Here, n, the number of transections, is calculated by counting the number of transections of alveoli in a field of known area, and ρ, the volumetric density of alveoli, is computed by measuring linear intercepts or point counting (9). β is the “shape coefficient,” a variable that relates the mean cross-sectional area of a solid to its volume. In case of polyhedral alveoli, β was estimated to be 1.55 by Weibel.
Timeline of Alveolarization by Morphometry
Studies using morphometric techniques to evaluate the timeline of formation of new alveoli in children were conducted three to five decades ago. Weibel (20) estimated the total number of alveoli (NA) in five subjects (including an 8-year-old boy and a 16-year-old woman) who died of nonrespiratory causes. The estimated NA in the two young people was nearly the same as that in the adults (296 × 106 as against 294 × 106). Dunnill (10) examined the lungs of ten children (birth to 8 years) who were term born and died of nonrespiratory causes and compared them with adult lungs. He found that NA increases with age rapidly at first and then gradually up to at least 8 years of age. By eight years of age the average number of alveoli (280 million) approached the average number in an adult (296 million). Davies and Reid (21) counted NA in lungs of 5 children who died of nonrespiratory causes from birth to eleven years. The alveolar count increased rapidly from birth (17.3 × 106) to three years (196 × 106) and then gradually increased to 303 × 106 at five years and 336 × 106 at eleven years.
Angus and Thurlbeck (22), in their study of forty-six subjects (14 subjects less than 19 years and 32 adults) attempted to determine the endpoint of human alveolarization by morphometry. They recognized the large scatter of NA in human lungs. They postulated that the number of alveoli per unit volume (NA/V) will not change during alveolar multiplication and then decrease rapidly when alveolarization ceases. Using this approach, they could not demonstrate a time point where alveolarization ceased. They noted that alveolar multiplication contributed more to increase in lung volume with growth than alveolar enlargement.
Hislop et al. (23) examined the lungs of twenty-nine infants from twenty-nine weeks of gestation to eighteen weeks of postnatal life. The aim of this study was to determine the early life increase in alveolar number. NA increased rapidly from about 20 million at twenty-nine weeks of gestation to 288 million at twelve weeks of age. The rate of increase was fastest in fetal life. According to this study, NA/V increased up to term and then decreased. This implies that alveolarization proceeds more rapidly than lung volume growth up to term and less rapidly after birth.
Thurlbeck (11) did the most influential study regarding human alveolarization to date. He estimated NA/V and NA in the lungs of fifty-six children (age: 6 wks–14 y) dying of nonrespiratory causes using Weibel’s technique. These morphometric measures were compared with age, body length, and body weight. Results show wide scatter in NA in different individuals of similar age. Analysis was performed after grouping individuals by age and estimating the average NA for each age range. Average NA in the two- to four-year-olds was found to be similar to that of the seven- to eight-year-olds, and therefore, he concluded that alveolarization was complete by two years of age.
Zeltner and Burri (13) examined alveolar microstructure using scanning and transmission electron microscopy in the lungs of seven children dying from nonrespiratory causes. Many alveolar septae in the seventeen-month-old infant’s lungs were immature with a double capillary layer, while those in the sixty-four-month-old showed mature septae, which were thinner and contained a single capillary layer. They postulated that microvascular maturation in alveolar septae takes place at the age of two to three years and contributed to the hypothesis that neo-alveolarization was not possible after this process was complete (14,24).
Drawbacks of the Technique of Morphometry
Practical Difficulties
As briefly alluded to in the introduction, the investigator faces a number of difficulties in attempting to count the number of alveoli in the human lung. First of all, by virtue of the elastic nature of the lung, it is prone to collapse when taken out of the thoracic cavity, and thus volumetric information is lost in lung biopsy specimens. Furthermore, unless properly fixed, a thin section of the lung distorts the architecture. To perform structural analysis of the periphery of the lung, the specimen must first be fixed by instillation of fixative through the airways or the blood vessels (2). Therefore a complete lung or lobe is necessary to prepare samples for lung structure by morphometry. This, clearly limits the availability of lung specimen to postmortem specimen.
