Chapter 33 Acute Renal Failure and Renal Support
Renal dysfunction is relatively common following cardiac surgery and is associated with a substantial increase in mortality rates. The incidence of postoperative renal dysfunction (PRD) varies according to the way in which it is defined and the population being studied. In unselected groups of cardiac surgery patients with PRD, mortality rates of 3.4% to 17% have been reported.1–4 The need for renal replacement therapy occurs in 1% to 4% of cases and is associated with mortality rates of 30% to 70%.1,2,4–7
In this chapter, the risk factors, diagnosis, and treatment of postoperative acute renal failure are discussed. Traditionally, renal replacement therapy for acute renal failure in cardiac surgery patients has involved peritoneal dialysis and intermittent hemodialysis. However, over the past decade, continuous and semicontinuous therapies that are better suited to the critically ill have become common. The indications, various types, and practicalities of these techniques are discussed. The physiology of normal and abnormal renal function is reviewed in Chapters 1 & 31.
ASSESSMENT OF RENAL FUNCTION
Tests of renal function evaluate either glomerular filtration rate (GFR) or tubular function. Severe renal hypoperfusion results in a reduction in the GFR and an increase in the plasma concentration of nitrogenous wastes (urea and creatinine). This is known as prerenal azotemia. When renal hypoperfusion is severe and sustained, acute tubular necrosis can occur, and it results in abnormal tubular function. The pathophysiologic mechanisms of prerenal azotemia and acute tubular necrosis are described in Chapter 1.
Glomerular Filtration
The two most widely used biochemical tests of GFR are plasma creatinine and blood urea nitrogen (BUN). The normal plasma creatinine concentration is 50 to 120 μmol/l (0.6 to 1.4 mg/dl) in males and 40 to 100 μmol/l (0.5 to 1.1 mg/dl) in females. The normal BUN concentration is 3.2 to 7.7 mmol/l (9 to 22 mg/dl). Of the two, creatinine is the more reflective of the GFR. Creatinine is released from muscle at a relatively constant rate, is freely filtered at the glomerulus and, unlike urea, is not reabsorbed in the tubules. Creatinine concentration is relatively constant despite changes in diet and metabolic rate. As people age, there is a gradual reduction in the GFR, which is matched by a decline in muscle mass. Thus, creatinine concentration remains relatively constant. The relationship between the creatinine concentration and the GFR is not linear (Fig. 33-1); with normal renal function, a small rise in creatinine concentration signals a large deterioration in the GFR; with severe renal dysfunction, large changes in creatinine reflect small changes in the GFR.
A more accurate estimate of the GFR, which is independent of age, is creatinine clearance. The normal range for creatinine clearance is 120 ± 25 ml/min for men and 95 ± 20 ml/min for women.8 Creatinine clearance (CCr) can be calculated on the basis of the plasma (PCr) and urinary (UCr) concentrations of creatinine and the urine volume (V):
This requires 24-hour urine collection. A simpler method is to estimate creatinine clearance on the basis of the following empiric formula:
Tubular Function
Tubular dysfunction results in the inability to generate appropriately concentrated urine in response to a physiologic stimulus. With prerenal azotemia, tubular function is intact. In this situation, high levels of aldosterone and antidiuretic hormone lead to maximal tubular reabsorption of sodium and the excretion of low-volume, hypertonic urine. Urine osmolarity is typically greater than 500 mOsm/l and urinary sodium concentration less than 20 mmol/l. An exception to these findings is seen in patients treated with diuretics and in the very elderly, who have a higher sodium concentration and a lower osmolarity. With acute tubular necrosis, there is loss of the normal hypertonicity of the medulla (see Chapter 1) and the inability to conserve sodium and excrete concentrated urine. In this circumstance, urine osmolarity is typically less than 400 mOsm/l, and urinary sodium concentration is greater than 20 mmol/l.
Urine Output
Oliguria (urine output <0.5 ml/kg/hr) is an important marker of renal hypoperfusion and evolving acute tubular necrosis. However, urine output must be interpreted with caution. With a normal diet, about 600 mOsm/day of solute waste products generated by metabolism must be excreted in the urine. With normal renal tubular function and maximal antidiuretic hormone stimulation, this requires a minimum of 500 ml/day of urine. In postoperative patients who have low dietary intake and high plasma levels of catecholamines, aldosterone, and antidiuretic hormone (see Chapter 32), oliguria may be a normal finding and not indicative of a reduced GFR or tubular dysfunction. Oliguria in association with normal plasma creatinine concentration and normal intravascular volume does not require treatment. However, oliguria in association with prerenal azotemia or acute tubular necrosis mandates a search for the cause of renal hypoperfusion, in particular, a careful assessment of intravascular volume status and cardiac function. Conversely, high urine output can occur despite intravascular volume depletion. Polyuria is common in the first few hours following cardiac surgery as a consequence of hypothermic cardiopulmonary bypass (CPB) and the administration of diuretics—particularly mannitol, which may be used in the CPB prime solution.
