CHAPTER 224 Vertebral Column and Spinal Cord Injuries in Children
Injuries to the spinal cord and vertebral column are relatively uncommon in the pediatric age range from birth to 17 years. The incidence is 1% to 10% of all spinal injuries.1–13 Data from major pediatric spinal trauma centers11,14–20 indicate that injury to the juvenile spine differs from its adult counterpart with respect to anatomic and biomechanical features, mechanism of injury, response to deformation, injury pattern, and outcome. Several injury types are also unique to or prevalent in children, such as spinal cord injury without radiographic abnormality (SCIWORA), pure ligamentous injuries, atlanto-occipital dislocation (AOD), and atlantoaxial rotatory fixation (AARF). Finally, special problems are encountered in immobilizing the child’s inherently mobile yet fragile spinal column. This chapter deals with all these issues.
Biomechanical Considerations
In contrast to the adult spine, the juvenile vertebral column is inherently more malleable to deforming forces. This physiologic hypermobility allows considerable movement between vertebral segments without damage, but at the expense of providing less protection to the underlying spinal cord, which suffers deformation poorly. Several unique features of the juvenile spine account for this physiologic hypermobility. First, the ligaments and joint capsules are elastic and can withstand considerable stretching without tearing.21–25 This explains the phenomenon of pseudosubluxation in young children, or seemingly “excessive” angular displacement between C2 and C3 and, to a lesser extent, between C3 and C4.21–29 Second, because of their high water content, the intervertebral disk and annulus in children are also exceedingly expansile in the longitudinal axis, with the vertebral column of neonates being able to lengthen by as much as 2 inches without rupture when forcefully distracted.30 Third, the facet joints are shallow and oriented more horizontally than in adults,21,24–26 which permits translational as well as flexion and extension movements. Fourth, the immature vertebral bodies are wedged anteriorly21–26 such that forward slippage between adjacent segments is enhanced. Fifth, the uncinate processes of the mature vertebral body, which normally restrict lateral and rotational movements, are absent in children younger than 10 years.31,32 Sixth, the biologically active and hypervascular growth zone in the end plate represents another potential site of shifting; this structurally brittle zone splits readily from the primary centrum with even moderate shearing stress.33 Finally, the proportionally large size of the infant’s head, coupled with the relatively delicate nuchal musculature, predisposes the infant’s neck to wide flip-flop swings when subjected to flexion and extension forces.34
Dynamic radiographs show that the upper cervical segments in infants and young children are especially hypermobile in flexion and thus most susceptible to flexion injuries. The fulcrum for maximal flexion is at C2-3 in infants and young children. The horizontal orientation of the facets and the anterior wedging of the vertebral bodies are more prominent in the upper four segments of the cervical spine in this age group.21,23 The atlanto-occipital articulation in neonates also allows excessive sliding movements between the occipital condyle and the lateral mass of C1, partly because of the disparate matching between a large foramen magnum and a small C1 arch, the flimsy atlanto-occipital ligaments and condylar capsules,1 and the convexity of the articulating surfaces.2 Moreover, the protective groove on C1 for the vertebral artery, as it winds behind the C1 articular process to enter the cranium, is so shallow in neonates that during hyperextension the artery may be crushed between the condyle and the C1 arch, as was found on postmortem angiography in some victims of sudden infant death syndrome.35
Many of these features of the juvenile spine transform into “adult states” around 8 or 9 years of age.21,22,25,26 In particular, the vertebral bodies become less wedged and more rectangular, the facet joints deepen and become more vertical, the uncinate processes enlarge, and the ligaments gain in tensile strength. With increasing age, the head also assumes a smaller proportion of the body and thus lessens its own lever effect. With this normal transition, pseudosubluxation is seen less often,23,36,37 and the fulcrum for maximal flexion shifts from the upper cervical spine to C3-4 around 6 years of age and then to C5-6 in adolescence and early adulthood.24,38,39
The age distribution of SCIWORA lesions11,14,18,27,34,40 suggests that this biomechanical maturation takes place much more abruptly and successfully in the upper cervical segments such that the upper part of the neck, although vulnerable to flexion forces before the age of 8 years, becomes much more resistant to injury shortly afterward. The lower cervical spine, in contrast, seems to mature more gradually; lower cervical injuries occur with relatively equal frequency from birth to 16 years, even though serious lower cervical cord injuries tend to be more prevalent in younger age groups.
Clinical Characteristics of Pediatric Spinal Injuries
Epidemiology
There is a seasonal peak for all categories of pediatric spinal injuries from June to September because of the increase in extracurricular activities during the summer break from school. The only other solitary peak in the otherwise smooth distribution curve is in the 2 weeks around the Christmas holiday.17
The cause of injury varies with age (Table 224-1). In the younger age group (0 to 9 years), the two predominant causes of injury are pedestrian-versus-vehicle accidents and falls, together accounting for more than 75% of the injuries.14,16,37 In the intermediate age group (10 to 14 years), motor vehicle accidents, including motorcycle crashes, account for 40%, and falls and pedestrian accidents become less prevalent. This reflects the increasing role of older children as illegal drivers. In the late adolescent group (15 to 17 years), motor vehicle and motorcycle accidents become the leading cause of injury (>70%), and sports accidents also rise in number.14,16–18,37 In keeping with these statistics, injuries in the 0- to 9-year-old group tend to occur at or near home, such as the basement steps, upstairs window ledges, and local playgrounds. Older children and adolescents are more likely to sustain injuries at a greater distance from home.17
The male-to-female ratio in pediatric spinal injuries varies with age, which may indirectly reflect gender differences in injury-prone activities in the different age brackets. The boy-girl ratio is smallest in the 0- to 9-year-old group (1.1 to 1.3 : 1)14,16,18 because pedestrian accidents affect both sexes equally and boys are slightly more susceptible to falls. The ratio increases marginally in the 10- to 14-year-old group but markedly in the 15- to 17-year-old group (2.3 to 2.5 : 1),14,16,18 when aggressive sports and reckless driving are signatures of adolescent masculine behavior.
Age and Injury Pattern
In most series of pediatric spinal trauma,14,16–18,37 the patients are split evenly between those with SCI and those who are neurologically intact. Among the group with SCI (50% of the total), almost a third have complete cord injuries41 and two thirds have incomplete cord injuries of varying severity. In addition, about 30% to 40% of the patients with SCI have SCIWORA,14,16,18 although the reported incidence of SCIWORA in children with traumatic myelopathy varies widely from 5% to 67%,1–3,7,11,12,14,18,27,42–46 depending on the availability of diagnostic tests and the awareness of this syndrome in the local medical community.
The patient’s age profoundly affects the injury pattern. There is a significantly higher incidence of neurological injury in the 0- to 9-year-old group than in the 10- to 17-year-old group. Among SCI patients, younger children are also much more likely than older children to sustain complete (Frankel grade A) and severe (Frankel grades B and C) cord injuries.14,16 These trends reflect the inadequacy of the immature vertebral column as a protector of the spinal cord. In addition, there are more SCIWORA and pure ligamentous injuries in the 0- to 9-year-old group than in the 15- to 17-year-old group and, conversely, more fracture-subluxations in the 10- to 17-year-old group (Table 224-2).14,16,18,22 In several studies, the incidence of cervical spine fractures was 20% to 25% in the 0- to 8-year age range versus 70% to 80% in children older than 13 years.6,16,17 These statistics are also a reflection of the suppleness of the younger spine and its ability to yield without breakage against deforming forces, in contrast to the more “brittle” consistency of the adult-like spine in older children.
Besides the age factor, the degree of neurological compromise also correlates with the presence of subluxation. Children with only fractures tend to have fewer neurological injuries (20% to 25%) than do those with fracture-subluxations or subluxations without fracture (>50%).14
Age and Injury Site
The majority of spinal cord and vertebral column injuries in children occur in the cervical region. Osenbach and Menezes reported that all pure ligamentous injuries, 76% of SCIWORA, and 70% of fracture-subluxations involve the cervical spine.18 Kewalramani and colleagues also found that 76% of pediatric SCIs occur in the cervical spinal cord and only 24% in the thoracic cord and conus.7,8
The patient’s age is a strong determinant of the injury site, as predicted by the developmental changes in biomechanics (Table 224-3). Upper cervical and craniovertebral junction injuries are 2 to 3 times as frequent in children younger than 3 years14,18,47 because of the extreme hypermobility at these joints in this age group. Lower cervical and thoracic injuries occur with equal frequency in both the 0- to 9-year-old group and the 10- to 17-year-old group because maturation at these joints occurs much more gradually than at the upper cervical articulations.18,27 Thoracolumbar and lumbar injuries are primarily lesions of adolescence.
TABLE 224-3 Level of Injury Related to Patient Age in 301 Children
LEVEL OF INJURY | AGE GROUP, NO. OF PATIENTS (%) | |
---|---|---|
0-9 yr | 10-16 yr | |
Upper cervical (to C3) | 42 (53) | 47 (21) |
Lower cervical (C4-7) | 20 (25) | 76 (34) |
Thoracic | 8 (10) | 26 (11) |
Thoracolumbar | 4 (5) | 37 (18) |
Lumbar | 6 (7) | 35 (16) |
Total No. of patients | 80 | 221 |
Data from Hadley MN, Zabramski JM, Browner CM, et al. Pediatric spinal trauma: review of 122 cases of spinal cord and vertebral column injuries. J Neurosurg. 1988;68:18-24; and Hamilton MG, Myles ST. Pediatric spinal injury: review of 174 hospital admissions. J Neurosurg. 1992;77:700-704.
Finally, multiple noncontiguous spinal injuries are found in 10% to 16% of patients,14,16 thus emphasizing the need to survey the entire vertebral column carefully in every injured child.
Treatment
The same general principles that apply to adults also apply to children regarding the assignment of instability, fracture reduction, spinal immobilization, and surgical fusion. Early surgical intervention is seldom mandated after the fracture has been reduced and the spine immobilized. The only undisputed indication for emergency surgery is progressive neurological deterioration because of either an irreducible subluxation, in which case open reduction and fixation are necessary, or cord compression by a hematoma, extruded disk, or bone fragments, for which decompression and simultaneous fusion are recommended. These circumstances are quite rare in children. In the majority of cases, nonoperative management is the rule for at least the first few days, during which time the cord edema may resolve, serious extraneural injuries are dealt with, and the general prognosis is clarified. Thereafter, the indications for delayed surgery include irreducible fracture-subluxations, markedly unstable fractures, and pure ligamentous instability (see later).14,16,29,48–50 The specific treatment varies with the injury type. SCIWORA is, by definition, not associated with overt instability and requires only external immobilization.27,51
Among upper cervical spine injuries, such as epiphysiolysis of the odontoid process, hangman’s fracture, C2-3 subluxation, AOD, and AARF, all but the last probably require internal fixation. Among lower cervical injuries, most fracture-subluxations and teardrop fractures are extremely unstable and require surgical fusion. Burst fractures with compromise of the spinal canal should be treated by anterior corpectomy and strut graft fusion reinforced with an anterior cervical plate. Most simple compression body fractures without significant loss of vertebral height, as well as uncomplicated spinous process fractures (“clay shuffler’s” fractures), are stable and can be treated with an external orthosis. On the whole, about 25% to 30% of all pediatric spinal injuries require surgical treatment. About 60% of fracture-subluxations are surgically fused, whereas only 10% to 15% of patients with fracture but no subluxation require fusion.14,16,18 Most pure ligamentous injuries should probably be treated by internal fixation because ligaments tend to heal poorly with just immobilization.18 The management details and fusion techniques for specific injury types are discussed later in separate sections.
Outcome
In one large review, the mortality rate in children with spinal injury was 28%.15 This is astounding when compared with an 11% mortality for adult spinal injury during the same study period. The majority of these pediatric deaths occurred at the accident scene and were invariably associated with unsalvageable multiorgan injuries. Only a small number of deaths were directly attributable to the SCI, all of which involved the upper cervical cord and had fatal cardiorespiratory complications. The study predicted that the mortality associated with pediatric spinal injury will not be influenced significantly by changes in treatment strategy and that injury prevention is still the most effective way to improve survival.15,16,51,52
Despite the high mortality, children who survive have a good prognosis for neurological improvement.14,16,18,50,53,54 The sole determinant of functional outcome is the initial neurological status. In the majority of patients with complete cord syndrome at admission, the injury remains complete; only 5% to 10% show some improvement, and virtually none make a full recovery.5,16–18,27 Patients with severe cord injuries also tend to remain severely impaired. However, significant improvement is expected in those with mild to moderate cord injuries; 75% to 85% of these patients improve by one to two Frankel grades, and more than half of these patients enjoy a full recovery.5,16 The good outcome in pediatric SCI may be related to the enhanced plasticity of the young nervous system.
Pecial Injury Types
SCIWORA
The acronym SCIWORA was first coined and described as a distinct syndrome by Pang and Wilberger34 in 1982. Children with SCIWORA suffer a traumatic myelopathy without identifiable fracture or subluxation on plain spine films, tomography, and CT. Increasing recognition of this entity over the past decade has led to the accrual of a large pool of clinical data.7,14,16,18,27,47
Biomechanics and Mechanisms of Spinal Cord Injury
Hyperextension of the cervical spine forces the interlaminar ligaments to bulge forward into the spinal canal and may be responsible for up to 50% narrowing of the canal’s diameter.55,56 In addition, the cord thickens as it shortens during hyperextension.57 Thus, the space available for the cervical cord within the canal is reduced during moderate hyperextension. During violent hyperextension, Taylor and Blackwood56 and Bourmer58 demonstrated rupture of the anterior longitudinal ligament, shearing of the intervertebral disk from the end plates, and retrodisplacement of the upper vertebrae. Dynamic radiography on fresh cadavers showed that elastic recoil of the displaced segment could result in perfect spontaneous reduction and give a normal radiographic appearance.59 With its elastic constituents, the juvenile spine is especially susceptible to this sequence of momentary dislocation and spontaneous reduction. Intersegmental movement in children is further facilitated by their horizontal facet joints,21,23–26,32 and the immature vertebral body splits readily from its end plate within the brittle growth zone and leaves no radiographic trace.33 The elastic elements,21,32,60 horizontal facets,21,24–26 wedge-shaped bodies,21,23 and absence of uncinate processes likewise predispose the juvenile spine to hyperflexion myelopathy.31,32
The spines of infants and young children are also uniquely vulnerable to distraction.42,61 Leventhal found that although the elastic spinal column of neonatal cadavers could be stretched up to 2 inches without structural damage, the inelastic spinal cord ruptures if stretched more than 0.25 inch.62 The clinical counterpart of Leventhal’s study is the finding of frank rupture of the cord and meninges within a completely intact vertebral column in infants who had undergone forceful breech extraction.24,63 In nonobstetric SCIWORA, the best evidence of distraction injury is found in patients when the thoracic spine is forcefully distracted in lap belt injuries. Rupture of the dura and spinal cord in these patients is evidenced by the resultant cerebrospinal fluid–pleural fistula.64 Finally, the precarious architecture of the neonatal atlanto-occipital articulations predisposes the upper cervical cord to ischemic necrosis from occlusion of the vertebral artery.
The Age Factor
As predicted by biomechanics, age influences SCIWORA in three ways. First, many studies have shown that the incidence of SCIWORA is higher than that of other injury types in young children (0 to 9 years) with traumatic myelopathy, and vice versa in older children (Fig. 224-1).14,44,46,65 Second, young patients with SCIWORA typically have more severe neurological injuries than do their older counterparts (Fig. 224-2).5,46 In our own series, 21 of 30 children younger than 8 years suffered complete or severe injuries as compared with only 1 of 25 children older than 8 years (P < .000001).27 Similarly, Osenbach and Menezes noted severe injuries (Frankel grades B and C) in 21 of 22 patients younger than 9 years versus comparable injury severity in only 1 of 9 older children.18 Third, several studies have shown that young children are far more susceptible to upper cervical SCIWORA whereas lower cervical lesions occur more often in older children. In our series, 9 of 10 upper cervical injuries occurred in children younger than 8 years versus 21 of 33 lower cervical injuries in older children (Fig. 224-3).27 In the series of Osenbach and Menezes, all 7 nonobstetric upper cervical injuries occurred in children younger than 9 years.18 Unlike cervical lesions, SCIWORA involving the thoracic cord is evenly distributed over the pediatric age range, with no age predilection.18,27,46 Thus, because young children tend to have upper cord injuries and most of them are complete or nearly complete, the rehabilitation potential of this age group is very limited and the socioeconomic burden is accordingly prohibitive.
Radiographically Occult Instability
It is important to distinguish between the radiographically occult instability in SCIWORA and the overt instability seen in fracture-subluxation and subluxation without fracture (pure ligamentous injury). In SCIWORA, the spinal cord is injured without radiographic evidence of a fracture or subluxation that would indicate extensive ligamentous tearing and gross instability. Yet there had to have been sufficient bone displacement at impact to inflict damage to the cord. This implies that the stabilizing ligaments and fibrocartilaginous structures, although sufficiently elastic to stretch and recoil without rupturing, may have been severely sprained or even partially torn to render the involved vertebral segments vulnerable to repeated stress. The resulting instability or biomechanically precarious state is therefore “occult” rather than overt.20,21,24–27,33 The fact that flexion-extension radiographs fail to disclose abnormal movements in SCIWORA does not argue against the existence of occult instability because even gross instability can be masked by muscle spasm.37 Moreover, motion studies executed with deliberate caution cannot compare with the random and unguarded neck movements in real-life activities. It is therefore not surprising that post-SCIWORA dynamic films do not reveal the precarious state of the injured segments.27
Until the advent of MRI, the existence of occult instability in SCIWORA was unproved although strongly suggested by two phenomena: delayed neurological deterioration and recurrent SCIWORA. In a number of children with SCIWORA, neurological deficits are not detectable immediately after trauma but develop and worsen after a latent period of 30 minutes to 4 days, apparently in the absence of further trauma.27,40,43,66–68 The mechanism of this delayed deterioration remains speculative. A few cases may be due to posttraumatic occlusion of radicular arteries as a result of thrombosis or spasm, with subsequent delayed infarction of the cord,43,66 but this must be rare. The majority of cases probably result from repeated “punch-drunk” trauma to the cord during the latent period. This presumption is supported by two observations. First, many of these children recall transient neurological symptoms at the time of the initial trauma, such as subjective weakness, acroparesthesia, or Lhermitte’s phenomenon, thus indicating that the cord had been distracted or hit during the intersegmental displacement. This displacement may well have overstretched or partially torn crucial ligaments and rendered the segments susceptible to repeated shifts during otherwise innocuous neck movements. Second, the incidence of delayed deterioration at our institution has decreased dramatically (13 of 24 cases treated before 198234 versus 2 of 33 since 198227) because of the rigorous implementation of prompt neck immobilization and neurosurgical referral for any child with neck pain and transient neurological symptoms. Many of these children have been found to have subtle neurological deficits that could easily have been missed on cursory examination, or they show evidence of myelopathy on somatosensory evoked potential (SSEP) testing. Such patients would presumably have been at risk for reinjury if they had not been immobilized expeditiously.