Attempting to assess alveolar number in childhood using morphometric analysis is problematic because lung specimens from healthy childhood are difficult to acquire, both because of the inherent low mortality rate in children over five years of age and because of the high incidence of respiratory morbidity and mortality associated with available specimens. In addition, changes in law have made it increasingly difficult to access pathologic specimens for research.
Assessment of peripheral lung development ideally requires serial measures in the same lung. The reliance on autopsy specimen means that serial measures of the anatomy of the lung periphery is not possible. Alveolarization was instead studied by calculating the alveolar numbers in different individuals, which introduced the problem of interindividual variability. Also, determining factors influencing growth and development of the lung periphery has only been possible by using animal models and surrogate markers.
Technical Issues
There have been numerous technical advances in the science of morphometry since Weibel’s pioneering work (19). These advances have surmounted various technical issues in morphometry. It is beyond the scope of this chapter to explore these in detail, but the reader is referred to the review by Weibel et al. (25), the published scientific debate between experts in the field (26–30), and the official policy statement of American Thoracic Society/European Respiratory Society for quantitative assessment of lung structure (2). I have detailed here some important issues that need to be understood for the evaluation of the published work on human alveolarization.
Compiling the results of the studies exploring alveolarization in children, it is evident that there is a wide variance between studies in both the measured alveolar number and the estimated age of completion of alveolarization (Figure 13-1). The preparation of pathologic specimen is markedly different in different studies. For example, Davies et al. (21) inflated lungs with buffered formalin at 75 cm water pressure, Angus and Thurlbeck (11,22) used inflation pressures of 25 cm water, while Weibel (20)used formalin steam instilled at pressures of 5–10 cm water while the lung was kept inflated by negative pressure. It follows that the degree of alveolar inflation will be different between studies. Despite this, several authors (10,11) have used Weibel’s value of 1.55 for the shape coefficient, β (see earlier), despite β being dependent on the relation between surface area and volume.
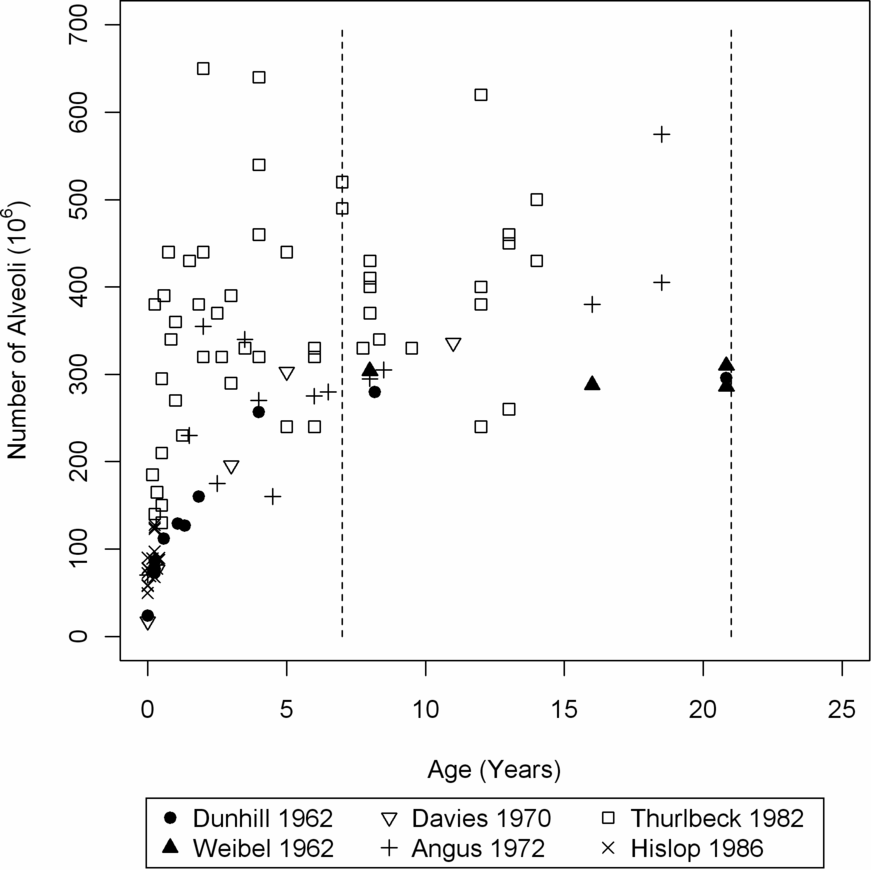
Number of alveoli in the developing human lung estimated by morphometry from previously published studies. References: Dunnill 1962(10), Weibel 1962(9), Davies 1970(21), Angus 1972(22), Thurlbeck 1982(12), Hislop 1986(23).
