurney K. The short-latency dopamine signal: a role in discovering novel actions? Nat Rev Neurosci. 2006;7:967-975.
334 Fiorillo CD, Newsome WT, Schultz W. The temporal precision of reward prediction in dopamine neurons. Nat Neurosci. 2008;11:966-973.
335 Redgrave P, Gurney K, Reynolds J. What is reinforced by phasic dopamine signals? Brain Res Rev. 2008;58:322-339.
336 Laurent PA. The emergence of saliency and novelty responses from reinforcement learning principles. Neural Netw. 2008;21:1493-1499.
337 Kobayashi S, Schultz W. Influence of reward delays on responses of dopamine neurons. J Neurosci. 2008;28:7837-7846.
338 Schultz W. Multiple dopamine functions at different time courses. Annu Rev Neurosci. 2007;30:259-288.
339 Schultz W. Behavioral dopamine signals. Trends Neurosci. 2007;30:203-210.
340 Schultz W, Preuschoff K, Camerer C. Explicit neural signals reflecting reward uncertainty. Philos Trans R Soc Lond B Biol Sci. 2008;363:3801-3811.
341 Mogoseanu D, Smith AD, Bolam JP. Monosynaptic innervation of trigeminal motor neurones involved in mastication by neurones of the parvicellular reticular formation. J Comp Neurol. 1993;336:53-65.
342 Mogoseanu D, Smith AD, Bolam JP. Monosynaptic innervation of facial motoneurones by neurones of the parvicellular reticular formation. Exp Brain Res. 1994;101:427-438.
343 Fay RA, Norgren R. Identification of rat brainstem multisynaptic connections to the oral motor nuclei using pseudorabies virus. III. Lingual muscle motor systems. Brain Res Rev. 1997;25:291-311.