The phenomenon of delayed neurological deterioration in SCIWORA suggests that the spinal column has been weakened temporarily by the impact, even if the initial neurological symptoms are mild. This concept of a postimpact period of vulnerability is supported by the phenomenon of recurrent SCIWORA, noted up to 10 weeks after initial SCIWORA in 8 of 42 children treated before 1986.69 Before this time, it had been our practice to immobilize children in hard collars for 2 months after SCIWORA. Four of the children with recurrent SCIWORA had removed their hard collars and were reinjured while engaging in forbidden sports such as wrestling, gymnastics, or basketball. The others were reinjured while wearing their collars. The neurological deficits resulting from the recurrent SCIWORA were much worse than those of the initial trauma, which in all cases were mild.69 It is noteworthy that none of these children had overt instability on dynamic radiographs either at the first SCIWORA or at the time of reinjury. These normal studies belie the precarious state of the “once touched” spinal cord when the unprotected neck is subjected to the erratic motions of a child at play.
Findings on Magnetic Resonance Imaging
The discovery of MRI abnormalities in the soft tissues of the spine represents the first direct evidence of occult ligament damage in SCIWORA.70 These abnormalities are extraneural, involving mainly ligaments and intervertebral disks, and neural, involving the cord itself.
Extraneural Abnormalities
The extraneural soft tissue injury demonstrated on MRI is well correlated with the mechanism of injury.70 For example, rupture of the anterior longitudinal ligament, seen as loss of continuity of the low-signal prevertebral line, widening of the anterior intervertebral space, and anterior disk herniation (Fig. 224-4), is found in cases of hyperextension. Rupture of the posterior longitudinal ligament, seen as loss of continuity of the retrovertebral line and small posterior disk herniation (Fig. 224-5), is correlated with hyperflexion. Intradiscal hemorrhages are seen when a translational interbody shearing mechanism is suspected. Hemorrhage of the tectorial membrane (tear) has been associated with the violent shaking of child abuse (Fig. 224-6), and hemorrhages in the interspinous and interlaminar ligaments are seen with distraction injury (Fig. 224-7). Furthermore, the level of these soft tissue injuries corresponds exactly to the level of the neurological lesion.19
These extraneural MRI findings strongly support the hypothesis that SCIWORA is associated with spraining or partial tearing of crucial stabilizing structures of the vertebral column.19,27 For years, these ligamentous and disk injuries, which are responsible for the post-SCIWORA vulnerable period, had escaped detection by conventional radiology but are now “exposed” by MRI. The continued use of MRI is expected to reveal more information about SCIWORA.
The signal characteristics of hemorrhage within non-neural tissues are detectable within hours of the injury on T1-weighted images as lines of hyperintensity because extravasated blood is converted within hours to methemoglobin (MHb) in non-neural tissues versus days to weeks in neural tissues (see later).4,30,71,72 Extraneural injuries in SCIWORA can thus be diagnosed by MRI within hours after the injury. The problem is that the adjacent fascial, muscular, and fat planes also produce high signal and can overwhelm the small streaks of MHb. The use of fat suppression sequences reduces the high signal of fat but not that of blood on short relaxation time (TR) (T1-weighted) images and is effective in highlighting the soft tissue hemorrhages in SCIWORA.
Neural Abnormalities
The MRI abnormalities seen within the traumatized spinal cord correspond to the signal characteristics of the various metabolized forms of extravasated hemoglobin (Table 224-4). Fresh blood from spinal cord hemorrhage contains intracellular oxyhemoglobin, which is rapidly converted to deoxyhemoglobin (DHb) within 3 hours. DHb is a stable intracellular compound and appears hypointense on long TR (T2-weighted) images.4,10,30,71,73 Gradient echo techniques, which are particularly sensitive to paramagnetic substances such as DHb, detect acute hemorrhage even better than do standard long TR sequences.12,30,71,73,74 Oxidation of intracellular DHb to MHb begins after approximately 3 days, although DHb may still be detectable up to 8 days after injury, especially with larger hematomas. Intracellular MHb is hyperintense on short TR images but hypointense on long TR images, whereas extracellular MHb appears hyperintense on both short and long TR images. Subsequent hemolysis around day 7 liberates MHb into extracellular tissues, where it may persist for months. Extracellular MHb is finally converted to hemosiderin after phagocytosis; this substance, which may persist for life, is markedly hypointense on long TR images and slightly hypointense on short TR images.73 The larger the hemorrhage, the longer it takes for all the DHb to be converted to MHb and ultimately for MHb to become hemosiderin. The signal characteristics are thus dependent not only on the time elapsed since injury but also on hematoma size.
TABLE 224-4 Magnetic Resonance Imaging Signal Alterations in Spinal Cord Injury As a Function of the Chemical State of Blood
Five patterns of parenchymal (cord) findings are seen on the post-SCIWORA MRI scan.70 (1) Complete disruption of the spinal cord is seen with severe distraction injuries in very young children (Fig. 224-8). This is usually accompanied by hypointensities on gradient echo sequences (DHb) of the rostral cord stump. The common sites of cord disruption are the upper cervical and upper thoracic levels. Severe flexion injury occasionally produces a similar picture. (2) Major cord hemorrhage has occurred when more than 50% of the cross-sectional area of the cord on axial MRI shows evidence of extravasated hemoglobin (Fig. 224-9). Depending on the timing of MRI, the signal characteristics may be those of DHb, intracellular or extracellular MHb, or hemosiderin. This pattern is associated with severe neural deficits and a dismal prognosis. (3) Minor cord hemorrhage has occurred when less than 50% of the cross-sectional area of the cord shows evidence of hemorrhage. It is associated with moderately severe initial deficits but a decent chance for recovery. (4) Edema only, without hemorrhage, is noted if the T1-weighted image shows isointensity or slight hypointensity but the T2-weighted image is intensely bright. No stage of hemoglobin metabolism produces this pattern of signal characteristics. Edema is predictive of a good outcome. (5) Approximately 35% of patients with clinically proven SCIWORA do not have MRI abnormalities in their spinal cords. These patients have an excellent prognosis for complete recovery.75
Thoracolumbar SCIWORA
About 13% of cases of SCIWORA involve the thoracic cord. SCIWORA of the thoracic spine is typically due to violent trauma, such as high-speed automobile accidents or crushing injuries.* Accordingly, these patients have a high incidence of associated thoracic, abdominal, and pelvic injuries. Three discrete subgroups of patients with thoracic SCIWORA are encountered. One consists of children who sustain severe distraction injuries related to lap seat belts and high-speed vehicular collisions.64 CT myelography typically shows spinal cord and dural rupture and free flow of contrast material into the mediastinum.25,42,76,77 The splinting effect of the rib cage normally protects the thoracic spine against horizontal displacement as a result of flexion and extension forces. However, because the sternocostal joints permit a fair amount of “bucket handle” movements, the rib cage provides little protection against longitudinal distraction. In lap belt injuries, there is always a component of forceful distraction caused by forward propulsion of the upper part of the chest while the pelvis is harnessed by the lap belt. The elastic juvenile spinal column then recoils like a spring to restore normal configuration after having stretched enough to rupture the cord and dura. As with upper cervical SCIWORA, thoracic SCIWORA secondary to distraction occurs almost exclusively in children younger than 8 years.
A second subgroup of thoracic SCIWORA includes children who are crushed by slow-moving vehicles.3,27 In these cases, tire marks are clearly imprinted on the body, so the body position and exact site of crushing can be determined. All thoracic SCIWORA victims injured by crushing were lying prone when run over by the cars that left tire marks on their backs. In contrast, children with tire marks on the abdomen or anterior chest region typically suffer intraperitoneal injuries but rarely SCI. In the group with ventral tire marks, the gentle S curve of the thoracic spinal column, being solidly buttressed by the ground, merely straightens out and is thus protected against excessive intersegmental displacement; the abdominal viscera bear the brunt of the injury. In the group with dorsal tire marks, the spinal column is hyperextended into the softness of the chest and abdominal cavities, thereby resulting in hyperextension cord injury and rupture of retroperitoneal organs while sparing the well-protected intraperitoneal contents.
Management
The initial management of a child with a presumed SCI begins in the field (Fig. 224-10). Patients with symptoms or mechanism of injury compatible with SCI should be immobilized supine in a hard collar on a fracture board. If the child’s head is large, the body from the shoulders down is propped up with folded blankets or foam sheets to prevent forced flexion of the neck.
In children with severe cord injuries diagnosed within 8 hours of the injury, a 24-hour course of high-dose corticosteroids is initiated. This recommendation is based on the beneficial effects of high-dose methylprednisolone in adult patients reported by the National Acute Spinal Cord Injury Study (NASCIS III).80–82 There are no equivalent data for children, and our endorsement of corticosteroids for SCIWORA must be understood to be “empirical” rather than evidence-based. Patients with cervical cord injuries are immobilized in a Guilford brace (B.A. Guilford and Sons Orthotic Laboratory Ltd., Cleveland, OH) or other cervical-thoracic brace for 3 months. Mid to low thoracic lesions are treated with a thoracolumbar orthosis (TLSO), and children with upper thoracic lesions are fitted with a TLSO with added chin and occiput supports. Physical and occupational therapies are begun in the intensive care unit (ICU). Those with severe residual deficits are transported to a rehabilitation facility when clinically stable. Patients with mild residual weakness are enrolled in an outpatient physical therapy program. Bracing is maintained for 3 months, during which time both contact and noncontact sports are strictly prohibited. The brace is favored over a hard collar for several reasons: (1) it can be tailored to fit children as young as 1 year; (2) it imparts greater limitation of motion; (3) it produces less skin irritation and chafing than a collar; and most importantly, (4) a child cannot easily remove the brace, which also interferes with play activity, both helping to enforce compliance. It may be helpful to embellish with graphic details the estates of paralysis, wheelchair existence, decubitus ulcers, impotence, and so on to older children and their families as a warning of the dire consequences of protocol violation.
Our decision to immobilize for 3 months is based anecdotally on the fact that one patient treated with just 2 months of wearing a hard collar suffered recurrent SCI with trivial trauma 10 weeks after the initial SCIWORA. Since changing from our old protocol of using just a hard collar for 2 months,34 no recurrent SCIWORA has been reported.64 The improved outcome may just as well be due to switching of the collar to bracing as to switching from 2 months to 3 months. Currently, there are institutions that favor the 2-month protocol, and unless adverse results are reported with the shorter immobilization, the choice of 2 versus 3 months must be left to the individual belief of the treating physician. After 3 months, flexion-extension radiographs are obtained with the child out of the brace to check for late instability. In our experience, this is rarely a problem with SCIWORA, nor is late deformity such as kyphosis or scoliosis.19,20,83
SCIWORA in the Comatose Child
The question is often asked how to “clear the neck” of a comatose child. A normal spine film and CT scan rule out most cases of gross fracture and dislocation, but not SCIWORA. In some ICUs, a comatose child is kept on a hard collar and flat log-rolling regimen as long as the child is unresponsive or being maintained on mechanical ventilation, but this could last weeks and skin breakdown around the chin and occiput can become troublesome long before a valid neurological examination is feasible. In this setting we suggest performing MRI and using SSEPs to detect spinal cord conduction block.75 If both MRI and SSEPs are normal, the spinal precautions are removed. If either the SSEPs or MRI is abnormal, strict spine precautions are observed until the patient is able to mobilize, at which time a proper brace is fitted.
Outcome
Several studies,5,18 including our own from 1989,27 have shown that the main predictor of long-term outcome in children with SCIWORA is the admission neurological status. Children with complete lesions rarely improve. Those with severe but incomplete lesions often improve with time but seldom regain normal function. Only patients with mild to moderate initial deficits can hope for a full recovery (Fig. 224-11). Thus, the overall outcome for SCIWORA can best be improved by preventing serious neurological damage. Primary prevention programs within the community such as ThinkFirst have lowered the overall incidence of SCI in children. Once SCIWORA has occurred, the pediatric neurosurgeon’s responsibility would be to minimize the extent of neuronal damage through three avenues: (1) rule out other (non-SCIWORA) categories of SCI, (2) anticipate and prevent delayed neurological deterioration, and (3) prevent recurrent injury. This means that occult fractures, disk herniation, epidural hematoma, and overt ligament instability must be excluded with dynamic radiographs, CT, and MRI before the diagnosis of SCIWORA can be established because each of these entities mandates more than simple bracing. The results reported by Pang and Pollack27 and Pollack and colleagues69 suggest that the incidence of delayed neurological deterioration and recurrent SCIWORA can be lowered by compulsive prehospital care and a low threshold for the use of spinal immobilization in any injured child meeting our diagnostic criteria for SCIWORA.
Prognostic Value of Magnetic Resonance Imaging in SCIWORA
Although preventive measures are still paramount in improving outcome, recent MRI data75 suggest that our earlier outcome predictions made without MRI27 have become somewhat obsolete. In our recent series,75 we correlated the five neural MRI abnormalities (really four abnormalities plus “normal”) with the four grades of neurological deficit (complete, severe, mild, and normal) at initial evaluation and 6 months later in a much larger population (Fig. 224-12). We found instead that although the general conclusions from the original outcome analysis27 stand, in specific cases MRI surpasses the initial neurological examination in predicting outcome. The implication of cord disruption, once clearly portrayed on MRI, is self-evident. In all patients with this finding, who invariably have complete sensorimotor paralysis, the deficits remain complete. Major hemorrhage is also associated with complete or severe deficits (Frankel grades B and C) at initial evaluation, as well as with very poor long-term outcome.71,84 Patients with this finding either remain profoundly impaired or achieve only minimal recovery. No one in our series ever ascended from severe to the mild or moderate category (Frankel grade D) of cord syndromes, although two patients initially found to be complete were raised to the severe grade. In short, cord disruption and major hemorrhage are no better predicators of outcome than the admission neurological examination.
All patients with no cord abnormality on post-SCIWORA MRI, except two, had mild initial deficits, and all made a complete recovery. The two exceptions initially had complete sensorimotor paralysis but within 2 days had also made a complete recovery.70 This phenomenon of “cord concussion” had been described by Quencer, who thought that the “chemical alterations” within the cord that led to the initial paralysis did not generate abnormal MRI signals.85 Thus, normal MRI findings always presage an excellent prognosis regardless of the initial neurological grading.
Pure Ligamentous Injuries (Subluxation without Fracture)
Unlike the case with SCIWORA, the diagnostic criteria and management guidelines for overt instability in children are not well defined. Certainly, if the radiograph shows extensive bone fractures or subluxation, the implication of gross instability is obvious. However, the biomechanics of many subtler kinds of ligamentous instability in children has not been studied as intensely as it has been in adults, and recommendations for children are often extrapolated from adult data. For example, cadaver studies by Fielding and coworkers regarding stability of the atlantoaxial joint showed that (1) the atlantodental interval (ADI) in normal adults seldom exceeds 3 mm, (2) an ADI of 3 to 5 mm implies rupture of the transverse ligament, (3) an ADI of 5 to 10 mm suggests additional incompetence of the alar ligaments, and (4) an ADI greater than 10 mm exists only if all other accessory odontoid ligaments are also ruptured.86,87 Thus, an ADI greater than 3 mm in adults is considered unstable.88 Equivalent scientific data are unavailable in children. From purely empirical observations and taking into account the elasticity of the juvenile ligaments, an ADI of 4 mm has been regarded as the upper limit of normal in children younger than 8 years.86,89 Other studies have also shown that 20% to 30% of children with trisomy 21 and an ADI greater than 6 mm deteriorate with time, thus suggesting that 6 mm should be used as the threshold for treatment in children with Down syndrome.90–93
Similar biomechanical studies of the subaxial spine by White and Panjabi and their associates showed that the angle between adjacent vertebrae in normal adults is always less than 11 degrees and that deformities greater than 11 degrees in adults are considered unstable.88,94–98 For children, however, the upper limit of normal should be less than 11 degrees. The reason is as follows: because the juvenile spine is so elastic and apt to recoil, the final resting position of the displaced segment may lie well short of its actual maximal excursion at the time of impact. The initial angle of displacement on the lateral radiograph is thus deceptively small, but the actual ligamentous disruption may have been profound. This scenario is illustrated by the case of a 10-year-old boy who suffered a flexion injury and was found to have an asymmetric central cord syndrome with a radiograph showing a kyphotic deformity of 8 degrees and horizontal displacement of 2 mm at C4-5. No dynamic radiographs were obtained for fear of aggravating the neurological deficits, but because the angulation did not satisfy the 11-degreee adult rule, he was initially treated with a halo vest and not surgical fusion. When the halo was removed 3 months later, his kyphosis progressed rapidly and he ultimately required a posterior fusion (Fig. 224-13).