Another limitation of the studies using morphometric techniques to determine endpoint of alveolarization is that the same inflation pressure was used to inflate lungs of children in different age groups. It has been noted that the range of lung volumes at which fixation occurs is between 50 and 70% of total lung capacity, with the actual value set by individually varying compliance of the thorax (31). This may change the relative degree of inflation of alveoli between the subjects, with a strong likelihood of bias (it is likely that compliance varies with age). The pronounced intersubject scatter (11,22) may also be partially explained by this phenomenon. The assumptions that the shape coefficient, β, and the distribution coefficient of alveoli (i.e., a coefficient relating to standard deviation of the size of individual alveoli) do not vary with age may also be flawed and may lead to a variability of about 20% and 10%, respectively in the results (11).
Measurements by classical morphometry were done on a very small sample (between 1:100000 to 1:1000000) of the alveoli (27). It is essential, in these circumstances, to ensure randomization of sampling. This has been achieved with newer morphometric techniques [e.g., design-based stereology as described by Hyde et al. (32,33)]. Unfortunately, the newer techniques (2) have not been used to determine the endpoint of alveolarization in humans.
New Histological Techniques to Determine Alveolar Number
Techniques that surmount the pitfalls mentioned have been developed following the development of the science of stereology and the description of the “disector” by Sterio (34). It is not the remit of this chapter to discuss this technique in detail, but interested readers are referred to excellent reviews on the subject (25,35,36). In brief, the process of counting the number of particles in a specimen starts by successively dividing the fixed specimen into blocks, from which a random number of blocks are selected. The selected blocks are then oriented in a random manner before subdivision into subblocks. A random fraction of these subblocks is then selected, and the process is repeated until sections that can be assessed under a microscope can be prepared. At this stage, random sets of two contiguous sections are assessed, viz., the “sampled section” and the “look-up section” (36). The number of particles that appear in the sampled section, but not the look-up section is calculated, and the number is then summed over all the sampled sections (Q). The number of particles in the whole specimen can then be calculated from Q and the fraction of the specimen assessed under the microscope. In case of counting alveoli in lung specimen, the fact that each alveoli have a single distinct opening is utilized in determining the so-called Euler characteristic of the network of alveolar openings in the periphery of the lung (37,38). It is clear that this technique does not require any assumption of shape or orientation of the alveoli and does not depend on the degree of inflation of the lung (25).
Nonhistologic Techniques to Determine Alveolar Dimensions
3Helium Magnetic Resonance
Over the last decade, tremendous progress has been made to develop safe, noninvasive markers of alveolar structure and/or dimension. One of the main developments is the technique of 3HeMR, which measures the self-diffusion of hyperpolarized 3Helium (3He) within the lung during a breath hold using magnetic resonance (MR). A simplified description of the technique is given in the following paragraph. However, readers are referred to the following resources for detailed explanation of the technique (17,18,39,40).
Magnetic resonance (MR) is based on the interaction of atomic nuclei with an unpaired spin (1Hydrogen in conventional MR; 3Helium and 129Xenon in hyperpolarized gas MR) with an external magnetic field (41). The technique utilizes a predetermined “sequence” of radiofrequency pulses to interact with these nuclei. As a result of this interaction, these nuclei emit radiofrequency pulses, which are measured as the “signal”. In diffusion weighted MR, the sequence employed “sensitizes” the nuclei to movement. In other words, the measured signal decreases with the degree of displacement of the nucleus from its original position. The diffusion coefficient of the nucleus (D) can be calculated from the signal. In the case of 3HeMR applied to human lungs, the MR signal is measured during a breath hold after inhalation of a bolus of 3He. The diffusion of 3He in this situation is constrained by alveolar walls, as alveoli are impermeable to the 3He (Figure 13–2). Therefore, the measured value of diffusion of these nuclei, the apparent diffusion coefficient (ADC), is a function of the degree of restriction of diffusion, which in turn depends on the size of the alveoli.