Oliguria is usually present with prerenal azotemia in the early period of acute tubular necrosis. If tubular necrosis is severe, anuria develops because of a low or absent GFR and tubular obstruction (see Chapter 1). If the GFR is then partially or fully restored, polyuria often develops due to the inability to concentrate urine.
Renal Imaging
Ultrasound scanning of the kidneys and renal tract is the most useful imaging technique in patients with acute renal dysfunction, and it can be performed at the bedside. Ultrasonography can identify obstruction in the upper or lower urinary tract and can confirm the presence and size of each kidney. Small (or large polycystic) kidneys indicate chronic renal disease. Doppler flow imaging may suggest the presence of atherosclerotic renal artery stenosis or, occasionally, renal vein thrombosis.
ACUTE RENAL FAILURE
Definition
Various definitions of renal dysfunction are used; they are based on the plasma creatinine concentration, the percentage of rise in creatinine concentration, or the need for renal replacement therapy. Recently, the RIFLE (risk, injury, failure, loss, and end-stage kidney disease) classification has been proposed by the Acute Dialysis Quality Initiative Workgroup as a consensus definition of acute renal failure in critically ill patients.9 Renal dysfunction is divided into three categories (risk, injury, failure) on the basis of plasma creatinine concentration and urine output. In addition, there are two levels of outcome (loss, end-stage kidney disease), determined by the persistence of renal failure at 4 weeks or 3 months (Table 33-1). The RIFLE classification has recently been evaluated in cardiac surgery patients and has been shown to be an independent predictor of mortality: patients categorized as having risk, injury, or failure postoperatively had 90-day mortality rates of 8%, 22%, and 33%, respectively.10
Table 33-1 RIFLE Classification of Renal Dysfunction and Failure
Classification | GFR Criteria | Urine Output Criteria |
---|---|---|
Risk | Increase in creatinine by 50% | Urine volume <0.5 ml/kg/hr for 6 hr |
Injury | Increase in creatinine by 100% | Urine volume <0.5 ml/kg/hr for 12 hr |
Failure | Increase in creatinine by 200% to more than 4 mg/dl (354 μmol/l), with a rise ≥0.5 mg/dl (44μmol/l | Urine volume <0.3 ml/kg/hr for 24 hr or anuria for 12 hr |
Loss | Persistent acute renal failure or complete loss of renal function for >4 weeks | |
End-stage kidney disease | End-stage kidney disease (>3 months) |
GFR, glomerular filtration rate
From Bellomo R, Ronco C, Kellum JA, et al: Acute renal failure—definition, outcome measures, animal models, fluid therapy and i nformation technology needs: the Second International Consensus Conference of the Acute Dialysis Quality Initiative (ADQI) Group. Crit Care 8:R204-R212, 2004.
Risk Factors for Acute Renal Failure Following Cardiac Surgery
The causes of renal failure following cardiac surgery are multifactorial. Renal hypoperfusion may occur because of periods of hypovolemia, hypotension, or low cardiac output. Nonpulsatile CPB leads to reduced renal blood flow.11,12 Renal function may be adversely affected by the systemic inflammatory response to surgery and CPB and by free plasma hemoglobin generated by bypass-induced trauma to red blood cells. Atheroembolism may occur due to aortic manipulations such as cross-clamping, cannulation, and the use of an intraaortic balloon pump. Nephrotoxic drugs—in particular radiocontrast agents used for coronary angiography—may be administered during the perioperative period.
The risk factors for renal failure requiring renal replacement therapy following cardiac surgery are listed in Table 33-2. Of these, preexisting renal dysfunction is by far the most important; it is a major predictor of postoperative mortality.13
Table 33-2 Factors That are Independently Associated with the Need for Postoperative Renal Replacement Therapy Following Cardiac Surgery
Requirement for blood transfusion |
Emergency operation |
Preoperative renal dysfunction (creatinine >124 μmol/l or > 1.4 mg/dl) |
Requirement for re-sternotomy |
Mitral valve surgery |
Low ejection fraction (< 40%) |
Use of an intraaortic balloon pump |
Prolonged cardiopulmonary bypass time |
From Bove T, Calabro MG, Landoni G, et al: The incidence and risk of acute renal failure after cardiac surgery. J Cardiothorac Vasc Anesth 18:442-445, 2004.
Causes of chronic renal dysfunction include hypertension, diabetes, connective tissue disease, chronic use of nonsteroidal antiinflammatory drugs, glomerulonephritis, and polycystic kidney disease. Chronic renal dysfunction is very common in patients presenting for cardiac surgery. In one large series, 51% of patients had mild renal dysfunction (GFR 60 to 90 ml/min); 24% had moderate renal dysfunction (GFR 30 to 59 ml/min); 2% had severe renal dysfunction (GFR <30 ml/min); and 1.5% were dialysis dependent.13
Nephrotoxic Drugs
Drugs may cause renal injury by impairing renal blood flow or by causing tubular damage. Drugs such as nonsteroidal antiinflammatory drugs (NSAIDS) adversely affect vascular tone in the glomerular arterioles and can therefore reduce renal blood flow and the GFR. These drugs should be avoided when renal blood flow is reduced (e.g., in hypovolemia and low cardiac output) or fixed (e.g., in bilateral renal artery stenosis). Similarly, the use of vasopressors in the presence of hypovolemia can lead to reduced renal blood flow (see subsequent material). By virtue of their ability to cause intravascular volume depletion, diuretics are also potentially nephrotoxic.