This case illustrates the dilemma when the neutral radiograph does not meet the 11-degree instability rule yet the neural deficits suggest ligamentous damage but also preclude a flexion study. In retrospect, this boy’s instability was obviously present despite the 8-degree angulation on the initial film. Such examples are common in pediatric neck injury. To avoid underdiagnosis, we recommend the following algorithm for children with angular deformities in the subaxial cervical spine (Fig. 224-14):
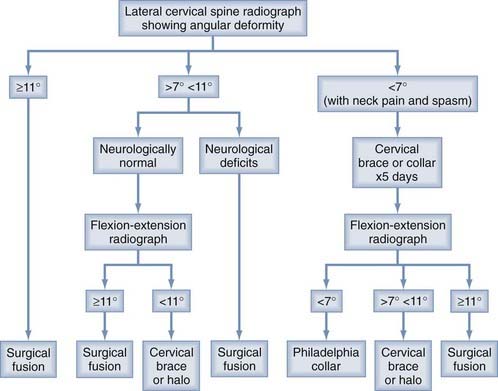
FIGURE 224-14 Management algorithm for ligamentous instability in children if the lateral radiograph shows a kyphotic deformity.
Another case illustrates the importance of flexion studies (Fig. 224-15). A 12-year-old girl suffered a flexion injury and had a painful stiff neck, a normal neurological examination, and a neutral film that showed an angulation of 9 degrees at C4 and C5. A flexion study showed that the angle increased to 12 degrees. Nonetheless, the child was immobilized in a Philadelphia collar for only 14 days, at which time a repeat radiograph showed that the kyphosis had increased to 17 degrees. Under the present guidelines, this patient would have undergone surgical fusion after the flexion study.
Besides the 11-degree rule, the studies of White and Panjabi showed that horizontal interbody displacement of greater than 3.5 mm in adults implies significant ligamentous rupture that will result in delayed instability.88,96–98 The juvenile spine, however, exhibits much greater physiologic horizontal movement than the adult spine, and displacement greater than 3.5 mm may be considered normal. Sullivan and coworkers noted forward glide of 3.5 to 4 mm between C2 and C3 in 13 of 100 normal children.23 Cattell and Filtzer observed similar findings in 19% of normal children younger than 8 years.26 In all probability, this “pseudosubluxation” between C2 and C3 and, to a lesser extent, between C3 and C4 is due to the elastic supporting soft tissues. The following recommendations are helpful in distinguishing clinical instability from physiologic hypermobility in the subaxial segments of children:
Atlanto-occipital Dislocation
Traumatic AOD is one of the most common fatal cervical spine injuries. Autopsy and postmortem radiographic examination of traffic fatalities revealed a 20% to 35% incidence of AOD.99–101 Improvements in emergency resuscitation and prehospital care have increased survival in patients with this previously almost invariably fatal injury. AOD is a particularly important entity in pediatric spine trauma, not only because it occurs more than twice as frequently in children as in adults102 but also because children who survive the initial crisis often make good recoveries despite the severe initial neurological deficits.103,104
Anatomy and Biomechanics
Movements within the O-C1-C2 unit are facilitated by the redundant articular capsules and thin occipitoatlantal and atlantoaxial membranes, which in turn impart minimal stability.98 Rather, stability is provided by the strong internal ligaments between C2 and the occiput, chiefly the tectorial membrane, a well-developed continuation of the posterior longitudinal ligament that straps the body of C2 to the clivus; by the paired alar ligaments that connect the dens to the occipital condyles; by the cruciate ligament; and by the apical dental ligament (Fig. 224-16). Flexion of the basion beyond the tip of the dens is limited by the tectorial membrane, whereas extension is checked by the tectorial membrane and by bony contact between the opisthion and the arch of C1. Lateral bending and rotation are controlled by the alar ligaments.105 The importance of the tectorial membrane and the alar ligaments was demonstrated by Werne, who found that sectioning of the former results in excessive flexion and extension, as well as vertical separation of the basion from the dens beyond the normal range of 1 cm, whereas sectioning of the latter results in increased lateral bending and rotation.106 When both the alar ligaments and tectorial membrane are cut, complete occipitoaxial dissociation occurs. The remainder of the internal ligaments—namely, the ascending band of the cruciate ligament and the apical ligament—are ineffectual in limiting motion. Finally, cadaver studies have shown that paraspinal muscle action also contributes to stability of the O-C1-C2 unit.107,108
Pathology and Pathogenesis
Traumatic AOD results from high-energy impacts that cause rupture of the tectorial membrane and alar ligaments.65,99,101,102,109–111 Pedestrian-vehicle accidents are the most common cause. Three factors make children more vulnerable to AOD than adults. First, children are more frequently involved in pedestrian-vehicle accidents.112 Second, the relatively larger head in children in comparison to the torso generates a greater shearing moment. Third, the occipitoatlantal joint is inherently more unstable in children.113
The force vectors involved in AOD may be reconstructed by circumstantial evidence. Adams’ study revealed a high incidence of associated submental lacerations and mandibular fractures,99 and others have reported posterior atlas fractures,114 both of which point to a hyperextension mechanism. Furthermore, 9 of Adams’ 12 subjects had pontomedullary lacerations,99 a lesion that is characteristic of hyperextension and rotation.115–117 However, approximately 25% of patients with AOD have coexistent anterior atlantoaxial subluxation, and other patients are seen with spinomedullary transection by a posteriorly pointing dens.99,110 These findings suggest a hyperflexion mechanism.118 Because the alar ligaments—the check ligaments for rotation—are also ruptured in AOD, the forces must be directed obliquely to the head.105,119–121 Thus, AOD is most often caused by hyperextension-distraction forces directed obliquely to the chin, but hyperflexion-distraction forces to the occiput are involved in some cases.
Recently, Sun and colleagues122 used high-resolution MRI to reveal a progressive pattern of disruption of the structures responsible for the stability of the O-C2 joint. Progressing from minor to severe injuries, the sequence of successive and additive involvement of structures is as follows: suboccipital musculature, atlanto-occipital and atlantoaxial membranes, apical and alar ligaments, tectorial membrane, and bilateral atlanto-occipital joint capsules. These authors postulated that involvement of the tectorial membrane is a critical threshold for instability at the O-C2 joint. Tectorial membrane damage can consist of frank rupture (Fig. 224-17) or stripping of the membrane from the clival or C2 attachments (Fig. 224-18). In both cases, the mechanical advantage of the membrane’s stabilizing function is rendered ineffective. Weakening of this membrane appears to “expose” the O-C1 joint capsules to overt disruption, the denouement of the AOD scenario, with the cord being left vulnerable to traction and crushing injuries. In our recent series of AOD in which 14 children underwent MRI,123 seven tectorial membranes had ruptured, three had been stripped off the clivus, and only four had remained intact.
Traynelis and coworkers classified AOD into three types.118 Type I, the most common, is characterized by anterior displacement of the occiput on the atlas. Type II is a longitudinal separation of the two structures, and type III is posterior displacement of the occiput. The prevalence of anterior AOD seems contradictory to the postulated hyperextension vector, but given the highly unstable nature of this injury, the location of the head relative to C1 may be more a function of postimpact positioning than the dynamics of the impact. As an example, when an injured child lies on a flat board, the occipital prominence of the large head forces the neck into flexion, thereby artificially creating an anterior displacement pattern. Rigid classification of AOD according to the relative position of the head therefore carries no correlative value with regard to mechanism and should be discouraged. Isolated examples of pure lateral dislocation,124 as well as pure rotatory subluxation, further attest to the extreme looseness of the disrupted O-C1 joint.125,126
Clinical Findings
Children with traumatic AOD have signs of brainstem, spinal cord, and cranial nerve injury. Thirty percent of children who survive AOD are apneic or in full cardiorespiratory arrest at the scene of the accident.47,127–133 Other brainstem findings include pupillary abnormalities, rotatory nystagmus, ocular bobbing, decerebrate posturing, and variable alterations in consciousness.123 The motor deficits include quadriparesis, cruciate paralysis, crossed hemiplegia, or hemiparesis, depending on the level of the pyramidal tract lesions. Brainstem injury in AOD is due to a combination of actual disruption of tissues, spinal medullary compression by bony structures or epidural hematoma, and ischemia secondary to vertebral artery spasm or thrombosis.134 Thrombosis of the anterior spinal artery produces the unique syndrome of ipsilateral hypoglossal palsy and crossed hemiplegia.135 The caudal six pairs of cranial nerves may also be injured from downward traction of the medulla against the fixed points at their exit foramina.136 However, the most common cranial neuropathy in AOD, sixth nerve palsy, is probably caused by the concomitant head injury. Posttraumatic hydrocephalus must be ruled out if the abducens palsy persists.
The true mortality rate associated with AOD is unknown. Of 35 nonfatal cases of pediatric AOD reported in the literature,104,111,122,129–133,136–145 only 5 had no initial deficits except for neck pain and torticollis. Of the 30 patients with deficits, 12 recovered fully; 3 remained quadriplegic and ventilator dependent, 2 of whom died 8 months after injury. The remaining 15 patients experienced various intermediate recoveries. Thus, children with AOD who have incomplete initial paralysis often make significant improvements, but in isolated cases, even initially flaccid paralysis does not preclude a good recovery.
Radiographic Diagnosis
One of the oldest criteria is the interval between the tip of the dens and the basion (DB interval) (Fig. 224-19). Wholey and associates examined 480 adult and 120 pediatric cervical spine radiographs and determined that the upper limit of the DB interval is 5 mm in adults and 10 mm in children, the age difference being attributed to incomplete summit ossification of the dens in children.146 Earlier application of the DB interval revealed considerable overlap between normal subjects and patients with AOD, possibly because of the mixed age groups.110 The most recent use of the DB interval exclusively in children showed that the value in normal children is less than 12.5 mm; the values in 11 children with proven AOD were all greater than 14 mm.138 However, several problems are inherent in this method. First, as with any absolute radiographic measurement, the number depends on the target-film distance. Wholey’s original measurements were done on an upright film at a target-film distance of 6 ft. When the DB intervals in 400 subjects were measured on supine films taken at 40 inches, Harris and colleagues found the upper limit to be 12 mm in adults.147 Second, the variable ossification of the odontoid summit in children makes universal application of a maximal DB interval unreliable. Third, the measurement varies with flexion, extension, and axial traction in normal subjects. Nevertheless, a DB interval greater than 14 mm in a child is almost certainly indicative of AOD, but values between 10 and 14 mm still defy precise designation.
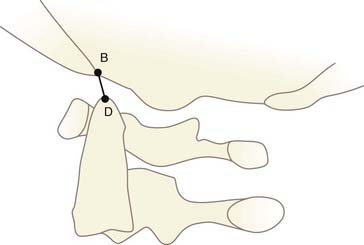
FIGURE 224-19 The dens-basion interval introduced by Wholey and colleagues.146 An interval of 10 mm is abnormal, and 14 mm is indicative of atlanto-occipital dislocation in children. B, basion; D, dens.
In 1980, Dublin and associates measured the distances from the posterior cortex of the mandible to the anterior arch of C1 (A) and to the odontoid (B) (Fig. 224-20).109 In anterior AOD, both A and B should be increased. However, because the mandible and the dens are not sagittally coplanar, these measurements vary greatly with the incident angle of the x-ray beam and are not consistently reproducible.34 We do not recommend this method.
To circumvent the problems with noncoplanar landmarks, Kaufman and coworkers measured the width of the atlanto-occipital joint itself.49 They found that the distance between any two opposing points along the joint surface should not exceed 5 mm in children on lateral or anteroposterior skull films. The problem with their original description is the use of plain films; superimposition of the mastoids and imperfect overlap of the two atlanto-occipital joints often make interpretation impossible.
Two methods using dimensionless numbers have been devised to eliminate the error attributable to radiographic magnification. The Powers ratio is obtained by dividing the distance between the basion (B) and the posterior arch of C1 (C) by the distance between the opisthion (O) and the anterior arch of C1 (A) (Fig. 224-21A).111 Survey of a normal population yields a mean BC/OA ratio of 0.77 ± 0.09. In the so-called type I AOD, the BC/OA ratio is increased because BC widens whereas OA shortens. Powers found that ratios greater than 1 are always indicative of anterior dislocation. Although highly predictive of anterior dislocation, the Powers ratio has two major shortcomings: it fails to diagnose distraction and posterior dislocation, and the opisthion is often difficult to pinpoint on the lateral spine films. In addition, anomalies of the posterior arch of C1 and the foramen magnum invalidate the ratio. The use of sagittally reconstructed CT has greatly simplified localization of the opisthion (see Fig. 224-21B).
The second dimensionless method uses the X lines proposed by Lee and colleagues134 (Fig. 224-22). Normally, BC2L and C2O tangentially intersect the dens and the highest point on C1, respectively. If both lines miss their tangential points, AOD is suspected. This method is not useful in children because its validity depends on atlantoaxial integrity and a fully developed dens.
In 1993, Harris and coauthors began using the basion-axial interval (BAI), defined by a line drawn tangential to the posterior cortical surface of the axis (posterior axial line [PAL]).147,148 The BAI is the distance between this line and a parallel line drawn through the basion (Fig. 224-23A). The normal BAI in adults ranges from +12 mm (basion anterior to the PAL) to −4 mm (basion behind the PAL). With anterior dislocation, the basion moves away from the dens and the BAI exceeds 12 mm (see Fig. 224-23B), whereas with posterior dislocation, the basion moves far behind the dens and the BAI becomes less than −4 mm. In 50 children studied, the anterior limit of the BAI was still +12 mm, but the basion never moves behind the PAL. These authors showed that when used in conjunction with the DB interval, the BAI is more accurate in adults than either the Powers ratio or X lines. In very young children with a pointed dens, however, the PAL becomes excessively oblique forward. This artificially moves the posterior reference point of the measurement forward and, in effect, underestimates the true extent of the anterior basion displacement.
In 2000, Sun and colleagues introduced the C1-2/C2-3 posterior interspinous ratio to assess the integrity of the atlanto-occipital joint.122 This ratio is obtained from the lateral plain radiograph by dividing the shortest distance between the posterior C1 ring and the C2 spinous process by the distance between the spinous processes of C2 and C3 (Fig. 224-24). These authors used abnormality of the tectorial membrane as the positive indicator for AOD and found that normal children and injured children with intact tectorial membranes (presumably without AOD) have an interspinous ratio of less than 2.5 whereas seven of eight children whose tectorial membranes had been damaged (presumably with AOD) had a ratio greater than 2.5. The hypothetical mechanism underlying an abnormal ratio in AOD was supposedly related to forward rocking and downward pressing of the occipital condyle on the anterior rim of the C1 facet secondary to a loose atlanto-occipital joint, thereby forcefully “jacking up” the posterior arch of C1 away from C2.122
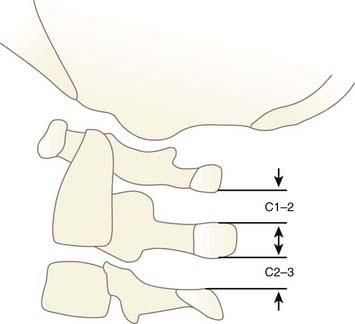
FIGURE 224-24 The C1-2/C2-3 ratio. Sun and colleagues suggest that the ratio should be less than 2.5 with a stable atlanto-occipital relationship.122
In a recent study comparing the diagnostic sensitivity and specificity of Wholey’s DB interval, Powers’ ratio, Harris’ BAI, and Sun’s interspinous ratio, Sun’s method was found to have the lowest sensitivity (25%).123 There are several explanations. First, AOD has been shown to occur without demonstrable injury to the tectorial membrane,123 which invalidates the use of its intactness as the reference standard for AOD. Second, if the abnormal C1-2 interspinous widening is due to altered contact relationships between the condyle and the facet of C1 after the loss of ligamentous restraint, postinjury handling of the occiput could randomly rearrange this relationship and unpredictably vary the C1-2 distance. If one takes Sun’s logic to extremity, in the most ferocious case of AOD when all the stabilizing ligaments are completely torn and the condyles are freely floating away from C1, C1 should not be pressed or levered by the condyle and the ratio should stay normal. This has in fact been observed in some of our patients.123 Thus, paradoxically, the more complete the disintegration of the O-C1 joint, the more likely would Sun’s criterion be falsely negative.
Despite such a large array of published radiographic criteria, it is ironic that 6 of the senior author’s 16 cases of AOD fell into the diagnostic borderland between normal and abnormal.123 This led to the realization that with the exception of Kaufman and associates’ flawed study,49,131 all the aforementioned criteria use bony landmarks that do not actually participate in the atlanto-occipital articulation. It stands to reason that direct measurement of the O-C1 joint interval should be a more precise indicator of O-C1 integrity.
Condyle-C1 Interval
Such a study using both coronal and sagittal reformatted CT images through the condyle-C1 joints of 89 normal children was published by us in 2007.149 We found that the normal occipital condyle-C1 joint in children is held tightly together by strong ligaments, with a mean condyle-C1 interval (CCI) of 1.28 mm (Fig. 224-25). No individual gap measurement in any projection exceeds 2.5 mm. In addition, there is strong left-right joint symmetry in both the CCI and conformational anatomy. Linear regression analysis between age and CCI shows that the mean CCI value is extremely stable through the age span of birth to 18 years and, furthermore, the left-right symmetry is also age conserved.
Based on these findings, we set a new diagnostic criterion for AOD: if the observed mean CCI in a patient is 4 mm or greater, or if the two joints are grossly asymmetric.149 On testing this new criterion in a series of 16 patients with AOD,123 we found that 14 patients had bilateral O-C1 joint widening, with a CCI of 7 to 20 mm (Fig. 224-26), and 2 patients had unilateral joint widening who qualified for the previously unrecognized entity of unilateral AOD (Fig. 224-27). Gross left-right asymmetry was seen in 7 patients, including complete bilateral joint dissociation and lateral shifting of both condyles on C1 (Fig. 224-28) and wide swinging of one condyle off the ipsilateral C1 facet pivoting on the opposite joint (Fig. 224-29). The most revealing findings were three cases in which all four “standard” published tests (Wholey’s, Powers’, Harris’ and Sun’s criteria) gave manifestly normal readings yet the O-C1 joints on both sides were obviously disrupted on reformatted CT and measured emphatically positive with the CCI criterion (Fig. 224-30).