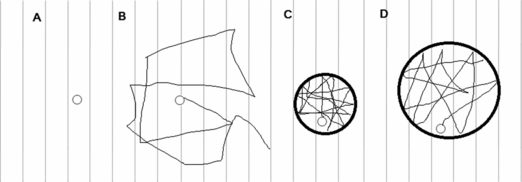
Panel A. Hypothetical stationary molecule – Diffusion coefficient D = 0. Panel B. Freely diffusing 3He molecule in air, D (free diffusion coefficient) = 0.88 cm2.sec-1. Panel C and D. Diffusion restricted by constraints, that is, alveolar walls in case of inhaled helium. The measured (apparent) diffusion coefficient (ADC) is higher in panel D than panel C and can be used as a marker of alveolar dimension. This representation is a simplification, as in reality, alveoli are not completely spherical, and they are open to alveolar ducts. However, as long as the majority of the constraints on diffusion of 3He are alveolar walls, ADC would represent alveolar dimensions.
Since the initial description of this technique, many studies have been performed in adults, confirming its utility to detect alveolar damage in emphysema (18,42,43) and in asymptomatic smokers (44). Validation of ADC against histologic parameters has been carried out in elastase-induced emphysema in rats (45,46) and in rabbits (47) and in explanted COPD affected human lungs (comparing with lungs of donors that were unsuitable for transplant) (48).
Further developments in this field include calculating length scales from the diffusion coefficients of 3He utilizing differences in the measured signal due to a number of gradient pulses, each of which sensitize 3He to different degrees of diffusion. Yablonskiy et al. (49,50) used this technique of 3HeMR and a simplified mathematical model of cylindrical alveolar ducts surrounded by a uniform sleeve of alveoli to estimate the acinar airway diameter (R) and effective alveolar depth (h). These values were validated against histological methods in rats (51) and human lungs (50). Shanbhag described measurements of average displacement (xrms) of the 3He nuclei using a diffusion probability profile (DPP) calculated from the technique of diffusion-weighted spectroscopy and q-space analysis (52). Two components of these measurements corresponded to diameter of the alveoli and length of airspaces, respectively.
Aerosol-Derived Airway Morphometry (ADAM)
Heyder (53) described a technique of estimating the size of airways using measurement of gravitational deposition of aerosols in airways during a breath-hold following inhalation of boluses of aerosols. This measurement was based on the principle that settling velocity of the aerosol was inversely related to the caliber of the airway in still air (54). Therefore, airway caliber could be estimated (effective airway diameter, EAD) from recovery of aerosol particles on exhalation after a timed breath hold (54,55). The smallest EAD, EADmin, corresponding to the most peripheral zone of the lung, was found to be a reliable estimate of the size of the most distal airspace generation, that is, alveoli (55). Some variables derived from ADAM have been shown to roughly correlate with histologic measures in human and dog lung specimens (56,57).
Relative Merits and Demerits of the Noninvasive Techniques
Both of these noninvasive techniques have the potential to be applied for research into alveolarization as they do not involve ionizing radiation and are free from other similar ethical concerns. Both these techniques dispense with the problem of sampling, as they effectively sample the whole lung. The respiratory maneuver required for 3HeMR is easy, as it involves just a short breath hold of three to ten seconds after inhaling the bolus of 3He from functional residual capacity (FRC). In contrast, ADAM involves breathing in the aerosol to total lung capacity (TLC) followed by a timed breath-hold and exhalation at a constant exhalation velocity.
ADAM measures EADmin, which is suggested to be equivalent to the alveolar diameter. This may be true if the lung is comprised of a perfectly symmetric branching tree, but the airway branching both at conducting level and at acinar level is nonsymmetric, both in terms of angles and relative cross-sectional areas of the daughter segments (58–60). Also, a review of physical principles behind gas mixing and aerosol mixing suggests that aerosol deposition depends on factors other than size of the airspaces (61). These factors are themselves dependent on developmental changes, including increased depth of alveoli and changes in relative flow (61). Therefore, ADAM may not be a suitable tool to evaluate development of alveoli in childhood and adolescence.