Aminoglycosides, amphotericin and, to a lesser extent, vancomycin can cause tubular injury. In the case of amphotericin, the lipophilic formulation is associated with reduced tubular injury and should be used when there is renal dysfunction.14
Cyclosporin-induced renal dysfunction is characterized by an obliterative vasculopathy of the afferent arteriole and tubulointerstitial fibrosis.15 These changes (which take weeks or months to develop) are believed to be caused by an alteration in the release of vasoactive substances, such as angiotensin II, endothelin, prostaglandins, and nitric oxide, as well as by the stimulation of certain proliferative cell genes.15 Contrast nephropathy is discussed subsequently.
Physiologic Consequences of Renal Failure
Acidosis and Hyperkalemia
In severe renal dysfunction, an elevated anion-gap metabolic acidosis develops due to the inability of the kidneys to excrete the acid load generated by metabolism (see Chapter 31). In chronic renal failure, metabolic acidosis becomes apparent when the GFR falls to about 25% of normal, but in acute tubular necrosis, acidosis is an early finding.
Potassium undergoes glomerular filtration and tubular secretion (see Chapter 32). Hyperkalemia can develop when renal blood flow is low—independent of acute tubular necrosis—as the result of reduced blood flow in the peritubular capillaries. Thus, hyperkalemia is closely linked to oliguria and is uncommon in polyuric renal failure.
Water and Sodium Excess
Oliguric renal failure is associated with water and sodium excess, which can cause pulmonary congestion and systemic edema and can contribute to hypertension. Water is usually ingested in excess of sodium, so hyponatremia is common (see Chapter 32).
NONDIALYTIC MANAGEMENT OF ACUTE RENAL FAILURE
Monitoring Renal Function
In all patients with acute renal dysfunction, a urinary catheter should be inserted. Fluid input and output should be recorded hourly. Acid-base, sodium, potassium, creatinine, BUN, and hemoglobin levels should be measured at least daily. Potassium should be measured much more frequently (e.g., every 1 to 4 hours) in acutely oliguric patients. Measurements of urinary sodium concentration and osmolarity are also useful. Careful monitoring of cardiorespiratory function is indicated. Intraabdominal pressure monitoring may also be indicated.
Preventing Further Renal Injury
Renal Tract Obstruction
Occasionally, raised intraabdominal pressure due to abdominal pathology contributes to renal (and respiratory and splanchnic) dysfunction. If this is suspected, intravesicular (bladder) pressure, which is a surrogate for intraabdominal pressure, should be measured. An intravesicular pressure greater than 24 cm H2O is associated with the development of postoperative renal failure.16 If intraabdominal hypertension is severe (intravesicular pressure >30 to 40 cm H2O) and there are signs of renal, respiratory, or splanchnic compromise, a laparotomy to decompress the abdomen should be considered.17,18
Optimizing Cardiovascular Performance
Maintaining an adequate circulating volume and hemodynamic state is of paramount importance in preventing further renal injury. Patients with evolving renal failure should undergo careful examinations of the cardiovascular and respiratory systems. If there is hypotension or evidence of low cardiac output, central venous and intraarterial pressure monitoring is indicated. Measurement of superior vena cava oxygen saturation (SSVCo2; see Chapter 20) is also valuable. Hypovolemia should be treated by a balanced salt solution. Normal (0.9%) saline can exacerbate acidosis (see Chapter 31) and Plasma-Lyte (which contains potassium; see Table 32-3) can exacerbate hyperkalemia. If, despite adequate volume resuscitation, the hemodynamic state remains inadequate, pulmonary artery catheterization and echocardiography should be considered to guide further treatment.
Table 33-3 Criteria for the Initiation of Renal Replacement Therapy
Oliguria (urine volume <500 ml/day) |
Anuria (urine volume <50 ml/12 hr) |
BUN >30 mmol/l (>85 mg/dl) |
Creatinine >400 mol/l (>4.5 mg/dl) |
Potassium >6 mmol/l |
Pulmonary edema not responsive to diuretics |
Severe metabolic acidemia (pH <7.1, base deficit >10 mmol/l) |
Uremic encephalopathy |
Uremic pericarditis |
Uremic neuropathy |
From Bellomo R, Ronco C: Indications and criteria for initiating renal replacement therapy in the intensive care unit. Kidney Int 66(suppl):S106-S109, 1998.