These unexpected findings prompted us to perform a detailed comparison of the diagnostic sensitivity of CCI against the four “standard tests” and other AOD-associated signs such as tectorial membrane damage, perimedullary subarachnoid hemorrhage (SAH), C1-2 epidural blood, and neurological signs of injury to the craniovertebral junction (Fig. 224-31). We found that the CCI was by far the most sensitive (100% sensitivity) index for AOD, with the others, including tectorial membrane tear, all below 70% and all of the “standard tests” trailing even further behind. A test of CCI’s ability to differentiate AOD from non-AOD injuries by using data from five categories of non-AOD upper cervical injuries also shows CCI to have by far the highest specificity among all the other tests.122 There are several explanations for CCI’s supremacy:
Diagnostic Paradigm for Atlanto-occipital Dislocation
The diagnosis of AOD begins with heightened suspicion in a patient with high cervical myelopathy or brainstem deficits (or both) after having suffered high-energy trauma. Perimedullary SAH or C1-2 epidural and subdural blood 122,137 on the head CT scan greatly strengthens the likelihood.123,150–153 Lateral cervical radiography is seldom definitively diagnostic but is helpful if O-C1 distraction is obvious or if there is retropharyngeal edema, which is common in, although not specific to AOD.123,137,138,154–159 Plain radiographs are also important to rule out concomitant subaxial fracture-dislocations.
The presence of any of the aforementioned three warning signs should compel the next level of investigation with thin-cut CT of the upper cervical spine and MRI. An abnormal CCI and conformational disfigurement of the O-C1 joints should be the primary concern on CT, but the lesser “standard tests” should also be performed for corroborative evidence. MRI accurately delineates the extent of ligament damage, as well as the severity of the spinal cord and brainstem injuries, which is of decided prognostic value for functional recovery75 (Fig. 224-32).
In clinical practice, four measurements of the O-C1 joint gap are often not necessary or even feasible to make the diagnosis of AOD. In a widely separated joint, one or two measurements should suffice. If any portion of a joint is wider than 7 mm, it is irrelevant whether any other portion of that joint is more closely apposed. If the joint is so widely dislocated horizontally that it has little or no overlapping surfaces, atlanto-occipital instability can be safely assumed without an actual CCI measurement.123 If the CCIs for both sides are between 3 and 4 mm and there is neither asymmetry nor dislocation but clinical suspicion for AOD is strong, a very careful traction stress test with incremental weights may be performed under fluoroscopy to see whether the CCI widens,154 preferably with the patient awake and under electrophysiologic monitoring.
Management of Atlanto-occipital Dislocation
Once the suspicion of AOD is entertained, however tentatively, traction should immediately be avoided. Traction has been known to worsen neurological deficits and increase O-C1 distraction.152,154,160 Preliminary stabilization can be maintained with a stiff cervical collar and side sand bags while obtaining CT and MRI scans. Excessive bending at the craniovertebral junction must be avoided by carefully placing padding under the shoulders and back to eliminate the torque effect of the prominent occiput.161 After AOD is confirmed by CT and MRI, a halo vest should be fitted to maintain temporary stability while other life-threatening injuries can be dealt with. Because AOD is such an unstable injury and patients are so susceptible to early deterioration, some form of definitive immobilization should be established as soon as it is medically tenable.
Although a few successful cases have been reported with nonsurgical management,111,130,162,163 halo immobilization alone is generally acknowledged to be inadequate for AOD. This conclusion is consistent with the well-known fact that pure ligamentous injuries heal poorly with immobilization.105,164 Moreover, the craniovertebral junction is the least secure site with the halo, and acute slippage of O-C1 alignment has frequently been reported while in the halo.47,103,120,129,165,166 Examples of chronic recurrent O-C1 dislocation causing delayed brainstem compression are known even when initial healing had ostensibly taken place with halo immobilization.109,138,160,167,168
We recommend internal fixation of the occiput to C2 and, in special cases, to C3. The type of O-C1-C2 fixation depends on the patient’s age and the corresponding anatomy of the region relevant to screw purchase, including the thickness of the occipital bone, the size and strength of the lateral mass of C1 and the pars interarticularis (isthmus) of C2, and the adjacency of the vertebral artery to the projected screw paths. Anatomy permitting, screw-plate fixation is biomechanically superior to wire loop techniques whether in providing immediate stability, in resisting loosening of the construct, in preventing vertical settling of the transfixed segments,169,170 or in reducing the number of segments needed to be incorporated into the fusion. Its more solid fixation often dispenses with the postoperative halo, a notable advantage in view of the potential hazards with the halo in children.51,171
Thus, in older children we prefer occipital screw-plate fixation using a C1-2 transarticular screw,172,173 a C2 isthmus screw,174 or the adapted Harms and Melcher technique of a C1 lateral mass screw and C2 isthmus screws.175 Although we usually select children older than 8 years for intra-axial screws, the age limit should be flexible to suit the morphology of the prospective screw paths, which should be preplanned with triplane CT reconstruction through the C2 isthmus and C1 lateral mass. In children, the thickest occipital bone buttress for screw anchorage is usually the midline keel and sometimes near the base of the mastoid.
In young children we still prefer sublaminar wiring,123,176–178 and if the C1 and C2 laminae are exceedingly delicate, as they are in children younger than 3 years, the fusion is extended to C3 or even to C4 to distribute the stress on the uprights, with minimal additional loss of combined segmental motion.51,177 When the occipital squama is too thin for regular screw purchase, we have recently used the “inside-outside” screw technique described by Pait and colleagues179 (see later). A postoperative halo is necessary if sublaminar wiring is used.
Neurological Outcome
AOD has historically been associated with a very poor outcome. Hamilton and Myles found no survivors after AOD in their review of pediatric spinal injuries.15,16 Dickman and coauthors reported 10 deaths in their series of 14 patients, with only 3 patients showing improvement.154 High mortality and severe morbidity are similarly reported by Imaizumi and associates152 and by Saeheng and Phuenpathom,180 whereas the SCI guidelines published jointly by the American Association of Neurological Surgeons and the Congress of Neurological Surgeons158 in 2002 documented poor neurological outcome in 42% and death in 5%. There is no doubt that the prognosis of patients with AOD depends on the severity of the initial neurological injury, in this regard not unlike other categories of pediatric SCI.75 Patients with complete high cord transection or severe head injuries have a very poor outcome,49,114,119,134 and good recovery usually occurs in those with partial or mild initial neurological syndromes.*
Our series documented early and delayed deaths in 19%, complete or severe residual quadriplegia in 12%, but excellent neurological recovery in 69%.123 Overall, our results are considerably better than the historical data. Comparison of the initial and final neurological states in our patients, however, shows no disagreement with the literature for those with initially complete quadriplegia, who tend to remain profoundly disabled. Ventilator-dependent, high-quadriplegic children generally do poorly despite aggressive measures to improve respiratory function, but even in patients with incomplete but severe initial injuries, functional improvements are unfortunately limited.
Atlantoaxial Rotatory Fixation
In normal individuals, the C1-2 joint is responsible for up to 60% of the total axial rotation at the craniocervical region, the atlanto-occipital articulation another 3 to 8 degrees, and the rest shared by the subaxial segments in diminishing measures from C3 downward. The unique configuration of the atlantoaxial complex lends itself to behave as the main rotational pivot of the cervical spine, but these same anatomic features also predispose this complex to a type of rotational deformity termed atlantoaxial rotatory fixation because of its apparent “fixed” state with respect to voluntary or involuntary correction.183 Patients with AARF typically have painful torticollis with the head in the “cock-robin” position (Fig. 224-33): the chin turned toward one side and the neck flexed laterally to the opposite side reminiscent of a robin listening for worms. AARF is most commonly encountered after upper respiratory infections,184–194 trauma,184,190,195–197 and surgery on the head and neck,189,190,197–199 but up to 24% of patients have no obvious cause.186,190 Rheumatoid arthritis, Down syndrome, Morquio’s disease, and an assortment of congenital cervical anomalies have also been associated.185,193,200,201 There is a definite preponderance of AARF in the pediatric population.
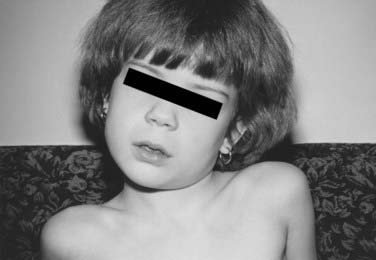
FIGURE 224-33 Typical appearance of the “cock-robin” deformity. The head is rotated to one side and laterally flexed to the opposite side.
The diagnosis of AARF has been the subject of intense debate for many years. Earlier attempts to define a “diagnostic” separation angle between C1 and C2 met with difficulty186,202–205 because pathologic rotatory fixation commonly occurs well within the physiologic range of rotation,206 which also explains why AARF cannot be distinguished from normal rotation on a static radiographic film. Furthermore, attempts to “catch” a fixed C1-2 angle in AARF with motion radiographs were without result because true C1 and C2 interlock without any interbody motion is, in actuality, exceedingly rare and symptomatic AARF patients are much more likely to show preservation of some intersegmental movements but manifest variable degrees of pathologic “stickiness” between C1 and C2 in rotation. The diagnosis of this condition is therefore more aptly founded on abnormal rather than absent relative motion between these two segments.206,207
Functional Anatomy of the Atlantoaxial Joint
A unique feature of the atlantoaxial articulation is that the facets are oriented more horizontally than all the other facet joints of the cervical spine, which have much steeper angles. This nearly (but not completely, an important distinction) horizontal orientation of the C1-2 articulation enables the atlas to rotate on the axis with minimal bony impediment, at least within certain limits. Furthermore, even though the osseous contour of the atlantoaxial joint looks concave on radiography, the padding of the joint cartilage converts the actual articulating surfaces to biconvex disks. Rotation of the atlas pivots about the odontoid process, which acts as an upright post to which most of the stabilizing ligaments within the whole occiput-atlas-axis complex are either attached or intimately related. Of these, the alar ligaments function as the check ligaments against excessive rotation of the atlas on the axis. Traditional teaching asserts that the alar ligaments connect the dorsolateral surfaces of the tip of the dens to the occipital condyles, but there is in fact an important extension of the alar ligament that binds the lateral mass of C1 to the odontoid,208 which may explain more fully the yoking between C1 and C2 in rotation. The thickened capsular ligament aids in restricting rotation and flexion of the atlantoaxial facet joints, but the rudimentary (in humans) apical ligament, the atlantodental ligament, the anterior and posterior atlanto-occipital membranes, and the atlantoaxial membrane all serve minor stabilizing functions.
Motion Analysis and Normal Motion Curve
To study the normal dynamics of C1-2 rotation, thin-cut nonoverlapping axial CT scans were used to determine the C1 and C2 angles in relation to the vertical axis (0 degrees) during a full rotational sweep of the head.183 The separation angle between C1 and C2, the C1-2 angle, is the algebraic difference between the two angles, or C1 − C2. Seven to eight sets of C1 angles and C1-2 angles are obtained with the head turned to different positions from 0 degrees to one side and then turned fully to the opposite side. Plotting the C1 angle on the x-axis, which denotes the head positions, against the C1-2 angle on the y-axis, which represents the C1 − C2 separation angles, into a motion curve thus displays the instantaneous relationship between C1 and C2 in all head positions during a full head swing; that is, the curve accurately defines the rational dynamics between C1 and C2 for the entire physiologic range of head rotation (to both sides). Combining the individual motion curves of multiple subjects into a composite curve by using the sixth-degree “best-fit” polynomial function on the total pool of C1 and C1-2 angles therefore formulates an accurate mathematical and graphic prediction of the entire spectrum of C1 and C2 relationships during normal axial rotation183 (Fig. 224-34).
Figure 224-34 depicts the composite curve of 21 children. It very nearly passes through the zero point, which means that the crossover between C1 and C2 when turning from one side to the other occurs with the head (C1) almost exactly at the vertical 0-degree position (i.e., with the nose straight forward). (Below 0 degrees on the y-axis, the C1-2 angle becomes negative, which means that C1 is on the other side of C2.) This indicates that the vertical 0-degree position is the null point of normal rotation. In addition, the portion of the curve within one quadrant describing motion on one side appears to be the exact mirror image of the curve in the diagonal quadrant depicting motion to the opposite side.
Normal and Abnormal Dynamics of C1-2 Rotation
Based on our motion analysis data,183 the precise events during C1-2 rotation can now be defined in the context of the functional anatomy of the O-C1-C2 joints. There are three distinct regions on the composite motion curve that reflect three distinct phases of C1-2 rotation. With initial head rotation, say to the right, C1 is “cranked” rightward by the tight bony structures of the roller-in-groove joint between its upper facet and the occipital condyle. In contrast, the lax capsular ligaments and the oblique orientation of the alar ligaments allow C2 to be unperturbed for the first 23 degrees or so of head movement. During this first, or single-motion, phase of rotation when C2 remains immobile and only C1 moves, the C1 and C1-2 angles are identical and the curve is perfectly linear with a gradient of exactly 1 (between 0 degrees and the solid arrow in Fig. 224-34). Beyond 23 degrees, the left alar ligament begins to tighten and pulls the odontoid process in the same direction as C1, thus commencing the second, or double-motion, phase of rotation (when both C1 and C2 are moving), in which the C1-2 angle is always less than the C1 angle. The curve begins to arc downward. With continued head turning, the ligaments become progressively more taut. Further C1-2 separation is increasingly impeded, and C2 is pulled more forcefully and faster toward C1 such that even though the C1-2 angle continues to widen, its rate of change is progressively less with each additional degree of C1 rotation. The gradient of the motion curve accordingly becomes less steep as the arc of the curve flattens to a plateau (between the arrows in Fig. 224-34). As the C1 angle approaches 65 degrees, the ligaments and capsular membranes become maximally stretched and the bony contours in the joint surfaces of the axis and atlas probably also reach their contact limits; further widening between C1 and C2 ceases, thus commencing the plateau, or unison-motion, phase of the curve (see Fig. 224-34). The two bones now turn as a couple with a fixed separation angle of about 43 degrees as the head continues to rotate as far as 90 degrees from the vertical 0-degree position. Head turning from 65 degrees onward is carried out by subaxial motion only.
In AARF, the defining pathologic state is therefore not any absolute angle of separation between C1 and C2 but the abnormal way in which C1 and C2 interrelate during axial rotation.206 It is the fluidity of motion and the degree of freedom in individual movements that characterize the severity of the condition. For example, the normal crossover null point is at or very near 0 degrees.183 If on counter-rotation C1 crosses C2 at −15 or −20 degrees from zero on the side opposite the patient’s initial head position, pathologic “stickiness” exists between C1 and C2. Likewise, if on reverse rotation the C1-2 angle narrows much more slowly than normal so that the motion curve is significantly shifted to the left, pathologic stickiness again exists. Certainly, if crossover does not occur at all or, in the extreme case, when the C1-2 angle remains undiminished on forceful counter-rotation, a functional “lock” between the bones has taken place.206
Normal C1-2 rotation does not occur solely and independently in the horizontal plane; “coupling movements” of other types also occur simultaneously. For instance, as the atlas rotates to the left, the orientation of the plane of rotation remains parallel to the horizon only for the first 20 to 25 degrees. Beyond 25 degrees, the right alar ligament begins to tighten, which serves not only to impede further rotation but, by virtue of its expansion to the C1 lateral mass, also to cause the right lateral mass of the atlas to telescope slightly forward and downward. This results in a slight head tilt to the right (completing the full cock robin position). Because the actual articulating surfaces between C1 and C2 are in fact on a slant in the coronal plane and not horizontal, the initial horizontal rotation must at some point encounter resistance. This tilting converts the plane of rotation of the right joint from horizontal to oblique, in better conformity to the angle of the joint surfaces, and this accordingly allows further rotation from 25 degrees to occur up to the expected 43 degrees. It must then follow that from 25 degrees leftward, the primary stress of the rotation is inflicted mainly on the right joint and any bony lock or capsular tear would probably occur in this joint, usually with the lateral mass of C1 popping forward and overhanging C2.183
Diagnostic Motion Study and Classification of Atlantoaxial Rotatory Fixation
By convention, the side toward which the chin is initially pointing is assigned the positive sign. Thus, all C1 angles at the P position are positive, and all angles on the opposite side are negative. The diagnostic motion curve is constructed as for the normal motion curve, except that the three data points from the P, P0, P− scan sequences are simply joined with straight lines. The diagnostic curve is analyzed in the context of normal C1-2 rotation by superimposing it on the physiologic composite motion curve, which is made into a normal template by adding 8 degrees to each side of the mean to account for the variance in distribution in younger subjects with physiologic hypermobile joints.206
Three types of motion curves show flagrant departures from physiologic rotation and can be considered representative of three AARF types.206 The antecedent trauma had evidently resulted in pathologic stickiness that prevents closure of the existing C1 − C2 separation angle by manual counter-rotation. In type I AARF patients, the reduction in the C1-2 angle at the maximum tolerated correction (at P−) is minimal (less than 20% of the C1-2 angle at P); the C1 − C2 separation angles are virtually unchanged even when the head is forcibly rotated way past the midline (Fig. 224-35). All type I motion curves are thus almost straight lines across the right and left upper quadrants of the normal template (Fig. 224-36). Type I AARF represents the abnormal spectrum’s most extreme form, with the two bones locked in the coupled configuration.206
In type II patients, the maximum reduction in the C1-2 angle is greater than 20% of the initial C1-2 angle (at P0), but C1 cannot be made to cross over C2. Their motion curves slope downward from right to left but never traverse the x-axis, where C1-2 crossover normally takes place (Fig. 224-37). Type II AARF patient thus have slightly less severe stickiness than do type I patients in that the initial separation angle is reducible but C1 could not be forced to cross C2.206
In type III patients, the C1-2 angle narrows with correction and C1 can be forced to cross C2 (all C1-2 angles at P− are negative), but always at very abnormal head positions. Their motion curves traverse the x-axis at points where the C1 angle is less than −20 degrees, far left of normal crossover near 0 degrees183 (Fig. 224-38). Type III AARF patients therefore show less stickiness than do the other two types because C1 can be forced to cross C2, but only if the head (or C1) is cranked way past the expected null point at 0 degrees. 206
Thus, the degree of pathologic stickiness in AARF can be graded according to the shape of the motion curve: the more horizontal the curve, the more locked are the two bones. For instant diagnosis and classification of AARF, the user superimposes a patient’s motion curve on the diagnostic domain diagram (Fig. 224-39), whose three diagnostic domains correspond roughly to the areas on the template occupied by the three respective types of curves.