The technique of 3HeMR relies on far fewer assumptions. The extremely high diffusivity of 3He atoms minimizes problems with nonuniform mixing. Measurements from 3HeMR have been well validated against histologic measures in both animal and human lungs (see earlier discussion). However, it must be remembered that ADC is not a measure of length but of physical restriction to diffusion. Many calculations of physical dimensions from diffusion restriction of 3He depend on geometric models and are therefore reliant on the validity of those assumptions (50). xrms does not depend on geometric assumptions (52), but gives a mean displacement of 3He atoms, which can only be approximated to physical dimensions of alveoli.
Another concept that can affect the interpretation of ADC is “diffusion time”. This is the time available for the 3He atoms to diffuse in the physically restricted environment of the peripheral lung. If the diffusion time is too short, the diffusion is not restricted, and ADC approximates D, the free diffusion of 3He atoms in air. If the diffusion time is too long, the helium atoms undergo minimal net displacement, and ADC tends to zero. Between these extremes (diffusion times of the order of a few milliseconds) the ADC is able to give information about dimensions of alveoli (62). The value of ADC depends on the selection of diffusion time and other factors such as the strength of the external magnetic field and the sequence and magnitude of the gradients employed. Therefore, a control group is necessary in most studies using 3HeMR. As long as the limitations are recognized, 3HeMR is a potent tool to explore the question of alveolarization in humans.
Early Studies of Alveolar Development Using 3HeMR and ADAM
Using 3HeMR, Altes et al. (63) measured ADC in twenty-nine healthy subjects ranging from four to thirty years. They reported an increase of ADC with age, which suggests increase in alveolar dimensions with age. Estimation of an expected increase in ADC in the absence of alveolarization was not attempted, and therefore this study did not fully address the question about age of completion of alveolarization.
Zeman et al. (64) used ADAM to determine EADmin at TLC in fifty-three children and young adults age six to twenty-two years and fifty-nine adults. EADmin increased with age, and TLC varied as the third power of EADmin. They postulated that between ages of six and twenty-two, alveoli do not increase in number but expand to cause lung growth. However, the relation of TLC with EADmin was determined after combining measurements of children and adults. There are also a few reservations with use of this technique to determine alveolar development (see paragraph on relative merits and demerits).
Indirect Measures of Alveolar Dimensions
Measurements of diffusing capacity of carbon monoxide (DLCO), alveolar volume (VA) and transfer coefficient (KCO = DLCO/VA) are well known physiological measures of alveolar–capillary function. The technique and principles behind these measures are well described in several textbooks and are not elaborated here (65). Some authors have used these measures as surrogates of alveolar growth (66–68). These measures, however, could be affected by hemoglobin levels, permeability of the alveolar–capillary barrier, ventilation–perfusion mismatch, and differences in pulmonary perfusion (67) and therefore should be interpreted with caution in the context of alveolar development.
Another indirect estimate of average airspace dimension depends on the finding that pressure–volume curves during passive lung deflation were related to the mean size of peripheral airspaces (69). An index of pulmonary distensibility derived from the pressure–volume curves has been shown to be related to morphometrically derived mean linear intercept (70,71). The recoil of thoracic wall has not been taken into account in the derivation, and therefore it is difficult to extrapolate this relationship to measurements in live humans. Also, it is likely that relative contributions to the P–V characteristics may change with age, and therefore, this technique has not been used to evaluate alveolar development.
Alveolarization Until Maturity in Animals
Many recent studies conducted in animals have suggested that new alveoli may continue to form throughout the period of lung growth and even in adulthood. Many of these utilize the new histologic technique mentioned earlier.