In normotensive patients, the lower limit of autoregulation of renal blood flow is 70 to 75 mmHg.19 This lower limit is likely to be higher in patients with chronic hypertension. Furthermore, in critically unwell patients, autoregulation may be lost entirely, in which case renal blood flow becomes pressure dependent.19 In patients with deteriorating renal function despite adequate volume resuscitation and satisfactory cardiac output, the use of vasoactive drugs to increase mean arterial pressure (MAP) is appropriate. The optimal MAP varies among individuals, but initial targets of greater than 75 mmHg in previously normotensive patients and greater than 85 mmHg in previously hypertensive patients are reasonable. The appropriate drug to increase MAP is a matter of contention. In septic patients, norepinephrine appears to offer a survival advantage over other agents,20 but in patients with poor left ventricular function, it can cause a fall in cardiac output, which may adversely affect renal blood flow.
Other strategies
A number of other strategies have been used to ameliorate renal injury. They include:
Contrast Nephropathy
Contrast nephropathy is characterized by the abrupt onset of renal dysfunction within a few hours of a dose of a radiocontrast agent. It is more likely to develop in patients with hypovolemia or preexisting renal dysfunction, particularly if it is due to diabetes. The mechanism of contrast nephropathy is thought to involve altered vascular tone within the glomerulus, causing reduced renal blood flow.
If possible, use of intravenous contrast agents should be avoided in patients with evolving or established renal dysfunction. If these agents are unavoidable, then fluid loading prior to contrast exposure limits subsequent renal damage.24 Treatment with furosemide or mannitol is not indicated.24 N-acetylcysteine, an antioxidant, has been investigated for the prevention of contrast nephropathy. The data concerning its effectiveness are conflicting. Two recent metaanalyses of randomized trials have not demonstrated a clear benefit.25,26 However, a subsequent large randomized trial demonstrated a survival benefit in patients undergoing primary angioplasty for myocardial infarction treated with high-dose N-acetylcysteine (1200 mg intravenously prior to contrast administration, followed by 1200 mg orally twice a day for 2 days).27
Treating the Physiologic Consequences of Acute Renal Failure
Acidosis and Hyperkalemia
The development of severe metabolic acidemia or acidosis (pH <7.1, base deficit >10 mmol/l) or hyperkalemia (K+ >6 mmol/l) is an indication for urgent renal replacement therapy (Table 33-3). Measures to temporarily control the plasma potassium concentration so as to prevent cardiac arrhythmias may be indicated (see Chapter 32) but should not delay the institution of renal replacement therapy.
Sodium and Water Balance
Patients with pulmonary congestion secondary to circulatory overload should receive a loop diuretic such as furosemide. Increased doses or a continuous infusion may be required to achieve a satisfactory diuresis (see Chapter 3). Oliguric patients with circulatory overload should be restricted to less than 1.5 l/day of water (intravenous and oral) and sodium restricted. If hyponatremia is present, only water should be restricted. Enteral nutrition should be provided by a low-volume, low-sodium, low-protein, high-calorie “renal” feed (see Chapter 34). These restrictions can be relaxed if renal replacement therapy is instituted.
The role of loop diuretics in treating patients who have oliguria and systemic edema but no pulmonary congestion is less clear. The administration of a diuretic may convert oliguric renal failure into polyuric renal failure, which makes fluid management much easier. However, it is vital that intravascular volume depletion does not occur—particularly in patients being treated with vasoactive drugs to support MAP. In practice, diuretics are widely used to treat oliguria. Loop diuretics should be discontinued if they do not rapidly produce diuresis, because their continued use in the setting of oliguria can lead to ototoxicity (see Chapter 3). Also, their use should not delay the institution of renal replacement therapy when it is indicated.
Drug Dosing and Renal Failure
All drugs should be reviewed to ensure that they are indicated, are effective, and are being administered at the correct dosage. For drugs such as vancomycin, warfarin, gentamicin, phenytoin, and digoxin, therapeutic drug monitoring is indicated. For many drugs (e.g., most β-lactam antibiotics), a dose reduction based on the patient’s creatinine clearance is indicated (see Table 35-15). For loop diuretics, the dosage may have to be increased to obtain the same pharmacologic effect. A small number of renally eliminated drugs with toxic side effects (e.g., life-threatening arrhythmias due to dofetilide) should be avoided entirely. Nephrotoxic drugs were discussed earlier. Aluminum-containing drugs (e.g., sucralfate) should be avoided with renal dysfunction because they can lead to aluminum toxicity.