Muscular Torticollis and Diagnostic Gray Zone
Patients with muscular torticollis, despite a painful stiff neck, have no actual injuries to the C1-2 joints and therefore manifest physiologic rotational behavior and have motion curves within the normal range. 206
Some patients have stickier C1-2 rotation than normal, and their motion curves cross the x-axis at the ambiguous zone of −8 to −20 degrees yet do not fulfill our AARF criteria206 (Fig. 224-40). They are designated as being in the diagnostic gray zone, which places them in a “wait-and-see” category regarding rendering of treatment. They are given a cervical collar and analgesics and then reimaged in 10 to 14 days. Many “gray” patients display normal curves on restudy, but some will stay symptomatic with marginal dynamics or even progress to type III AARF. The gray zone thus serves as a therapeutic buffer to avoid overtreatment.
The diagnostic paradigm for AARF based on our imaging criteria is shown in Figure 224-41.
Consequences of Untreated Chronic Atlantoaxial Rotatory Fixation
Neurological deficits are uncommon in patients with acute AARF. Vertebral artery thrombosis secondary to stretch injury may cause intractable vomiting and vertigo or even brainstem deficits, but this is very rare.209 Chronic untreated AARF, however, frequently leads to generalization of painful spasm to both sternomastoids and the posterior nuchal muscles, thus accentuating the fixed lateral head tilt. This severely distorts the head and neck relationship and, together with the cessation of C1-2 rotation, may theoretically accelerate degenerative changes in the subaxial cervical spine.210,211 The facial asymmetry in chronic cases is thought to be due to unrelenting and asymmetric muscle pull on the mandible and other growing maxillofacial structures.210,212 This is at best a cosmetic issue but at worst can lead to disturbed dentition and malocclusion.
Three other changes with chronicity are potentially more sinister206:
Management of Atlantoaxial Rotatory Fixation
Treatment Protocol
Treatment of AARF is rendered in two phases: reduction and stabilization.214 All children with AARF, regardless of the duration of symptoms, are subjected to a course of traction supplemented with benzodiazepines and analgesics. Halter traction is set up for acute and subacute patients. Skull caliper traction is used on chronic patients after our initial abysmal failure with the halter. When the patient’s head returns to the midline, successful reduction is verified with repeat of the CT sequence. If normal dynamics has been restored, the patient goes to the stabilization phase. If complete reduction is not achieved, traction with a halter or skull tongs may be repeated, depending on whether the AARF is type II and type III, or type I, respectively.
After reduction, all acute (treatment delay of less than 1 month) and subacute (treatment delay of 1 to 3 months) patients are immobilized with a Guilford brace for 3 months. The first recurrences are treated by repeat traction, but second recurrences are treated with the halo device for 3 months. Third recurrences and recurrence while in the halo are treated with posterior C1-2 fusion. Chronic patients (treatment delay longer than 3 months) who have been successfully reduced by traction are immobilized with the halo device for 3 months. Failure to reduce or failure to maintain reduction while in the halo is treated by C1-2 fusion.214
Management Outcome
Figure 224-42 compares the course and results of treatment of the three types of AARF.214 Type I AARF patients surpass type II and III patients in every category of management difficulty and have a much poorer prospect for preservation of physiologic rotation. In turn, type II patients also exceed type III patients in the same categories. The differences between types are particularly marked in the proclivity to repeat slippage, the need for multiple invasive procedures, the duration of treatment, and the likelihood of permanent loss of rotation.
Figure 224-43 analyzes the effect of the chronicity of AARF on the course and outcome of treatment. Chronic AARF patients, much more so than acute or even subacute patients, are likely to face a long traction time, suffer repeated slippage, have a greater than 50% rate of nonreduction and fusion, and would very likely end up with absent or permanently restricted rotation at C1 and C2.
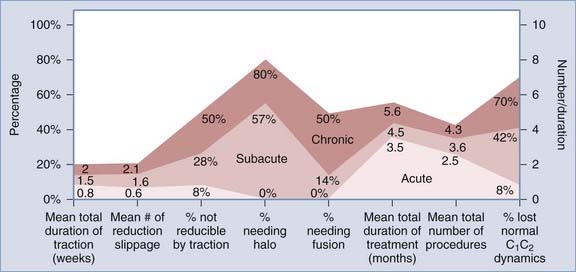
FIGURE 224-43 Comparison of the course and result of treatment between acute, subacute, and chronic atlantoaxial rotatory fixation. The x- and y-axes are identical to those in Figure 224-42. Chronic patients did much more poorly than acute patients in every category. Seventy percent of chronic patients lost normal C1-2 rotatory function through either surgical fusion or permanently impaired dynamics versus only 8% of acute patients. Subacute patients have an intermediate course, but more than 40% had loss of normal C1-2 rotation, thus faring almost as poorly as chronic patients.
Combining the effects of chronicity and severity of AARF, the best and worst outcome classes are undoubtedly chronic type I and acute type III patients, respectively.214
The recommended management algorithm for AARF is summarized in Figure 224-44.
Pathogenesis of Atlantoaxial Rotatory Fixation
In acute AARF, the stickiness is probably caused by some type of interposing soft tissue within the joint that prevents reverse rotation. This may be a piece of torn joint cartilage186,209 or infolding synovium.203 A flap of ripped capsule may be “nipped” by the joint surfaces183 and result in a block, or a corner of the C1 facet could have protruded through a capsular rent and is trapped by the “buttonhole” effect. The anteriorly riding C1 facet regularly loses 85% of its contact surface with C2 at end-rotation.215 During a particularly violent head swing, the C1 facet could have gone completely beyond the contact points with C2 and its inferior lip now jumps over and becomes tightly locked against the front of the C2 facet.203,205,210 C1and C2 will now move ineluctably as a couple on forced counter-rotation (type I AARF), but further rotation in the direction of the displacement could still be possible, more so because the restrictive ligaments and capsules have now been torn or sprained. Traction could disengage some C1-2 interlocks and pull infolding tissues out of joints, but not disimpact a “frozen” C1-2 lock, “undo” the buttonholed facet, or dispel a snugly wedged-in fragment of cartilage.
In long-neglected rotatory fixation, permanent changes occur that would explain the difficulties in reducing chronic AARF. Contractures of joint capsules and periarticular ligaments and tendons,186 osteoarthritis,210 fibrous adhesion bands,216,217 and even osseous cross-unions210,218 between the articulating surfaces have been found during open surgery.
Translational Atlantoaxial Subluxation
In contrast to rotatory fixation, translational atlantoaxial subluxation occurs as a result of violent trauma. The true incidence of this injury is unknown, but it is probably extremely rare, at least among survivors.219–221 Most affected children are in the 0- to 9-year-old group, a group in whom occipitoatlantoaxial injuries are more common.11,14,17,195 The majority of cases result from high-speed pedestrian-vehicular accidents.14,195,222,223 Children who survive the initial moments after injury tend to be seen at two extremes: either they have severe head injury, frequently aggravated by anoxic encephalopathy,87 or they have only minor neck pain, subtle myelopathy, or C2 hypoesthesia. Some cases are not initially diagnosed at all.87,195,222,224–226 Long-term survivors of this injury seldom have severe myelopathy, possibly because of selected survival at the injury scene.
Most cases of translational atlantoaxial subluxation are anterior and result from flexion injuries.227 Exceptions are the very rare posterior C1-2 subluxation caused by hyperextension16,228 and the even rarer C1-2 distraction. The transverse ligament is chiefly responsible for preventing excessive anterior translation of the atlantoaxial joint. Secondary support is provided by the alar ligaments. It is tempting to assume rupture of the transverse ligament as the probable cause of translational C1-2 subluxation, but Adams could find no such correlation in his autopsy study.219 In fact, the most common abnormality in his patients was in the facet joints and their capsules. Braakman and Penning also noted that the dental synchondrosis has less strength than the transverse ligament in children because most children younger than 8 years suffer epiphysiolysis of the odontoid rather than translational C1-2 subluxation.229 If this is true, it would be unusual to encounter isolated rupture of the transverse ligament without dental epiphyseal separation. Therefore, “pure” traumatic translational C1-2 subluxation occurs only under very specific circumstances and probably in the presence of certain predisposing factors.
In most cases, the diagnosis is obvious on the lateral cervical radiograph. In the absence of other fractures, an atlantodental interval greater than 4 mm is abnormal in children.87,223,230,231 The retropharyngeal soft tissues are usually widened. Levine and Edwards reported an avulsed C1 tubercle (where the transverse ligament inserts on the C1 arch) on open-mouth views of the odontoid.232 Occasionally, flexion-extension films are required to detect an otherwise hidden instability. Although immobilization alone has been tried for translational C1-2 subluxation,191,222,224,226 it is generally thought that extensive ligamentous injury requires C1-2 fusion.87,223,232
Special Considerations
Approximately 20% of patients with trisomy 21 (Down’s syndrome) have atlantoaxial instability.233 Among these patients, there are reports of atlanto-occipital dislocation,93,234,235 atlantoaxial instability,91,234,236,237 and AARF.200 Because a child with Down’s syndrome has more lax ligaments and a higher incidence of vertebral anomalies that predispose the cord to injury,92,93,236,238–240 the index of suspicion for SCI after trauma should be raised in these children. Atlantoaxial instability has also been reported in patients with dens anomalies (e.g., os odontoideum and odontoid hypoplasia), juvenile rheumatoid arthritis, Morquio’s syndrome, Scott’s syndrome, pseudoachondroplasia, spondyloepiphyseal dysplasia, spondylometaphyseal dysplasia, and cartilage-hair hypoplasia.241–245 Special consideration should be given to investigating the spine and spinal cord in these patients, even after what would usually be considered trivial trauma.
Spinal Stabilization in Children: Problems and Pitfalls
Problems Related to Immediate Stabilization
The application of skull caliper traction is still the simplest and most effective method of immediate stabilization, but it must be modified according to the age of the child.51 Caliper traction is not recommended for children younger than 4 years because it is liable to cause ping-pong or other depressed skull fractures. Children in traction also tend to thrash about in bed and are at higher risk than if a one-piece orthosis is worn. Thus, for children between 1 and 4 years of age, the halo device is used for immediate stabilization. If the unstable segments in these young children are grossly malaligned, especially when the cord injury is incomplete, the segments should be reduced by fluoroscopically guided manual manipulation under general anesthesia, preferably with electrophysiologic monitoring. Afterward, fine-tuning and maintenance of the reduction can be achieved by adjusting the halo ring. If closed reduction is unsuccessful, the surgeon should proceed with open reduction and simultaneous fusion.
Skull caliper traction can be used safely on children from 5 to 12 years old, but with a modified protocol. First, strong sedatives are administered to contain body motions and relax the nuchal muscles. Second, in young children, the forces required for reduction are much less than those needed for adults, and the degree of ligamentous instability is often underestimated. The traction weight must therefore be reduced from the usual 5 lb per level in adults to 2 or 3 lb per level with increments of 2 lb, up to a maximum of 25% of the child’s body weight. Each increment in weight must be monitored with a lateral x-ray film to guard against overdistraction.51 Finally, we recommend the pediatric RotoRest bed (Kinetic Concepts, Inc., San Antonio, TX), in which the entire body can be fit snugly between adjustable side panels so that the child feels more secure and is less disposed to thrash about. Upward sliding of the body is checked by well-padded shoulder stoppers.51 The gentle rotation of the RotoRest bed is also helpful for reducing skin complications, which can develop rapidly in children.
Problems with External Orthoses
Halo Fixation Device
Among all the available cervical orthoses, the halo vest provides the most rigid immobilization of the cervical spine in children.51,94,228,246–248 It also permits the most precise positioning of the neck to achieve and maintain alignment. It is the only external orthosis that does not have a chin support, so it interferes the least with mandibular motion and eating.249 Early mobilization enables these children to participate in rehabilitation.250,251 In our experience, children tend to sabotage the immobilization if they are not allowed some physical activity. However, the rate of complications associated with the halo is also higher in children than in adults,250 and most are related to the thinness of the child’s calvaria. The anterior pins should be placed immediately above the eyebrows to take advantage of the thick lateral supraorbital buttress250 and laterally to avoid injuring the supraorbital and supratrochlear nerves. The torque used for pin tightening should be between 2 and 4 inch-lb, depending on the size of the child. For children 10 months to 3 years of age, “tiny tot” haloes are available with a small ring and up to 10 small pins that should be only finger-tightened.252 However, one great disadvantage of the multipin system is that it leaves few unused holes on the ring for pin changes in the event of pin site infection or loosening (see later).
The frequency of pin loosening is much higher in children than in adults.250,253 Some pins migrate cephalad because they were inserted above the skull equator, where the contour of the skull is upward sloping. Pins may also loosen because the correct degree of tightness was not rechecked. As a result of the viscoelastic properties of the skull, the pins frequently loosen up to 4 inch-lb after the vest and uprights are attached to the ring, and they must be retightened to the correct torque 24 hours later. If a loose pin is discovered 2 to 3 weeks after insertion, management depends on the age of the patient. Garfin and colleagues found that with 6 inch-lb of torque, the halo pin only partially penetrates the outer table of the adult skull and there is usually a solid margin of cortical bone to allow safe retightening.253 Thus, it is usually safe to retighten loose pins in older children, provided that definite resistance can be felt on tightening. The skull tables in young children are much thinner. Accelerated osteoclasis around the pin site softens the bone so much that retightening can cause full-thickness penetration and an intracranial abscess.254 Loose pins should be replaced and not tightened in these children.51
Pin site infections are also much more common in children than in adults. Baum and coworkers reported a 31% incidence of pin infection in children versus 6% in adults.250 The pin sites should be cleansed thoroughly with 10% hydrogen peroxide solution 4 times daily, and all scabs and crust around the pins must be removed to allow drainage. If the infection is mild and superficial, with only minimal surrounding scalp erythema and drainage, and the pin remains tight, oral antibiotics are prescribed. If the scalp is red and swollen, the drainage becomes copious and purulent, or the child complains of constant local pain or headaches, the pin should be removed and intravenous antibiotics administered to prevent the dreaded cranial osteomyelitis. A CT scan should be performed to rule out an intracranial abscess.254–257
Children in haloes also have a greater likelihood than adults to show slippage of alignment, partly because of unrecognized pin loosening and partly because children do not protect their haloes. Direct contact of the ring and posterior uprights with the bedding should be avoided to minimize transmitted flexion stress. We use a large foam wedge to prop up the back of the vest so that the child can recline comfortably with the head and halo ring hanging free and still be able to watch television, read, play board games, and self-feed.51 In addition, children sometimes undergo rapid and drastic changes in body weight and contour after SCI, so the original vest may become ill fitting and need to be recustomized.
Minerva Cast
On rare occasion, the Minerva cast has been used on young children who do not tolerate the halo pins. The thermoplastic jacket is constructed of Polyform, a splinting material made from the polyester polycaprolactone, and Polycushion, a closed-cell foam for padding.258 This lightweight material solves the problems of hyperthermia and encumbered ambulation. Clinical trials have also found that the thermoplastic Minerva jacket actually restricts intersegmental “snaking” movements in the cervical spine better than the halo vest does. It deserves further evaluation for adaptation for use in children.
Guilford Brace
The Guilford brace has been used extensively in our institution on children with SCIWORA.19,27,69,252 This brace has padded mandibular and occipital supports that interlock to form a rigid metal ring below the ears. The ring is connected by strong metal strips to anterior and posterior thoracic plates that are attached to each other with shoulder and axillary straps. The Guilford brace is comparable to other cervicothoracic braces in its ability to limit flexion, extension, and rotation, especially of the mid to lower cervical segments.228,247 Like the other orthoses, the Guilford brace is not as effective in controlling lateral bending. Its main advantage over other braces is that it can be custom-made within 24 hours to fit any body size down to that of a 1-year-old. With deepening of the chin and occipital plates to restrict rotation, it is also suited for maintaining reduction in patients with AARF.
Molded Body Splint
There is no ideal orthosis for long-term immobilization of infants with grossly unstable necks. One example of this condition is diastrophic dwarfism, in which affected infants have ligamentous laxity at multiple segments and are usually seen in the first few months of life with apnea and quadriplegic spells because of multilevel subluxation and cervicomedullary injury. These neurological manifestations are completely reversible as long as the neck can be kept in a neutral or slightly extended position. Because no orthosis can reliably eliminate intersegmental motions in these infants, wiring and bone graft fusion often end in nonunion, and we usually do not attempt surgical fusion until these patients reach 12 to 18 months of age. In the interim, the infant is immobilized in a thermoplastic body and head splint molded to the contour of the thorax, head, and neck in the desired degree of extension.51 The body section incorporates the pelvis and hip joints and has a crotch strap that interlocks with cross-shoulder straps over the chest and abdomen, based on the same principle as an infant car seat. The head section has a padded headband that is strapped across the forehead to secure the head. The back slab can be fenestrated if hyperthermia develops, which happens not infrequently (Fig. 224-45). Diastrophic dwarfs are not usually ambulatory for many months after birth anyway, and with proper skin care, they can be kept safely in this molded body splint for up to 3 years (Fig. 224-46), at which time extensive surgical fusion has a better chance of success.