Alveolarization Following Pneumonectomy in Adult Animals
Hsia et al. (72) examined peripheral lung structure in the left lungs of five dogs using morphometric techniques five months and sixteen months following right pneumonectomy. Alveolar surface density progressively increases between five and sixteen months postpneumonectomy to reach values approximating the surface density in control dogs. This adaptive response was postulated to be due to initial expansion of alveolar airspaces to fill the thoracic cavity following pneumonectomy followed by septation of enlarged airspaces (i.e. formation of new alveoli). Fehrenbach et al. (73) used the new technique of design-based stereology to assess compensatory lung growth following left pneumonectomy in adult mice. They performed left pneumonectomy in eleven adult mice and determined alveolar numbers in the right lung at day 6 and day 20 postpneumonectomy. By day 20, the right lung had gained 49% of the total alveoli lost due to removal of the left lung. In the unique situation of pneumonectomy, the residual lung can “regrow” alveoli.
Alveolarization Through the Period of Growth in Rabbits and Rhesus Monkeys
Kovar et al. (16) determined alveolar number by Weibel’s morphometric methods in rabbits at various ages from birth to thirty-six weeks of life (adulthood). They found that the alveolar number increases progressively from birth to adulthood, though the rate of new alveolarization decreases with age. Hyde et al. (15) examined the lungs of twenty-six rhesus monkeys at various ages from 4 days to 7.6 years of life (i.e., neonatal period to adulthood: somatic growth complete by about six years) using design-based stereology. The number of alveoli increased significantly with age, throughout the period of somatic growth. Rhesus monkeys are more plausible as models for cessation of alveolarization in humans than rabbits.
Calorie-Related Changes in Alveolar Number
Karlinsky et al. (74) restricted calorie intake by 50% in a group of hamsters for a period of thirty days and compared morphometric data with control hamsters. They found increased mean linear intercept and decreased lung internal surface area in the starved hamsters, suggesting destruction of alveoli related to calorie restriction. Massaro et al. (75) restricted calorie intake in adult mice by 67%. They estimated alveolar number and alveolar dimensions in the calorie-restricted mice using newer histologic techniques (76) and compared alveoli with controls. Alveolar volume increased by 44% within seventy-two hours of calorie restriction. They allowed another group of mice to feed ad-libitum after fifteen days of calorie restriction. Histologic analysis just seventy-two hours after adlibitum feeding showed that alveolar dimensions and number were restored to values prior to calorie restriction. The molecular mechanisms related to these changes were investigated, and the pathway seemed to be associated with mediators associated with apoptosis. A surprising degree of alveolar plasticity in adult mice was revealed – new alveoli could be formed or destroyed based on caloric intake.
Possible Mechanisms of Postmaturity Alveolarization
Schittny et al. (77) determined the progress of alveolarization in Sprague-Dawley rats from four days of life to sixty days (adulthood in these rats) using design-based stereology techniques. They found that new alveolar septae were being formed well into adulthood. Using 3-D synchrotron radiation X-ray tomography, they showed local duplication of single capillary layers in areas of postmaturity septal growth, which indicated a potential mechanism for postmature alveolarization. These studies dispelled the notion that the immature double capillary layers in alveolar walls were a prerequisite for new septation (14,24). Taken together, this information from mammals supports alveolarization beyond early childhood. There is no reason why this could not happen in humans. In their paper, Hyde et al. (15) have called for reconsideration of previous reports of postnatal alveolar development in humans because the previous reports were based on bias-prone techniques. Unfortunately, there have not been any studies on alveolar development in humans using new stereologic techniques. However, recent studies based on noninvasive techniques suggest that new alveoli may continue to form through the period of lung growth in humans.
Alveolarization Until Maturity in Humans
Noninvasive Measurements of Alveolar Dimension During Normal Lung Growth
Narayanan et al. (78) determined ADC and xrms as surrogates of alveolar dimensions using two techniques of 3HeMR in 109 healthy subjects between seven and twenty-one years. Measurements were also done with different degrees of gentle inflation of the lung in selected subjects. Lung volumes were measured by plethysmography in all subjects. Changes of the 3HeMR measures during lung growth were compared to changes with lung inflation. Lung inflation was used as a model for lung enlargement without alveolarization, and the comparisons were carried out based on statistical methods. Both variables, ADC and xrms, increased with lung growth, but at a rate significantly less than the expected increase in the absence of new alveolarization. Based on the statistical model, Narayanan et al. estimated that the observed 3.4-fold increase in FRC between seven and twenty-one years of age was accompanied by a 1.94-fold (95% CI, 1.64–2.30) increase in alveolar number and a 1.75-fold (95% CI, 1.48–2.07) increase in alveolar volume (78). This was the first direct evidence regarding alveolarization to adulthood in humans.