RENAL REPLACEMENT THERAPY
Recovery of renal function following an episode of acute renal failure may take days or weeks. During this time, artificial methods of blood purification are commonly required. A wide variety of techniques are available; collectively, they are known as renal replacement therapies. These techniques all have the same goal: the safe removal of water and solute (potassium, acids, and uremic toxins). The indications for renal replacement therapy in critically ill patients with acute renal failure are listed in Table 33-3. Preoperative renal function predicts the likelihood of the need for postoperative renal replacement therapy: preoperative creatinine clearances of less than 20, 20 to 40, 40 to 60, and above 60 ml/min are associated with the requirement for renal replacement therapy of 47%, 7%, 2%, and 1%, respectively.28
Renal replacement therapy may also be used to treat severe acidosis resulting from causes other than acute renal failure, such as hepatic ischemia or drug ingestion. There is also experimental interest in using renal replacement therapy to remove so-called middle molecules—compounds that have molecular weights of 1000 to 50,000 Daltons—to provide immunomodulation in patients with systemic inflammation.29
Principles of Renal Replacement Therapy
Ultrafiltration involves the mass movement of solvent (water) with its constituent solute molecules (solvent drag) across a semipermeable membrane (the hemofilter) on the basis of the properties of the membrane and the pressure differences (hydrostatic and osmotic; see Eq. 1-12) across the membrane. The transport of middle molecules occurs mainly by means of convection, not diffusion, and is dependent on the pore size of the membrane. Ultrafiltration results in the loss of extracellular fluid across the hemofilter. This fluid is known as ultrafiltrate, and its rate of production is controlled by a volumetric pump. For renal replacement therapies based mainly or entirely on ultrafiltration, the volume of the ultrafiltrate greatly exceeds the desired negative fluid balance, so replacement (or substitution) fluid must be given. Substitution fluid, like dialysate, is a sterile, buffered, balanced electrolyte solution (see subsequent material).
Devices and Circuits
Venous Access
Wide-bore (13.5French), kink-resistant catheters should be used for venous access. A long (25-cm) femoral venous catheter provides excellent flow characteristics but limits patient mobility. In patients who are mobilizing, a short (15 cm) catheter placed in the right internal jugular vein is preferable. If a left-sided jugular access is used, a longer catheter (20 cm) is required to ensure that the tip lies within the superior vena cava. Subclavian insertion should be avoided because complications are more common30 and flow characteristics are less favorable at this site.
TYPES OF RENAL REPLACEMENT THERAPY
Intermittent Hemodialysis
For acute renal failure in the ICU, daily (as opposed to alternate-day) dialysis is associated with a shorter time to renal recovery and improved survival rates.31 Following cessation of therapy, several hours are required for water and electrolytes to fully equilibrate. Potassium measured immediately after dialysis is typically 30% less than that measured 5 hours later. In patients who are receiving intermittent hemodialysis and who are anuric, sodium and water intake should be restricted (see earlier material). However, a normal protein intake (1 to 1.5 g/kg/day) should be maintained.
Continuous Renal Replacement Therapy
A number of techniques of continuous renal replacement therapy have been developed to treat acute renal failure in the ICU. The first to be used clinically was continuous arteriovenous hemofiltration; however, it has generally been superseded by continuous venovenous techniques. With continuous venovenous hemofiltration (CVVH), blood is pumped through a high-flux membrane, which usually has a lower surface area (1.2 to 1.6 m2) than that used for intermittent hemodialysis. Blood flow is typically 150 ml/min. Volumetric pumps control the rates of ultrafiltrate production and substitution fluid delivery. Ultrafiltration rates of 2l/hr or more are typical (see subsequent material). Fluid balance is determined by controlling the rate of ultrafiltrate production and the substitution fluid delivery. Solute removal is accomplished by solvent drag. CVVH is shown schematically in Figure 33-2.
The efficiency of solute removal by CVVH may be increased by the addition of hemodialysis. This is known as continuous venovenous hemodiafiltration (CVVHDF). With CVVHDF, dialysate is pumped countercurrent to blood flow as shown in Figure 33-2C. Total effluent flow is the sum of the ultrafiltrate and the dialysate volumes. Alternatively, a dialysis-only technique, in which there is no ultrafiltration, may be used. This is known as continuous venovenous hemodialysis (CVVHD). Much lower dialysate flow rates are used (1 to 2 l/hr) in CVVHD and CVVHDF than in intermittent hemodialysis.
Although there are few data to recommend one continuous renal replacement therapy over another, the delivered dose is important. For CVVH, ultrafiltration rates of 35 ml/kg/hr and 45 ml/kg/hr have been associated with lower mortality rates in critically ill patients than are found with the use of 20 ml/kg/hr.32 Suggested doses for CVVH and CVVHDF are listed in Table 33-4. Higher ultrafiltration rates (>50 ml/kg/hr), referred to as high-volume hemofiltration, have been used experimentally in an attempt to modulate the inflammatory response in the critically unwell.29,33 Thus far there are insufficient data to support the routine use of high-volume hemofiltration.
Table 33-4 Suggested Dosages for Renal Replacement Therapy in Acute Renal Failure in Critically Ill Patients
Substitution Fluids for Continuous Renal Replacement Therapy
A number of substitution fluids are available commercially; some examples are shown in Table 33-5. Lactated Ringer (Hartmann) solution may also be used (see Table 32-3). All are isotonic, buffered electrolyte solutions with sodium and chloride concentrations similar to those of extracellular fluid. If CVVHDF is used, the substitution fluid is also used for the dialytic component of treatment. Substitution fluids differ primarily in their potassium concentration and the choice of buffer.