Problems Related to Internal Surgical Fixation
General Principles
It is common practice at many SCI centers to intubate adult patients with unstable injuries while they are awake so that any sudden changes in the patient’s neurological status can be recognized. Awake intubation is seldom tolerated by children. Young children, in particular, tend to resist vigorously and may actually jeopardize the unstable injury further. We prefer to perform fiberoptic nasotracheal intubation on children after induction of general anesthesia with intravenous thiopental and vecuronium bromide. In this manner, the airway can be quickly and atraumatically secured with minimal head movement. Spinal cord function is monitored with real-time SSEPs throughout intubation, halo application, and the surgical fusion that follows.51
It is our impression and that of others that bone bank bone has less chance than autologous bone grafts of inducing successful fusion in children.259 The amount of available bone is obviously limited by the size of the child, and both iliac crests should be prepared for use. Other bone sources include ribs, fibula, and split-thickness cranial bone from the occiput.260
Permanent stability at the fusion site ultimately depends on the amount of new bone that manages to form across the fusion surfaces. The larger the fusion surface available for new bone formation, the more exuberant the callus and the stronger the subsequent bony bridge. Although methyl methacrylate incorporated into a wiring construct enhances the immediate stability of the construct,261 it also diminishes the available fusion surface for new bone formation and ultimately weakens the permanent strength of the fusion.262 This is a particularly relevant issue in children, in whom the fusion surface is already limited. Moreover, acrylic does not bind to bone, weakens with time, and remains a permanent foreign body.263,264 Methyl methacrylate is therefore not used in children except when a malignancy is involved.
Continuous micromotion between fusion segments is strongly correlated with nonunion. As mentioned, halo immobilization in children is sometimes complicated by pin loosening and slippage in position. Non–halo-type orthoses provide even less satisfactory external stabilization.228 Thus, for children with unstable injuries, the burden of immediate stabilization rests largely on the immediate strength of the fusion construct; this is in contrast to adult patients, in whom the halo can be depended on to provide more reliable external immobilization. Thus, one should always select the construct that gives maximal immediate internal stability, that is, the one that is most effective in eliminating intersegmental motion. Fusion techniques for children should be judged on this important principle.
Injuries Involving C2-7
For unstable subaxial injuries in children 1 to 5 years of age, we favor the sublaminar wiring technique.51 Braided, MRI-compatible titanium Codman or Songer cables are used because their malleability, sliding quality, and crimp-lock mechanism enable safe passage and strenuous tightening. For very young children with delicate dura, Codman cables are preferred because of their soft pliability. Iliac crest bone grafts are then secondarily wired to the fusion surface or tightly pressed down against it by strapping sutures strung across the deep muscles. This extremely strong and stable construct ensures a high rate of union in children. Regardless of the age of the child, the laminae are still the strongest bone buttress to support any wiring. Even in children 1 to 3 years old, 18-gauge cables can be used without producing laminar fracture. Tightening the cables usually makes the two adjacent laminae crunch against each other to produce a more stable friction block than either the interfacetal or interspinous wiring techniques.261
Posterior Mid to Lower Cervical Fusion with Instrumentation
The ultimate challenge lies in attempting to stabilize a young child with kyphotic instability after extensive laminectomy. Not only are the fusion surfaces drastically reduced, but the best support for the wiring, the laminae, are also gone. The use of interfacetal wiring or facetal strut grafts is limited by the fragility of the facets in young children. For children younger than 5 years, we use a contoured Luque rectangle with the top and bottom bars fastened to one or two intact laminae at each end of the laminectomy defect, but the fusion necessarily includes at least two extra levels beyond the laminectomy defect. For older children, the best alternative is lateral mass screws and plates.265 These posterior plates were not used extensively in children because of the variable size of their articular pillars and the closeness of the vertebral artery to the screw path, but recently available thinner and shorter screws have broadened their use in children, albeit selectively, depending on the size of the vertebrae and the age of the child. They are the preferred technique because the resulting rigid construct dispenses with the need for the halo.
Injuries Involving the C1-2 Joint
Different fusion techniques are used for the atlantoaxial articulation because of its unique anatomic and biomechanical characteristics. For example, the atlas does not have a spinous process for interspinous wiring. Because of the wide gap between the posterior arches of C1 and C2, simple sublaminar wiring does not impart stability in extension unless the two arches are actually made to approximate. Moreover, the inclination of the atlantoaxial facet joints and the interfacetal ligaments is teleologically “suited” to a 45-degree range of axial rotation96,257 and a high degree of translational freedom. Any fusion technique for this extremely mobile joint must therefore withstand all four planes of motion—flexion, extension, rotation, and translation.
The Gallie method, which uses a single midline wire loop around the arch of C1 and the spinous process of C2, does not meet the desired objectives because it eliminates neither extension nor rotation.266 In contrast, the Brooks fusion uses bilateral wires passed around the arch of C1 and the lamina of C2 and then tightened separately over a corticocancellous bone graft compressed against the C1 and C2 rings.111 A modification of the Brooks technique by Griswold and colleagues involves the use of an almost T-shaped bone graft wedged between the C1 and C2 bony rings; the flat part of the T prevents the graft from being displaced into the spinal canal.267 With the modified Brooks construct, there is stability in both flexion and extension: flexion is eliminated by the wires, and extension is stopped by the buttressing bone graft,96 which also serves as a friction block between the two bony arches and offers stability against axial rotation and translation. The modified Brooks fusion is very effective in children with atlantoaxial instability, especially when the C1 arch is fixed in an upward tilt that leaves a wide gap between it and the C2 neural arch (Fig. 224-47). A newer modification of the Brooks method in which two separate interposing bone grafts are used, one on each side of the midline, allows the Brooks principle to be used in cases in which the middle third of the C1 arch has to be removed for decompression, such as when C1-2 realignment is less than perfect.51,268
There is, however, a caveat when using the Brooks method in young children. It seems that the incidence of rapid graft absorption is higher in young children than in adults, possibly because halo fixation in young children does not provide the same degree of stability and the continued micromotion at the fusion site promotes graft resorption and retards bone formation, thereby ultimately leading to graft shrinkage, which then loosens the wire loops.51 Thus, in children younger than 3 or 4 years, we prefer to pass bilateral sublaminar wires around C1 and C2 and then tighten them until the two arches touch.20,51 This is quite easily and safely achieved in most young children without placing excessive strain on the arch of C1 (Fig. 224-48). The conjoined bony rings offer stability in both flexion and extension, and the two-point fixation provided by the double wires also increases friction between the twin rings and reduces axial rotation and translation. On-laid bone grafts are then compressed tightly against the fusion surface with deep sutures. The inherent strength of this construct is undiminished by any interim changes in the grafts, and fusion is almost guaranteed as long as the bone rings and wires hold up against breakage.
Atlantoaxial Fusion with Instrumentation
In older children, other options are available for instrumentation of the C1-2 joint. Magerl pioneered the use of transarticular screw fixation of C1-2 in 1979, and he and Seemann reported their experience in a large series of adults in 1986.172 Modern adaptations of the original technique using newer drill assemblies, precise planning of the trajectory, and guidance with frameless stereotactic navigation have been described.269 The chief advantages of this technique include excellent fixation against translation and rotation; when supplemented with an interposing bone graft between the arches of C1 and C2, which prevents flexion and extension, use of the halo postoperatively can be avoided.270 In addition, it is useful even when the arches of C1 or C2 have been removed for decompression. Reported successful fusion rates are 95% to 98%,271,272 as compared with 80% to 86% when wire-graft techniques are used with the halo.268,271 Complication rates for vertebral artery injury, ischemic stroke, and neural injury are exceedingly low when proper precautions are taken.269,271–273 With the addition of bilateral vertical plates, the C1-2 transarticular screw construct can be extended to incorporate lateral mass screw fixation of the subaxial spine.
The bulk of the literature on C1-2 transarticular screw fixation concerns adults. In the last 5 years, this technique has been applied to an increasing number of children, and emerging data suggest great promise (Fig. 224-49). Wang and colleagues reported a series of 13 children (the youngest being 4 years old) with a 100% fusion rate, no complications, and no postoperative halo use,274 as opposed to an 84% fusion rate with a wire-graft method and halo reported by others.177 Recently, Gluf and Brockmeyer reported equally good results in 67 children, and their experience and insight have underscored a number of special precautions in children that must be heeded.275
In some adults, the bony groove for the vertebral artery at C2 may be so large that the width of the pars interarticularis (isthmus) is reduced to below safety limits for passage of a 3.5-mm screw. Madawi and associates276 and Paramore and coworkers277 reported a 20% exclusion rate for at least one isthmus because of the vertebral artery anatomy, and in 4% to 5%, neither side was deemed safe. The isthmus in children is accordingly narrower than in adults (Fig. 224-50). Especially in younger children, the absolute dimension of the isthmus must be premeasured and the screw path preplanned on a virtual image before surgery. Triplane computer reconstruction with the cursor traversing the long axis of the isthmus (simulating the screw path) should be done on every child. If only unilateral screw placement is feasible, a postoperative halo should be used. Madawi and associates reported a higher incidence of screw malpositioning if the C1-2 subluxation is poorly reduced with considerable offset in the facet contact surfaces.276,278 Good alignment is therefore very important in pediatric patients.
Occipital-Cervical Fusion with Instrumentation
Occasionally, an anteriorly dislocated C1 arch cannot be reduced by traction or manipulation. This happens in some cases of os odontoideum, chronic posttraumatic anterolisthesis, and several forms of mucolipidosis in which abnormal tissues are interposed between the dens and the anterior arch of C1. Sublaminar wire fusion of the unreduced ring of C1-2 may add to the spinal cord compression. In such instances, it is safer to remove the arch of C1 to decompress the cord before attempting to fuse the occiput, C2, and C3 together. For this situation and for the progressive kyphosis sometimes seen after occipitocervical decompression for Chiari malformation, we use a Steinmann pin shaped in an inverted U with the bend contoured to conform to the occipital boss.51 The two vertical bars are wired to the top two available laminae, and the curved U bend is wired to the occiput through bur holes on both sides of the midline (Fig. 224-51). Use of the Luque rectangle adds a measure of inferior stability by fixing the lower rim of the rectangle to the lower intact lamina (Fig. 224-52).20 Cancellous bone grafts are packed into the lateral gutters at C1, on the decorticated laminae, and on the occipital squama.
The chief disadvantage of the rigid U-bar technique is that sliding motion between the metal bar and the metallic cables cannot be totally eliminated. Completely rigid fixation is not obtained, and because of the great angular momentum of the child’s heavy head working against the cable-bar contact, a halo vest is always recommended postoperatively. Plates have been affixed more rigidly with screws to the occiput and the cervical spine,279–288 but with children, bone thickness of the occiput is of great concern in terms of both providing adequate bony purchase for the traditional outside-to-inside screws and avoiding penetration of the dura. In fact, the unevenness of bone thickness in the occipital squama of a child is such that screw purchase is secure only into the midline keel and far laterally near the mastoid process.289
Pait and colleagues described the “inside-outside” technique, which obviates most of the problems related to screw purchase in thin skulls and is eminently suitable for occipital-cervical fusion in children.179 In this technique, a lateral mass plate is contoured to fit the shape of the occiput and the cervical spine, and the plate is affixed to the occiput by means of a flat-headed screw placed through a bur hole in the calvaria so that the head of the screw is in the epidural space and the threads face outward—hence the sobriquet “inside-outside.” The burr hole marks the “entry site” of the inside-outside screw. After the dura is separated from the inner table of the skull, a “keyhole” is cut with a craniotome foot-plated saw blade from the entry site some distance away to the premarked “exit site” for the screw. The size of the exit site is cut to the size of the screw stem, always much smaller than the burr hole. The inside-outside screw is attached to the guide rod (Dyna-Lok T-bolt and nut; Sofamor Danek, Memphis, TN) and then carefully placed epidurally into the entry site and guided to the exit site (Fig. 224-53A). The reconstruction plate is placed “through” the outpointing screw stem, and a nut is semitightened on the exposed screw threads with a socket wrench (see Fig. 224-53B). After the C1-2 transarticular screw (or C2 pedicle screw) and all the subaxial cervical lateral mass screws have been inserted and tightened on the plate, the occipital inside-outside screw is tightened firmly against the nut with the socket wrench while a screwdriver is inserted through the socket wrench into the hex in the hollow inside-outside screw to provide counter-torque (see Fig. 224-53C).
Different plate systems can be linked to the inside-outside screw, depending on the size and age of the child. Children as young as 2 years have been treated with AO/Synthes Posterior Cervical Plates (Synthes Spine, Paoli, PA), but with this plate system, the locations of the apertures for the cervical screws are restricted. With the newer Star Lock CerviFix System, the screw heads on the cervical spine are attached to the vertical bars via sliding links. The inside-outside screws, as well as all the cervical screws, can be placed individually before application of the vertical elements and final adjustment of alignment, thereby eliminating a great deal of the “fidget factor” of the procedure (Fig. 224-54). With this technique, children as young as 12 months can safely be instrumented for occipital C1-2 fusion. The thinness of the occipital bone in young children can be compensated for by choosing screw heads with larger diameters to distribute the crushing forces. A modified axis plate with a custom-tapered perforated vertical stem (Sofamor Danek, Memphis, TN) is designed especially for sublaminar wiring to small delicate laminae (Figs. 224-55 and 224-56). Postoperative halo devices are not usually necessary except for the very young, when redistribution of stress from the fragile laminae to the external orthosis is still necessary. Preliminary fusion rates are very promising.123,179
Problems with Fusion Extension
Unintentional inclusion of adjacent “bystander” vertebrae into the fusion mass sometimes occurs in children.89 Bystander fusion can include the entire neck, even though the bone grafts were originally laid down over just one or two levels.51,86,89 The process appears to be related to a combination of overzealous subperiosteal dissection of the bystander laminae and spinous process and denudation of the cortical bone, migration of the bone grafts into adjacent sites, and rigid immobilization of the entire cervical spine. Children have a greater capacity than adults to form exuberant callus whenever the periosteum is breached and the neck is effectively immobilized. Migration of bone grafts seems to be less important because as long as the periosteum is intact, stray grafts do not promote bystander fusion.
The obvious disadvantage of overfusion is unnecessary loss of mobility, but there is also a theoretical threat of accelerated spondylogenesis in the remaining mobile segments that are now burdened with excessive motion and stress. The two factors are directly related: the greater the combined loss of mobility at the fusion, the greater the compensatory increase in stress at the remaining mobile segments. Fusion of a single level below C2 results in the loss of 8 to 17 degrees of flexion-extension and 8 to 12 degrees of axial rotation, depending on the level and age of the patient.96,290 Inadvertent inclusion of the occiput into an atlantoaxial fusion means the loss of 13 degrees of flexion-extension at the O-C1 joint, in addition to the loss of 45 degrees of axial rotation at the C1-2 joint (>50% of total axial rotation).96,183 Both situations represent a potential handicap and should be avoided. The most effective preventive measure is meticulous preservation of the periosteum and other soft tissues overlying the bony surfaces adjacent to the intended fusion surface.
Aufdermaur M. Spinal injuries in juveniles: necropsy findings in twelve cases. J Bone Joint Surg Br. 1974;56:513-519.
Brockmeyer DL, Apfelbaum RI. A new occipitocervical fusion construct in pediatric patients with occipitocervical instability. J Neurosurg Spine. 1999;90:271-275.
Grabb PA, Pang D. Magnetic resonance imaging in the evaluation of spinal cord injury without radiographic abnormality (SCIWORA) in children. Neurosurgery. 1994;35:406-414.
Hamilton MG, Myles ST. Pediatric spinal injury: review of 174 hospital admissions. J Neurosurg. 1992;77:700-704.
Hulbert RJ, Crawford NR, Choi WG, et al. A biomechanical evaluation of occipitocervical instrumentation: screw compared with wire fixation. J Neurosurg Spine. 1999;90:84-90.
Magerl F, Seemann PS. Stable posterior fusion of the atlas and axis by transarticular screw fixation. In: Kehr P, Weidner A, editors. Cervical Spine. Berlin: Springer-Verlag; 1986:322-327.
Pait TG, Al-Mefty O, Boop FA, et al. Inside-outside technique for posterior occipitocervical spine instrumentation and stabilization: preliminary results. J Neurosurg Spine. 1999;90:1-7.
Pang D. Spinal cord injury without radiographic abnormalities in children, 2 decades later. Neurosurgery. 2004;55:1325-1343.
Pang D. Principles and pitfalls of spinal stabilization in children. In: Pang D, editor. Disorders of the Paediatric Spine. New York: Raven Press; 1995:575-604.
Pang D, Li V. Atlanto-axial rotatory fixation Part III: a prospective study of the clinical manifestation, diagnosis, management and outcome of children with atlanto-axial rotatory fixation. Neurosurgery. 2005;57:954-972.
Pang D, Li V. Atlanto-axial rotatory fixation Part II: new diagnostic paradigm and a new classification based on motion analysis using computed tomographic imaging. Neurosurgery. 2005;57:941-953.
Pang D, Li V. Atlanto-axial rotatory fixation Part I: biomechanics of normal rotation at the alanto-axial joint in children. Neurosurgery. 2004;55:614-626.
Pang D, Nemzek WR, Zovickian J. Atlanto-occipital dislocation. Part 1: normal occipital condyle-C1 interval in 89 children. Neurosurgery. 2007;61:514-521.
Pang D, Pollack IF. Spinal cord injury without radiographic abnormality in children—the SCIWORA syndrome. J Trauma. 1989;29:654-664.
Pang D, Wilberger JE. Spinal cord injury without radiographic abnormalities in children. J Neurosurg. 1982;57:114-129.
Pollack IF, Pang D, Hall WA. Subarachnoid pleural and subarachnoid-mediastinal fistulae. Neurosurgery. 1990;26:519-525.
Pollack IF, Pang D, Sclabassi R. Recurrent spinal cord injury without radiographic abnormalities in children. J Neurosurg. 1988;69:177-182.
Pueschel S, Scola FH. Atlanto-axial instability in individuals with Down’s syndrome: epidemiologic, radiographic and clinical studies. Pediatrics. 1987;80:555-560.
Ruge JR, Sinson GP, McLone DG, et al. Pediatric spinal injury: the very young. J Neurosurg. 1988;68:25-30.
Sonntag VK, Dickman CA. Craniocervical stabilization. Clin Neurosurg. 1993;40:243-272.
Sudeck P. Uber Drchungsverrenkung des Atlas. Dtsch Z Chir. 1923;183:289-303.