Alveolarization Following Pneumonectomy in Adult Human
Butler et al. (79) assessed measurements derived from 3HeMR in an adult woman fifteen years after undergoing right pneumonectomy for hilar adenocarcinoma at the age of thirty-three years. The radial dimensions of acinar airways (R) calculated by applying Yablonskiy’s model (50) on the 3HeMR measurements were found to be close to normal (330±20 μm ) as against the expected value of ~390 μm, implying an increase in the number of alveoli. They calculate that there was a 64% increase in the number of alveoli following pneumonectomy.
Recovery of Alveolar Structure Following Preterm Birth
3HeMR is an ideal tool to study recovery of lung alveoli following injury. Extreme preterm birth and neonatal chronic lung disease (CLD) are important factors that affect alveolar development. Many studies (80–83) have used histologic techniques to determine peripheral lung structure on lung specimens from children who have died of CLD. Apart from one child who survived up to 7.75 years in Husain’s series, all the other human histologic data regarding the lung structure in extreme preterm survivors are from infants who died before forty months of age. Overall, these studies suggest that in children born extremely preterm, disordered peripheral lung development and, consequently, deranged alveolar structure persists until at least three years of age. When considered with the previous concept that human alveolarization was complete by three years, it was assumed that deranged alveolar structure would be a lifelong feature in preterm survivors (84,85).
This reasoning could be challenged for two reasons. First, histologic data are necessarily limited to the fatal, severe cases of preterm CLD and therefore cannot be generalized to survivors. The second challenge is to the assumption that human alveolarization is complete at three years. Animal models were developed to answer the first challenge. The best known of the animal models was the preterm baboon model developed by Dr. Coalson’s team (84,86,87). Despite this pioneering work, there were no data on long-term survivors because the maximum survival reported in the preterm baboons was eight months – a human developmental equivalent of three years. Regarding the second challenge, the assumption has come under increasing scrutiny based on the results with animal models and humans. Narayanan et al. (88) published the first direct proof that alveolar damage sustained due to preterm birth may not be lifelong. They compared measurements related to alveolar dimensions using 3HeMR in 119 children from ten to fourteen years stratified into four groups (term born, mild preterm, extreme preterm without CLD, and extreme preterm with CLD). ADC was similar in all four groups and was not related to risk factors for CLD, which implied that any derangement in alveolar development due to extreme preterm birth or CLD could be compensated for within the first decade of life in survivors. The intrasubject spread of ADC (SDADC) was larger in the CLD group, suggesting some subtle residual damage (89). This study had adequate statistical power to detect even small differences in alveolar dimensions in the preterm group. These results strongly support the novel notion that human alveoli have a capacity to regenerate far beyond early childhood.
The Physiologic Rationale for Continued Alveolar Growth
Over the last decade, the possibility that at least some alveolarization occurs beyond early childhood was recognized by authors including Burri (90) and Massaro (91). This concept is supported by many indirect findings from other studies, not necessarily evaluating alveolarization. The findings from animal studies and 3HeMR discussed earlier reinforce the hypothesis of alveolar development through the period of lung growth. The other indirect findings that support this hypothesis of continued alveolar growth follow.
Metabolic Demand and “the Call for Oxygen”
Tenney et al. (92) measured total alveolar surface area and alveolar diameter in twenty-six representative mammals of diverse body size and metabolic rates. They found that the internal surface area of the lung correlates very well with resting oxygen consumption. Notably, alveolar diameter was found to negatively correlate with body weight-specific oxygen consumption. For example, mammals with high metabolic activity like shrews and bats had the smallest alveoli, and the relatively sluggish mammals like dugong and manatee had the largest alveoli. The relationship was explained by Massaro on the basis of the “call for oxygen” (91). Oxygen delivery can be affected by tidal volume and respiratory rate, but there are physiological limitations to these variables (92).