The potassium concentration varies from 0 to 5 mmol/l, depending on the solution. With acute hyperkalemia, it is appropriate to use a zero- or low-potassium substitution/dialysis fluid to achieve rapid control of the plasma potassium concentration. Once potassium has normalized, a more physiologic potassium solution may be used. Potassium (as chloride or phosphate) may be added to the substitution/dialysis fluid to achieve the desired concentration (typically about 4 mmol/l).
The choice of buffer is important. Citrate anticoagulation (see subsequent material) is most easily managed using a citrate-buffered substitution fluid; otherwise the main choices are lactate or bicarbonate. Lactate-buffered solution is very stable, relatively inexpensive, and widely used. Use of lactate-buffered solution commonly results in moderate hyperlactatemia (5 to 10 mmol/l) and has a mild acidifying effect on the extracellular fluid.34 This is not usually clinically significant; however, with severe metabolic acidosis, more effective control of acid-base status is obtained by using bicarbonate-buffered substitution fluid. Bicarbonate buffered substitution fluid may also result in fewer adverse cardiovascular events than lactate buffered fluid.35 Bicarbonate-buffered solutions avoid the problem of lactic acidosis, but they are more expensive than lactate-buffered solutions and are unstable with long-term storage. The sodium bicarbonate component must be added immediately prior to use.
Substitution fluid may be added to the circuit postfilter or prefilter (predilution) (see Figs. 33-2A and B). Administration of the fluid postfilter provides the most efficient solute clearance, whereas administration prefilter avoids hemoconcentration within the filter, which reduces the likelihood that the filter will clot. If high rates of ultrafiltration are used (>20 to 30 ml/kg/hr), some of the substitution fluid may be administered prefilter to prolong filter life. With higher blood flows, solute clearance is minimally affected by predilution. If citrate buffered substitution fluid is used, all of it must all be administered prefilter (see subsequent material).
Hybrid Techniques
SLED and EDDF combine the advantages of intermittent hemodialysis (high-efficiency solute clearance, dialysis-free time) and continuous techniques (hemodynamic stability) and are well suited to treating acute renal failure in the ICU.36,37
Choice of Renal Replacement Therapy
The choice of renal replacement therapy for acute renal failure is determined by institutional practice, the availability of equipment, and the experience of the team responsible for its management. In hemodynamically unstable patients who are receiving vasoactive drugs, the hemodynamic stability obtained with continuous or hybrid techniques is a distinct advantage. In the recovery period after acute illness, however, dialysis-free time, which allows patients to mobilize and participate in physical therapy, is beneficial. Despite the increased utilization of continuous techniques,38 the results from small randomized trials have not demonstrated a survival advantage compared with intermittent hemodialysis in the critically ill.39–42 Suggested doses of the different types of renal replacement therapies used in the critically ill are shown in Table 33-4.
ANTICOAGULATION AND FILTER LIFE
Heparin
Systemic heparinization is the most widely used technique of anticoagulation, and it can be used with all forms of renal replacement therapy. It may be used at a low dose (i.e., 500 to 1000 IU/hr) with intermittent monitoring of activated partial prothrombin time (aPTT) or activated clotting time (ACT). If filter life is inadequate with low-dose heparin, full heparinization (see Chapter 30) is indicated, with the goal of keeping the ACT and aPTT in the therapeutic range.
Side effects of heparin include bleeding and heparin-induced thrombocytopenia (see Chapter 30). With severe coagulopathy or pathologic bleeding, renal replacement therapy may be administered without any anticoagulation or, alternatively, regional anticoagulation should be used. Regional anticoagulation may be achieved with the use of citrate or heparin. With regional heparin anticoagulation, heparin is administered prefilter, and protamine (1 mg for every 100 IU of heparin) is administered postfilter.
Citrate
Citrate anticoagulation is highly effective and avoids the problems associated with systemic heparinization.43 However, it requires separate infusions of citrate and calcium and careful monitoring of ionized calcium and acid-base status. Sodium citrate is infused prefilter, either as a stand-alone infusion or as a citrate buffered substitution fluid. Citrate complexes with free calcium and causes ionized hypocalcemia within the circuit (0.3 to 0.4 mmol/l44), which has a potent anticoagulant effect. Some of this calcium-citrate complex is lost in the ultrafiltrate; the remainder is metabolized in the liver to bicarbonate, with the calcium liberated back into the circulation. The calcium lost in the ultrafiltrate must be replaced by an infusion of calcium chloride. A protocol for citrate anticoagulation using citrate-buffered substitution fluid, which is used at my institution, is shown in Box 33-1, Figure 33-3, and Table 33-6.
Contraindications
Settings
Monitoring
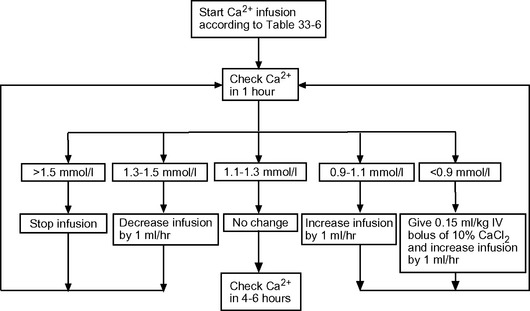
Figure 33.3 Algorithm for calcium chloride infusion (10% solution) in cases of citrate anticoagulation.