Sullivan CR, Bruwer AJ, Harris E. Hypermobility of the cervical spine in children: a pitfall in the diagnosis of cervical dislocation. Am J Surg. 1958;95:636-640.
Taylor AR, Blackwood W. Paraplegia in hyperextension cervical injuries with normal radiographic appearances. J Bone Joint Surg Br. 1948;30:245-248.
Villas C, Arriagada C, Zubieta JL. Preliminary CT study of C1-C2 rotational mobility in normal subjects. Eur Spine J. 1999;8:223-228.
White AA, Panjabi MM. The basic kinematics of the human spine. Spine. 1978;3:12-20.
1 Anderson M, Schutt A. Spinal injury in children: a review of 156 cases seen from 1950 through 1978. Mayo Clin Proc. 1980;55:499-504.
2 Andrews L, Jung S. Spinal cord injuries in children in British Columbia. Paraplegia. 1979;17:442-451.
3 Burke DC. Spinal cord trauma in children. Paraplegia. 1971;9:1-14.
4 Geisler FH, Dorsey FC, Coleman WP. Recovery of motor function after spinal cord injury—a randomized, placebo-controlled trial with GM-1 ganglioside. N Engl J Med. 1991;324:1829-1838.
5 Hadley MN, Sonntag VKH, Rekate HL. Pediatric vertebral column and spinal cord injuries. Contemp Neurosurg. 1988;10:1-6.
6 Henrys P, Lye ED, Lifton C, et al. Clinical review of cervical spine injuries in children. Clin Orthop Relat Res. 1977;129:172-176.
7 Kewalramani LS, Kraus JF, Sterling HM. Acute spinal cord lesions in a pediatric population: epidemiological and clinical features. Paraplegia. 1980;18:206-219.
8 Kewalramani LS, Tori JA. Spinal cord trauma in children: neurologic patterns, radiologic features, and pathomechanics of injury. Spine. 1980;5:11-18.
9 Melzak J. Paraplegia among children. Lancet. 1969;2:45-48.
10 Mendelsohn DB, Zollars L, Weatherall, et al. MR of cord transection. J Comput Assist Tomogr. 1990;14:909-911.
11 Ruge JR, Sinson GP, McLone DG, et al. Pediatric spinal injury: the very young. J Neurosurg. 1988;68:25-30.
12 Schaeffer DM, Flanders AE, Osterholm JL, et al. Prognostic significance of magnetic resonance imaging in the acute phase of cervical spine injury. J Neurosurg. 1992;76:218-223.
13 Wilberger JEJr. Spinal Cord Injuries in Children. Mount Kisco, NY: Futura; 1986.
14 Hadley MN, Zabramski JM, Browner CM, et al. Pediatric spinal trauma: review of 122 cases of spinal cord and vertebral column injuries. J Neurosurg. 1988;68:18-24.
15 Hamilton MG, Myles ST. Pediatric spinal injury: review of 61 deaths. J Neurosurg. 1992;77:705-708.
16 Hamilton MG, Myles ST. Pediatric spinal injury: review of 174 hospital admissions. J Neurosurg. 1992;77:700-704.
17 Hill SA, Miller CA, Kosnik EJ, et al. Pediatric neck injuries: a clinical study. J Neurosurg. 1984;60:700-706.
18 Osenbach RK, Menezes AH. Spinal cord injury without radiographic abnormality in children. Pediatr Neurosci. 1989;15:168-175.
19 Pang D. Spinal cord injury without radiographic abnormality (SCIWORA) in children. In: Betz R, editor. The Child with a Spinal Cord Injury. Rosemont, IL: American Academy of Orthopedic Surgeons and Shriners Hospital; 1996:139-160.
20 Pang D. Pediatric spinal cord injuries. In: McLone DG, editor. Paediatric Neurosurgery. 4th ed. Philadelphia: WB Saunders; 2001:660-694.
21 Bailey D. The normal cervical spine in infants and children. Radiology. 1952;59:712-719.
22 Fesmire FM, Luten RC. The pediatric cervical spine: developmental anatomy and clinical aspects. J Emerg Med. 1989;7:133-142.
23 Sullivan CR, Bruwer AJ, Harris E. Hypermobility of the cervical spine in children: a pitfall in the diagnosis of cervical dislocation. Am J Surg. 1958;95:636-640.
24 Townsend EHJr, Rowe ML. Mobility of the upper cervical spine in health and disease. Pediatrics. 1952;10:567-573.
25 Von Torklus D, Gehle W. The Upper Cervical Spine. New York: Grune & Stratton; 1972.
26 Cattell HS, Filtzer DL. Pseudosubluxation and other normal variations in the cervical spine in children: a study of one hundred and sixty children. J Bone Joint Surg Am. 1965;47:1295-1309.
27 Pang D, Pollack IF. Spinal cord injury without radiographic abnormality in children—the SCIWORA syndrome. J Trauma. 1989;29:654-664.
28 Papvasiliou V. Traumatic subluxation of the cervical spine during childhood. Orthop Clin North Am. 1978;9:945-954.
29 Sherk HH, Schut L, Lane JM. Fractures and dislocations of the cervical spine in children. Orthop Clin North Am. 1976;7:593-604.
30 Kalfas I, Wilberger J, Goldberg A, et al. Magnetic resonance imaging in acute spinal cord trauma. Neurosurgery. 1988;23:295-299.
31 Braakman R, Penning L. Injuries of the Cervical Spine. Amsterdam: Excerpta Medica; 1971.
32 Todury G. The cervical spine: its development and changes during life. Acta Orthop Belg. 1959;25:602-607.
33 Aufdermaur M. Spinal injuries in juveniles: necropsy findings in twelve cases. J Bone Joint Surg Br. 1974;56:513-519.
34 Pang D, Wilberger JE. Spinal cord injury without radiographic abnormalities in children. J Neurosurg. 1982;57:114-129.
35 Gilles FH, Bina M, Sotrel A. Infantile atlantooccipital instability: the potential danger of extreme extension. Am J Dis Child. 1979;133:30-37.
36 Scher AT. Anterior cervical subluxation: an unstable position. AJR Am J Roentgenol. 1979;133:275-280.
37 Webb JK, Broughton RBK, McSweeney T, et al. Hidden flexion injury of the cervical spine. J Bone Joint Surg Br. 1976;58:322-327.
38 Baker D, Berdon W. Special trauma problems in children. Radiol Clin North Am. 1966;4:289-305.
39 Braakman R, Penning L. The hyperflexion sprain of the cervical spine. Radiol Clin North Am. 1968;37:309-320.
40 Osenbach RK, Menezes AH. Pediatric spinal cord and vertebral column injury. Neurosurgery. 1992;30:385-390.
41 Frankel HL, Hancock DO, Hyslop G, et al. The value of postural reduction in the initial management of closed injuries of the spine with paraplegia and tetraplegia. Paraplegia. 1969;7:179-192.
42 Burke DC. Traumatic spinal paralysis in children. Paraplegia. 1974;11:268-276.
43 Choi JU, Hoffman HJ, Hendrick EB, et al. Traumatic infarction of the spinal cord in children. J Neurosurg. 1986;65:608-610.
44 Hachen HJ. Spinal cord injury in children and adolescents. Paraplegia. 1977;16:55-64.
45 Hasue M, Hoshino R, Omata S, et al. Cervical spine injuries in children. Fukushima J Med Sci. 1974;20:115-123.
46 Yngve DA, Harris WP, Herndon WA, et al. Spinal cord injury without osseous spine fracture. J Pediatr Orthop. 1988;8:153-159.
47 Rockswald GL, Seljeskog EL. Traumatic atlantocranial dislocation with survival. Minn Med. 1979;62:151-154.
48 Fielding J. Injuries of the cervical spine in children. In: Rockwood CAJr, King RE, editors. Fracture in Children, vol 3. Philadelphia: JB Lippincott; 1984:683-705.
49 Kaufman RA, Carrol CD, Buncher CR. Atlantooccipital junction: standards for measurement in normal children. AJNR Am J Neuroradiol. 1987;8:995-999.
50 Zabramski JM, Hadley MN, Browner CM, et al. Pediatric spinal cord and vertebral column injuries. BNI Q. 1986;2:11-17.
51 Pang D. Principles and pitfalls of spinal stabilization in children. In: Pang D, editor. Disorders of the Paediatric Spine. New York: Raven Press; 1995:575-604.
52 Werthem SB, Bohlman HH. Occipitocervical fusion. Indications, techniques, and long-term results in thirteen patients. J Bone Joint Surg Am. 1987;69:833-836.
53 Beatson T. Fractures and dislocations of the cervical spine. J Bone Joint Surg Br. 1963;45:21-35.
54 Hubbard DD. Injuries of the spine in children and adolescents. Clin Orthop Relat Res. 1974;100:56-65.
55 Alexander EJ, Davis C, Field C. Hyperextension injuries of the cervical spine. Arch Neurol Psychiatry. 1958;79:146-150.
56 Taylor AR, Blackwood W. Paraplegia in hyperextension cervical injuries with normal radiographic appearances. J Bone Joint Surg Br. 1948;30:245-248.
57 Breig A, El-Nadi AF. Biomechanics of the cervical spinal cord: relief of contact pressure on and over-stretching of the spinal cord. Acta Radiol (Diag). 1966;4:602-624.
58 Bourmer HR. Zur Frage der Halsmarkschadigung bei Hyperestensionsverletzungen der Wirbelsaule. Arch Klin Chir. 1951;268:409-416.
59 Marar BC. Hyperextension injuries of the cervical spine: the pathogenesis of damage to the spinal cord. J Bone Joint Surg Am. 1974;56:1655-1662.
60 Sudeck P. Uber Drchungsverrenkung des Atlas. Dtsch Z Chir. 1923;183:289-303.
61 Glasauer F, Cares HL. Biomechanical features of traumatic paraplegia in infancy. J Trauma. 1973;13:166-170.
62 Leventhal HR. Birth injuries of the spinal cord. J Pediatr. 1960;56:447-453.
63 Abroms I, Brosnan MJ, Zuckerman JE, et al. Cervical cord injuries secondary to hyperextension of the head in breech presentations. Obstet Gynecol. 1973;41:369-378.
64 Pollack IF, Pang D, Hall WA. Subarachnoid pleural and subarachnoid-mediastinal fistulae. Neurosurgery. 1990;26:519-525.
65 Davis D, Bohlman H, Walker E, et al. The pathological finding in fatal craniospinal injuries. J Neurosurg. 1971;34:603-613.
66 Ahmann PA, Smith SA, Schwartz JF, et al. Spinal cord infarction due to minor trauma in children. Neurology. 1975;25:301-307.
67 Cheshire D. The pediatric syndrome of traumatic myelopathy without demonstrable vertebral injury. Paraplegia. 1977;15:74-85.
68 Walsh JW, Steven DB, Young AB. Traumatic paraplegia in children without contiguous spinal fracture of dislocation. Neurosurgery. 1983;12:439-445.
69 Pollack IF, Pang D, Sclabassi R. Recurrent spinal cord injury without radiographic abnormalities in children. J Neurosurg. 1988;69:177-182.
70 Grabb PA, Pang D. Magnetic resonance imaging in the evaluation of spinal cord injury without radiographic abnormality (SCIWORA) in children. Neurosurgery. 1994;35:406-414.
71 Flanders AE, Schaeffer DM, Doan HT, et al. Acute cervical spine trauma: correlation of MR imaging findings with degree of neurologic deficit. Radiology. 1990;177:25-33.
72 Weirich SD, Cotler HB, Narayana PA, et al. Histopathologic correlation of magnetic resonance imaging signal patterns in a spinal cord injury model. Spine. 1990;15:630-638.
73 Yamashita Y, Takahashi M, Matsuno Y, et al. Acute spinal cord injury: magnetic resonance imaging correlated with myelopathy. Br J Radiol. 1991;759:201-209.
74 Levitt MA, Flanders AE. Diagnostic capabilities of magnetic resonance imaging and computed tomography in acute cervical spinal column injury. Am J Emerg Med. 1991;9:131-135.
75 Pang D. Spinal cord injury without radiographic abnormalities in children, 2 decades later. Neurosurgery. 2004;55:1325-1343.
76 DePinto D, Payne T, Kittle CF. Traumatic subarachnoid-pleural fistula. Ann Thorac Surg. 1978;25:477-478.
77 LeBlanc HJ, Nadell J. Spinal cord injuries in children. Surg Neurol. 1974;2:441-444.
78 Rocha Campus BA, Silva LB, Ballalai N, et al. Traumatic subarachnoid-pleural fistula. J Neurol Neurosurg Psychiatry. 1974;37:269-270.
79 Scher AT. Trauma of the spinal cord in children. S Afr Med J. 1976;50:2023-2025.
80 Bracken MB, Shepard MJ, Holford TR, et al. Administration of methylprednisolone for 24 or 48 hours or tirilazad mesylate for 48 hours in the treatment of acute spinal cord injury. Results of the Third National Acute Spinal Cord Injury Randomized Controlled Trial. National Acute Spinal Cord Injury Study. JAMA. 1997;277:1597-1604.
81 Bracken MB, Shepard MJ, Holford TR, et al. Methylprednisolone or tirilazad mesylate administration after acute spinal cord injury: 1-year follow up. Results of the Third National Acute Spinal Cord Injury Randomized Controlled Trial. J Neurosurg. 1998;89:699-706.
82 Bracken M, Shepard MJ, Collins WF, et al. A randomized, controlled trial of methylprednisolone or naloxone in the treatment of acute spinal-cord injury: Results of the Second National Acute Spinal Cord Injury Study. N Engl J Med. 1990;322:1405-1411.
83 Pollack IF, Pang D. Spinal cord injury without radiographic abnormality (SCIWORA. In: Pang D, editor. Disorders of the Pediatric Spine. New York: Raven Press; 1995:509-516.
84 Kulkarni MV, McArdle CB, Kopanicky D, et al. Acute spinal cord injury: MR imaging at 1.5 T. Radiology. 1987;164:837-843.
85 Quencer RM. The injured spinal cord. Evaluation with magnetic resonance and intraoperative sonography. Radiol Clin North Am. 1988;26:1025-1045.
86 Fielding J. Selected observations on the cervical spine in the child. Curr Pract Orthop Surg. 1973;5:31-55.
87 Fielding JW, Cochran GVB, Lawsing JF, et al. Tears of the transverse ligament of the atlas. J Bone Joint Surg Am. 1974;56:1683-1691.
88 White AA, Panjabi MM. The clinical biomechanics of the occipitoatlanto-axial complex. Orthop Clin North Am. 1978;9:867-878.
89 Fielding JW, Hawkins RJ. Roentgenographic diagnosis of the injured neck. Am Assoc Orthop Surgeons Instr Course Lect. 1976;25:149-170.
90 Martel E, Tishler J. Observations on the spine in mongoloidism. AJR Am J Roentgenol. 1966;97:630-638.
91 Pueschel S, Scola FH. Atlanto-axial instability in individuals with Down’s syndrome: epidemiologic, radiographic and clinical studies. Pediatrics. 1987;80:555-560.
92 Pueschel S, Scola FH, Perry CD, et al. Atlanto-axial instability in children with Down’s syndrome. Pediatr Radiol. 1981;10:129-132.
93 White KS, Ball WS, Prenger EC, et al. Evaluation of craniocervical junction in Down syndrome: correlation of measurements obtained with radiography and MR imaging. Radiology. 1993;186:377-382.
94 Kopits SE, Steingass MH. Experience with the “halo-cast” in small children. Surg Clin North Am. 1970;50:935-943.
95 Rogers W. Treatment of fracture dislocation of the cervical spine. J Bone Joint Surg. 1942;24:245-258.
96 White AA, Panjabi MM. The basic kinematics of the human spine. Spine. 1978;3:12-20.
97 White AA, Panjabi MM. Clinical Biomechanics of the Spine, 2nd ed. Philadelphia: JB Lippincott; 1990.
98 White AA, Panjabi MM. The problem of clinical stability in the human spine: a systemic approach. In: White AA, Panjabi MM, editors. Clinical Biomechanics of the Spine. Philadelphia: JB Lippincott; 1978:196.
99 Adams VI. Neck injuries. I. Occipitoatlantal dislocation—a pathological study of twelve traffic fatalities. J Forensic Sci. 1992;37:556-564.
100 Alker G, Oh Y, Leslie E. High cervical spine and craniofacial junction injuries in fatal traffic accidents: a radiographic study. Orthop Clin North Am. 1978;9:1003-1010.
101 Bucholz RW, Burkhead WZ. The pathological anatomy of fatal atlanto-occipital dislocations. J Bone Joint Surg Am. 1979;61:248-250.
102 Bohlman H. Acute fractures and dislocations of the cervical spine: an analysis of three hundred hospitalized patients and review of the literature. J Bone Joint Surg Am. 1979;61:1119-1142.
103 Gabrielsen TO, Maxwell JA. Traumatic atlantooccipital dislocation: with case report of patient who survived. AJNR Am J Neuroradiol. 1966;97:624-629.
104 Harmanli O, Koyfman Y. Traumatic atlanto-occipital dislocation with survival: a case report and review of the literature. Surg Neurol. 1993;39:324-330.
105 Dvorak J, Panjabi MM. Functional anatomy of the alar ligaments. Spine. 1987;12:183-189.
106 Werne S. Studies in spontaneous atlas dislocation. Acta Orthop Scand. 1957;23:1-150.
107 Goel VK, Clark CR, Callaes K, et al. Moment-rotation relationships of the ligamentous occipito-atlanto-axial complex. J Biomech. 1988;21:673-680.
108 Menezes AH, Muhonen M. Management of occipital-cervical instability. In: Cooper P, editor. Management of Post Traumatic Instability. Park Ridge, IL: American Association of Neurological Surgeons; 1990:65-76.
109 Dublin AB, Marks WM, Wienstock D, et al. Traumatic dislocation of the atlanto-occipital articulation (AOA) with short-term survival. J Neurosurg. 1980;52:541-546.