Sapoval (93) predicted on theoretical considerations that an ideal acinar structure and dimension must exist for ideal gas exchange. It is probable that an ideal alveolar size exists as well, related to metabolic demand. This would make sense from the physical standpoint because oxygen molecules are more likely to encounter alveolar walls during Brownian motion if alveolar walls are closer together (in a smaller alveolus). It is also likely that this ideal dimension is also sensitive to interindividual variations in metabolic demand.
Using design-based stereology in adult human lungs, Ochs et al. (38) showed that alveolar number was closely related to adult lung volume and that mean alveolar size was almost constant between subjects. If alveolarization were completed by two to three years of age, the final number of alveoli, and by extrapolation, the final size of the lung and the final metabolic demand, would have to be set by then, which is implausible.
Alveolar Plasticity
There is evidence from both animal and human studies that alveolar dimensions (and number) may be related to metabolic needs. Massaro et al. (75,94) and Karlinsky et al. (74) showed calorie-related changes in alveolar number in adult mammals. A similar phenomenon has been reported in humans. Coxson (95) compared computed tomographic (CT) images of twenty-one young adults with anorexia nervosa with images of sixteen age-matched controls (all females). CT measures of attenuation confirmed that the adults with anorexia nervosa had changes similar to emphysematous lungs. Massaro (96) and Coxson (95) hypothesized that these changes were an adaptive response to diminished consumption of oxygen during periods of starvation. Alveolar regeneration after refeeding supports the theory that certain mechanisms must exist for alveolarization to proceed beyond early childhood. Both histologic studies (97) and 3HeMR studies (98) indicate that alveoli tend to become larger with age in adulthood. This is probably related to declining metabolic needs. Conversely, in a physiological study of elite swimmers, Armour et al. (99) noted increased lung volumes (including vital capacity, total lung capacity, and functional residual capacity) and higher diffusing capacity of carbon monoxide (DLCO) but unchanged diffusion coefficient (KCO = DLCO/Alveolar volume) and index of pulmonary distensibility compared to controls. Within the limits of the technique, the findings support increased alveolar number in elite swimmers.
Survivors of Preterm Birth
Evidence from 3HeMR shows that alveolar dimensions in children born very preterm and in survivors of neonatal chronic lung disease are essentially identical to term born children (88). This indicates catch-up of alveolar structure following preterm birth. However, functional respiratory studies in preterm survivors show that preterm infants have lower DLCO at school age (66) and young adulthood (67). DLCO is, however, a test of alveolar–capillary function and is influenced by the permeability of the alveolar–capillary barrier and ventilation–perfusion mismatch, apart from the alveolar surface area (see earlier). Therefore, DLCO can remain abnormal even if alveolar structure has normalized in preterm survivors. This dichotomy between alveolar structure and function is more likely to happen in preterm survivors than in diseases of mature alveolar–capillary units such as COPD. Narang et al. (100) showed that though DLCO is decreased at rest in ex-preterm subjects studied at twenty-one years of age, it normalizes with exercise. Such an improvement with dynamic testing is not plausible with persistent structural damage to alveoli. This again supports the notion that alveolar structure can recover following preterm birth.
Conclusions and Future Developments
To conclude, it is clear that emerging evidence with newer techniques of measurement of alveoli (both invasive and noninvasive) and other indirect techniques support the theory that new alveoli continue to form through the period of lung growth. It is time for this new paradigm of alveolarization to be disseminated. However, the gold standard “proof” will require replicating histologic studies in normal human lungs using newer stereologic techniques through the period of lung growth. In the absence of such a gold standard study, future studies may utilize the noninvasive nature of 3HeMR to conduct longitudinal studies of alveolar dimensions. The theory may also be strengthened if the results described earlier are replicated using measurements of 3HeMR in growing lungs using various diffusion times. The noninvasive nature of 3HeMR may also be utilized to test alveolar plasticity by measuring alveolar dimensions before and after influences such as exposure to environmental tobacco smoke or calorie restriction. Measurements from 3HeMR have potential to be used as noninvasive endpoints if new alveolar therapies are developed in the future. The availability of these new measurement techniques demonstrates the continued growth potential of the healthy alveoli. The critical questions are to determine the mechanisms by which alveolar numbers are regulated and whether knowledge of those mechanisms can be translated to therapies.