Table 33-6 Initial Substitution Fluid Flow and Calcium Chloride Infusion Rates for Citrate Anticoagulation of Continuous Venovenous Hemofiltration
COMPLICATIONS OF RENAL REPLACEMENT THERAPY
Other problems include hypophosphatemia, hypomagnesemia, thrombocytopenia, anemia, fluid balance errors, and depletion of water-soluble vitamins. Magnesium and phosphate are lost by diffusion and ultrafiltration and should be replaced. Magnesium complexes with citrate, and therefore special attention to magnesium levels are required when citrate anticoagulation is used. Thrombocytopenia is common as the result of platelet damage or sequestration by the filter and pumps and, occasionally, as the result of heparin-induced thrombocytopenia (see Chapter 30).
RENAL RECOVERY
Patients who survive acute renal failure have a good chance for renal recovery; less than 10% require long-term dialysis.6,32,45 Renal recovery is particularly likely when renal function is normal preoperatively.
1 Antunes PE, Prieto D, Ferrao de Oliveira J, et al. Renal dysfunction after myocardial revascularization. Eur J Cardiothorac Surg. 2004;25:597-604.
2 Provenchere S, Plantefeve G, Hufnagel G, et al. Renal dysfunction after cardiac surgery with normothermic cardiopulmonary bypass: incidence, risk factors, and effect on clinical outcome. Anesth Analg. 2003;96:1258-1264.
3 Conlon PJ, Stafford-Smith M, White WD, et al. Acute renal failure following cardiac surgery. Nephrol Dial Transplant. 1999;14:1158-1162.
4 Bove T, Calabro MG, Landoni G, et al. The incidence and risk of acute renal failure after cardiac surgery. J Cardiothorac Vasc Anesth. 2004;18:442-445.
5 Landoni G, Zangrillo A, Franco A, et al. Long-term outcome of patients who require renal replacement therapy after cardiac surgery. Eur J Anaesthesiol. 2006;23:17-22.
6 Luckraz H, Gravenor MB, George R, et al. Long- and short-term outcomes in patients requiring continuous renal replacement therapy post cardiopulmonary bypass. Eur J Cardiothorac Surg. 2005;27:906-909.
7 Chertow GM, Lazarus JM, Christiansen CL, et al. Preoperative renal risk stratification. Circulation. 1997;95:878-884.
8 Doolan PD, Alpen EL, Theil GB. A clinical appraisal of the plasma concentration and endogenous clearance of creatinine. Am J Med. 1962;32:65-79.
9 Bellomo R, Ronco C, Kellum JA, et al. Acute renal failure—definition, outcome measures, animal models, fluid therapy and information technology needs: the Second International Consensus Conference of the Acute Dialysis Quality Initiative (ADQI) Group. Crit Care. 2004;8:R204-212.
10 Kuitunen A, Vento A, Suojaranta-Ylinen R, et al. Acute renal failure after cardiac surgery: evaluation of the RIFLE classification. Ann Thorac Surg. 2006;81:542-546.
11 Nakamura K, Harasaki H, Fukumura F, et al. Comparison of pulsatile and non-pulsatile cardiopulmonary bypass on regional renal blood flow in sheep. Scand Cardiovasc J. 2004;38:59-63.
12 Nakamura K, Koga Y, Sekiya R, et al. The effects of pulsatile and non-pulsatile cardiopulmonary bypass on renal blood flow and function. Jpn J Surg. 1989;19:334-345.
13 Cooper WA, O’Brien SM, Thourani VH, et al. Impact of renal dysfunction on outcomes of coronary artery bypass surgery: results from the Society of Thoracic Surgeons National Adult Cardiac Database. Circulation. 2006;113:1063-1070.
14 Deray G. Amphotericin B nephrotoxicity. J Antimicrob Chemother. 2002;49(suppl 1):37-41.
15 Busauschina A, Schnuelle P, van der Woude FJ. Cyclosporine nephrotoxicity. Transplant Proc. 2004;36:229S-233S.
16 Sugrue M, Jones F, Deane SA, et al. Intra-abdominal hypertension is an independent cause of postoperative renal impairment. Arch Surg. 1999;134:1082-1085.
17 Sugrue M. Abdominal compartment syndrome. Curr Opin Crit Care. 2005;11:333-338.
18 Malbrain ML, Deeren D, de Potter TJ. Intra-abdominal hypertension in the critically ill: it is time to pay attention. Curr Opin Crit Care. 2005;11:156-171.
19 Bersten AD, Holt AW. Vasoactive drugs and the importance of renal perfusion pressure. New Horiz. 1995;3:650-661.
20 Martin C, Viviand X, Leone M, et al. Effect of norepinephrine on the outcome of septic shock. Crit Care Med. 2000;28:2758-2765.
21 Bellomo R, Chapman M, Finfer S, et al. Low-dose dopamine in patients with early renal dysfunction: a placebo-controlled randomised trial: Australian and New Zealand Intensive Care Society (ANZICS) Clinical Trials Group. Lancet. 2000;356:2139-2143.
22 Uchino S, Doig GS, Bellomo R, et al. Diuretics and mortality in acute renal failure. Crit Care Med. 2004;32:1669-1677.
23 Mehta RL, Pascual MT, Soroko S, et al. Diuretics, mortality, and nonrecovery of renal function in acute renal failure. JAMA. 2002;288:2547-2553.
24 Solomon R, Werner C, Mann D, et al. Effects of saline, mannitol, and furosemide to prevent acute decreases in renal function induced by radiocontrast agents. N Engl J Med. 1994;331:1416-1420.
25 Pannu N, Manns B, Lee H, et al. Systematic review of the impact of N-acetylcysteine on contrast nephropathy. Kidney Int. 2004;65:1366-1374.
26 Zagler A, Azadpour M, Mercado C, et al. N-acetylcysteine and contrast-induced nephropathy: a meta-analysis of 13 randomized trials. Am Heart J. 2006;151:140-145.
27 Marenzi G, Assanelli E, Marana I, et al. N-acetylcysteine and contrast-induced nephropathy in primary angioplasty. N Engl J Med. 2006;354:2773-2782.
28 Wijeysundera DN, Karkouti K, Beattie WS, et al. Improving the identification of patients at risk of postoperative renal failure after cardiac surgery. Anesthesiology. 2006;104:65-72.
29 Cole L, Bellomo R, Journois D, et al. High-volume haemofiltration in human septic shock. Intens Care Med. 2001;27:86-978.
30 Hernandez D, Diaz F, Rufino M, et al. Subclavian vascular access stenosis in dialysis patients: natural history and risk factors. J Am Soc Nephrol. 1998;9:1507-1510.
31 Schiffl H, Lang SM, Fischer R. Daily hemodialysis and the outcome of acute renal failure. N Engl J Med. 2002;346:305-310.
32 Ronco C, Bellomo R, Homel P, et al. Effects of different doses in continuous veno-venous haemofiltration on outcomes of acute renal failure: a prospective randomised trial. Lancet. 2000;356:26-30.
33 Laurent I, Adrie C, Vinsonneau C, et al. High-volume hemofiltration after out-of-hospital cardiac arrest: a randomized study. J Am Coll Cardiol. 2005;46:432-437.
34 Tan HK, Uchino S, Bellomo R. The acid-base effects of continuous hemofiltration with lactate or bicarbonate buffered replacement fluids. Int J Artif Organs. 2003;26:477-483.
35 Barenbrock M, Hausberg M, Matzkies F, et al. Effects of bicarbonate- and lactate-buffered replacement fluids on cardiovascular outcome in CVVH patients. Kidney Int. 2000;58:1751-1757.
36 Kumar VA, Craig M, Depner TA, et al. Extended daily dialysis: a new approach to renal replacement for acute renal failure in the intensive care unit. Am J Kidney Dis. 2000;36:294-300.
37 Kielstein JT, Kretschmer U, Ernst T, et al. Efficacy and cardiovascular tolerability of extended dialysis in critically ill patients: a randomized controlled study. Am J Kidney Dis. 2004;43:342-349.
38 Hyman A, Mendelssohn DC. Current Canadian approaches to dialysis for acute renal failure in the ICU. Am J Nephrol. 2002;22:29-34.
39 Mehta RL, McDonald B, Gabbai FB, et al. A randomized clinical trial of continuous versus intermittent dialysis for acute renal failure. Kidney Int. 2001;60:1154-1163.
40 Gasparovic V, Filipovic-Grcic I, Merkler M, et al. Continuous renal replacement therapy (CRRT) or intermittent hemodialysis (IHD)—what is the procedure of choice in critically ill patients ? Ren Fail. 2003;25:855-862.
41 Uehlinger DE, Jakob SM, Ferrari P, et al. Comparison of continuous and intermittent renal replacement therapy for acute renal failure. Nephrol Dial Transplant. 2005;20:1630-1637.
42 Tonelli M, Manns B, Feller-Kopman D. Acute renal failure in the intensive care unit: a systematic review of the impact of dialytic modality on mortality and renal recovery. Am J Kidney Dis. 2002;40:875-885.
43 Monchi M, Berghmans D, Ledoux D, et al. Citrate vs. heparin for anticoagulation in continuous venovenous hemofiltration: a prospective randomized study. Intens Care Med. 2004;30:260-265.
44 Dorval M, Madore F, Courteau S, et al. A novel citrate anticoagulation regimen for continuous venovenous hemodiafiltration. Intens Care Med. 2003;29:1186-1189.
45 Schiffl H. Renal recovery from acute tubular necrosis requiring renal replacement therapy: a prospective study in critically ill patients. Nephrol Dial Transplant. 2006;21:1248-1252.
46 Bellomo R, Ronco C. Indications and criteria for initiating renal replacement therapy in the intensive care unit. Kidney Int. 1998;66(suppl):S106-S109.