110 Grobovschek M, Scheibelbrandner W. Atlanto-occipital dislocation. Neuroradiology. 1983;25:173-174.
111 Powers B, Miller MD, Kramer RS, et al. Traumatic anterior atlanto-occipital dislocation. Neurosurgery. 1979;4:12-17.
112 Brooks A, Jenkins EB. Atlanto-axial arthrodesis by the wedge compression method. J Bone Joint Surg Am. 1978;60:279-284.
113 Englander O. Non-traumatic occipito-atlanto-axial dislocation: a contribution to the radiology of the atlas. Br J Radiol. 1942;15:341-345.
114 Eismont FJ, Bohlman HH. Posterior atlanto-occipital dislocation with fractures of the atlas and odontoid process: report of a case with survival. J Bone Joint Surg Am. 1978;60:397-399.
115 Britt R, Herrick MK, Mason RT, et al. Traumatic lesions of the pontomedullary junction. Neurosurgery. 1980;6:623-631.
116 Leetsma JE, Kalelkar MB, Teas S. Ponto-medullary avulsion associated with cervical hyperextension. Acta Neurochir (Wien). 1983;32:69-73.
117 Lindenberg R, Freytag E. Brainstem lesions characteristic of traumatic hyperextension of the head. Arch Pathol. 1970;90:509-515.
118 Traynelis VC, Marano GD, Dunker RO, et al. Traumatic atlanto-occipital dislocation: case report. J Neurosurg. 1986;65:863-870.
119 Georgopoulos G, Pizzutillo PD, Lee MS. Occipito-atlantal instability in children. J Bone Joint Surg Am. 1987;69:429-436.
120 Pang D, Wilberger JE. Traumatic atlantooccipital dislocation with survival: case report and review. Neurosurgery. 1980;7:503-508.
121 Woodring JH, Selke AC, Duff DE. Traumatic atlantooccipital dislocation with survival. AJR Am J Roentgenol. 1981;137:21-24.
122 Sun PP, Poffenbarger J, Durhan S, et al. Spectrum of occipitoatlantoaxial injury in young children. J Neurosurg Spine. 2000;1:28-39.
123 Pang D, Nemzek WR, Zovickian J. Atlanto-occipital dislocation Part 2: the clinical use of (occipital) condyle-C1 interval, comparison with other diagnostic methods, and the manifestation, management, and outcome of atlanto-occipital dislocation in children. Neurosurgery. 2007;61:995-1015.
124 Watridge CB, Orrison WW, Arnold H, et al. Lateral atlantooccipital dislocation: case report. Neurosurgery. 1985;17:345-347.
125 Altongy J, Fielding JW. Combined atlanto-axial and occipitoatlantal rotatory subluxation. J Bone Joint Surg Am. 1990;72:923-926.
126 Clark CR, Kathol MH, Walsh T, et al. Atlantoaxial rotatory fixation with compensatory counter occipitoatlantal subluxation. Spine. 1986;11:1048-1050.
127 Bools J, Rose B. Traumatic atlantooccipital dislocation: two cases with survival. AJNR Am J Neuroradaiol. 1986;7:901-904.
128 Collato PM, Demuth WW, Schwentker EP, et al. Traumatic atlanto-occipital dislocation: case report. J Bone Joint Surg Am. 1986;68:1106-1109.
129 Dibenedetto T, Lee CK. Traumatic atlanto-occipital instability: a case report with follow-up and a new diagnostic technique. Spine. 1990;15:595-597.
130 Farley FA, Graziano GP, Hensinger RN. Traumatic atlantooccipital dislocation in a child. Spine. 1992;17:1539-1541.
131 Kaufman RA, Dunbar JS, Botsfor JA, et al. Traumatic longitudinal atlanto-occipital distraction injuries in children. AJNR Am J Neuroradiol. 1982;3:415-419.
132 Montane I, Eismont FJ, Green BA. Traumatic occipitoatlantal dislocation. Spine. 1991;16:112-116.
133 Putman WE, Straaton FT, Rohr RJ, et al. Traumatic atlantooccipital dislocations: value of the Powers ratio in diagnosis. J Am Osteopath Assoc. 1986;86:798-804.
134 Lee C, Woodring JH, Goldstein SJ, et al. Evaluation of traumatic atlantooccipital dislocations. AJNR Am J Neuroradiol. 1987;8:19-26.
135 Page CP, Story JL, Wissinger JP, et al. Traumatic atlantooccipital dislocation: case report. J Neurosurg. 1973;39:394-397.
136 Fruin AH, Pirotte TP. Traumatic atlantooccipital dislocation: case report. J Neurosurg. 1977;46:663-666.
137 Ajuja A, Glasauer F, Alker G, et al. Radiology in survivors of traumatic atlantooccipital dislocation. J Neurol. 1994;41:112-118.
138 Bulas DI, Fitz CR, Johnson DL. Traumatic atlanto-occipital dislocation in children. Radiology. 1993;188:155-158.
139 Bundschuh CV, Alley JB, Ross M, et al. Magnetic resonance imaging of suspected atlantooccipital dislocation: two case reports. Spine. 1992;17:245-248.
140 Cohen A, Hirsch M, Katz M, et al. Traumatic atlanto-occipital dislocation in children: review and report of five cases. Pediatr Emerg Care. 1991;7:24-27.
141 Evarts C. Traumatic occipito-atlantal dislocation: report of a case with survival. J Bone Joint Surg Am. 1970;52:1653-1660.
142 Farthing J. Atlantocranial dislocation with survival: a case report. N C Med J. 1948;9:34-36.
143 Hosono N, Yonenobu K, Kawagoe K, et al. Traumatic anterior atlanto-occipital dislocation: a case with survival. Spine. 1993;18:786-790.
144 Lee C, Woodring JH, Walsh JW. Carotid and vertebral artery injury in survivors of atlanto-occipital dislocation: case reports and literature review. J Trauma. 1991;31:401-407.
145 Matava MJ, Whitesides TE, Davis PC. Traumatic atlanto-occipital dislocation with survival: serial computerized tomography as an aid to diagnosis and reduction: a report of three cases. Spine. 1993;18:1897-1903.
146 Wholey MH, Bruwer AJ, Baker HLJr. The lateral roentgenogram of the neck: with comments on the atlanto-odontoid relationship. Radiology. 1958;71:350-356.
147 Harris JJr, Carson GC, Wagner LK. Radiologic diagnosis of traumatic occipitovertebral dislocation. 1. Normal occipitovertebral relationships on the lateral radiographs of supine subjects. AJNR Am J Neuroradiol. 1993;162:881-886.
148 Harris JHJr, Carson GC, Wagner LK, et al. Radiologic diagnosis of traumatic occipitovertebral dislocation. 2. Comparison of three methods of detecting occipitovertebral relationships on lateral radiographs of supine subjects. AJNR Am J Neuroradiol. 1993;162:887-892.
149 Pang D, Nemzek WR, Zovickian J. Atlanto-occipital dislocation. Part 1: normal occipital condyle-C1 interval in 89 children. Neurosurgery. 2007;61:514-521.
150 Brinkman W, Cohen W, Manning T. Posterior fossa subarachnoid hemorrhage due to an atlantooccipital dislocation. AJR Am J Roentgenol. 2003;180:1476.
151 Gross A. Traumatic basal subarachnoid hemorrhages: autopsy material analysis. Forensic Sci Int. 1990;45:53-61.
152 Imaizumi T, Sohma T, Hotta H, et al. Associated injuries and mechanism of atlanto-occipital dislocation caused by trauma. Neurol Med Chir (Toyko). 1995;35:385-391.
153 Przybylski GJ, Clyde BL, Fitz CR. Craniocervical junction subarachnoid hemorrhage associated with atlanto-occipital dislocation. Spine. 1996;21:1761-1768.
154 Dickman CA, Papadopoulos SM, Sonntag VKH, et al. Traumatic occipitoatlantal dislocation. J Spinal Disord. 1993;6:300-313.
155 Donahue DJ, Muhlbauer MS, Kaufman RA, et al. Childhood survival of atlantooccipital dislocation: underdiagnosis, recognition, treatment, and review of the literature. Pediatr Neurosurg. 1994;21:105-111.
156 Fisher CG, Sun JCL, Dovrak M. Recognition and management of atlanto-occipital dislocation: improving survival from an often fatal condition. Can J Surg. 2001;44:412-420.
157 Kenter K, Worley G, Griffin T, et al. Pediatric traumatic atlanto-occipital dislocation: five cases and a review. J Pediatr Orthrop. 2001;21:585-589.
158 Diagnosis and management of traumatic atlanto-occipital dislocation injuries. Neurosurgery. 2002;50(3 Suppl):S105-S113.
159 Papadopoulos SM, Dickman CA, Sonntag VK, et al. Traumatic atlantooccipital dislocation with survival. Neurosurgery. 1991;28:574-579.
160 Grob D. Transarticular screw fixation for atlanto-occipital dislocation. Spine. 2001;26:703-707.
161 Herzenberg JE, Hensiger RN, Dedrick DK, et al. Emergency transport and positioning of young children who have an injury of the cervical spine: the standard backboard may be dangerous. J Bone Joint Surg Am. 1989;71:15-22.
162 Naso WB, Cure J, Cuddy BG. Retropharyngeal pseudomeningocele after atlanto-occipital dislocation: report of two cases. Neurosurgery. 1997;40:1288-1291.
163 Steinmetz MP, Verrees M, Anderson JS, et al. Dual-strap augmentation of a halo orthosis in the treatment of atlantooccipital dislocation in infants and young children. J Neurosurg Spine. 2002;96:346-349.
164 Frank C, Amiel D, Woo SL, et al. Normal ligament properties and ligament healing. Clin Orthop Relat Res. 1985;196:15-25.
165 Sponseller PD, Cass JR. Atlanto-occipital fusion for dislocation in children with neurologic preservation. A case report. Spine. 1997;22:344-347.
166 Yamaguchi N, Ikeda K, Ishise J, et al. Traumatic atlanto-occipital dislocation with long-term survival: case report. Neurol Med Chir (Tokyo). 1996;36:36-39.
167 Harty JA, Sparkes J, McCormack D, et al. Recognition of progressive atlanto-occipital dislocation (by a changing neurologic status and clinical deformity). J Orthrop Trauma. 2003;17:299-302.
168 Takayasu M, Hara M, Suzuki Y, et al. Treatment of traumatic atlanto-occipital dislocation in chronic phase. Neurosurg Rev. 1999;22:135-137.
169 Hulbert RJ, Crawford NR, Choi WG, et al. A biomechanical evaluation of occipitocervical instrumentation: screw compared with wire fixation. J Neurosurg Spine. 1999;90:84-90.
170 Montesano PX, Juach EC, Anderson PA, et al. Biomechanics of cervical spine internal fixation. Spine. 1994;16:10-16.
171 Pang D, Hanley EN. Special problems of spinal stabilization in children. In: Cooper PR, editor. Management of Post-Traumatic Spinal Instability. Neurological Topics. Park Ridge, IL: American Association of Neurological Surgeons; 1990:181-206.
172 Magerl F, Seemann PS. Stable posterior fusion of the atlas and axis by transarticular screw fixation. In: Kehr P, Weidner A, editors. Cervical Spine. Berlin: Springer-Verlag; 1986:322-327.
173 Sasso RC, Jeanneret B, Fischer K, et al. Occipitocervical fusion with posterior plate and screw instrumentation: a long term follow-up study. Spine. 1994;19:2364-2368.
174 Smith MD, Anderson P, Grady MS. Occipitocervical arthrodesis using contoured plate fixation: an early report on a versatile fixation technique. Spine. 1993;18:1984-1990.
175 Harms J, Melcher RP. Posterior C1-2 fusion with polyaxial screw and rod fixation. Spine. 2001;26:2467-2471.
176 Belzberg A, Tranmer B. Stabilization of traumatic atlanto-occipital dislocation: case report. J Neurosurg. 1991;75:478-482.
177 Lowry DW, Pollack IF, Clyde B, et al. Upper cervical spine fusion in the pediatric population. J Neurosurg. 1997;87:671-676.
178 Pang D, Sun PP. Pediatric vertebral column and spinal cord injuries, 5th ed. Youmans JR, editor. Neurological Surgery, vol 3. Philadelphia: WB Saunders. 2004:3315-3357.
179 Pait TG, Al-Mefty O, Boop FA, et al. Inside-outside technique for posterior occipitocervical spine instrumentation and stabilization: Preliminary results. J Neurosurg Spine. 1999;90:1-7.
180 Saeheng S, Phuenpathom N. Traumatic occipitoatlantal dislocation. Surg Neurol. 2001;55:35-40.
181 Goldberg AL, Baron B, Daffner RH. Atlantooccipital dislocation: MR demonstration of cord damage. J Comput Assist Tomogr. 1991;15:174-175.
182 Levine AM, Edwards CC. Traumatic lesions of the occipitoatlantoaxial complex. Clin Orthop Relat Res. 1989;239:53-68.
183 Pang D, Li V. Atlanto-axial rotatory fixation Part I: biomechanics of normal rotation at the alanto-axial joint in children. Neurosurgery. 2004;55:614-626.
184 Coutts M. Atlanto-epistropheal subluxations. Arch Surg. 1934;29:297-311.
185 DeRoeck BN. A case of rotatory dislocation of atlas on axis. Radiography. 1977;43:127-130.
186 Fielding JW, Hawkins RJ. Atlantoaxial rotatory fixation. J Bone Joint Surg Am. 1977;59:37-44.
187 Hess JG, Bronstein IP, Abelson SM. Atlanto-axial dislocations. Am J Dis Child. 1935;49:1137-1147.
188 Keuter EJW. Non-traumatic atlanto-axial dislocation associated with nasopharyngeal infections. Acta Neurochir (Wien). 1969;21:11-22.
189 Mazur JM, Hopla DM, Bass RM. Cervical vertebrae subluxation. Laryngoscope. 1983;93:1155-1159.
190 Phillips W, Hensinge RN. The management of rotatory atlantoaxial subluxation in children. J Bone Joint Surg Am. 1989;71:664-668.
191 Pinkham JR. Inflammatory subluxation of the atlanto-axial joint. South Med J. 1976;69:1507-1509.
192 Sullivan AW. Subluxation of the atlanto-axial joint: sequel to inflammatory processes of the neck. J Pediatr. 1949;35:451-464.
193 Wilson BC, Jarvis BL, Hayden RCIII. Nontraumatic subluxation of the atlanto-axial joint: Grisel’s syndrome. Ann Otol Rhinol Laryngol. 1987;96:705-708.
194 Wittek A. Ein Fall von Distensionluxation im Atlanto-epiphyseal Gelenke. Munch Med Wochenschr. 1908;55:1836-1837.
195 Birney T, Hanley E. Traumatic cervical spine injuries in childhood and adolescence. Spine. 1989;14:1277-1282.
196 Corner E. Rotatory dislocations of the atlas. Ann Surg. 1907;45:9-26.
197 Fielding JW. Cineroentgenography of the normal cervical spine. J Bone Joint Surg Am. 1957;39:1280-1288.
198 Eadie PA, Moran R, Fogarty EE, et al. Rotatory atlanto-axial subluxation following pharyngoplasty. Br J Plast Surg. 1989;42:722-723.
199 Sipila P, Palva A, Sorri M, et al. Atlanto-axial subluxation. Arch Otolaryngol. 1981;107:181-182.
200 Sherk HH, Nicholson JT. Rotatory atlanto-axial dislocation with ossiculum terminale and mongolism. J Bone Joint Surg Am. 1969;51:957-964.
201 Wortzman G, Dewar FP. Rotary fixation of the atlanto-axial joint. Rotational atlanto-axial subluxation. Radiology. 1968;90:479-487.
202 Fielding JW, Stillwell WT, Chynn KY, et al. Use of computed tomography for the diagnosis of atlanto-axial rotatory fixation. J Bone Joint Surg Am. 1978;60:1102-1104.
203 Kawabe N, Hirotani H, Tanaka O. Pathomechanism of atlantoaxial rotatory fixation in children. J Pediatr Orthop. 1989;9:569-574.
204 Kowalski HM, Cohen WA, Cooper P, et al. Pitfalls in the CT diagnosis of atlanto-axial rotatory subluxation. AJR Am J Roentgenol. 1987;149:595-600.
205 Ono K, Yonenobu K, Fuji T, et al. Atlanto-axial rotatory fixation: radiographic study of its mechanism. Spine. 1985;10:602-608.
206 Pang D, Li V. Atlanto-axial rotatory fixation Part II: new diagnostic paradigm and a new classification based on motion analysis using computed tomographic imaging. Neurosurgery. 2005;57:941-953.
207 Li V, Pang D. Atlanto-axial rotatory fixation. In: Pang D, editor. Disorders of the Pediatric Spine. New York: Raven Press; 1995:31-553.
208 Dvorak J, Panjabi M, Gerber M, et al. CT-functional diagnostics of the rotatory instability of upper cervical spine: Part I: an experimental study on cadavers. Spine. 1987;12:197-205.
209 Govender S, Kumar KPS. Staged reduction and stabilisation in chronic atlantoaxial rotatory fixation. J Bone Joint Surg Br. 2002;84:727-731.
210 Crossman JE, David K, Hayward R, et al. Open reduction of pediatric atlantoaxial rotatory fixation: long-term outcome study with functional measurements. J Neurosurg Spine. 2004;100:235-240.
211 Crossman JE, Thompson D, Hayward RD, et al. Recurrent atlantoaxial rotatory fixation in children: a rare complication of a rare condition. J Neurosurg Spine. 2004;100:307-311.
212 Roche CJ, O’Malley M, Dorgan JC, et al. A pictorial review of atlanto-axial rotatory fixation: key points for the radiologist. Clin Radiol. 2001;56:947-958.
213 Al-Etani H, D’Astous J, Letts M, et al. Masked rotatory subluxation of the atlas associated with fracture of the clavicle: a clinical and biomechanical analysis. Am J Orthop. 1988;5:375-380.
214 Pang D, Li V. Atlanto-axial rotatory fixation Part III: a prospective study of the clinical manifestation, diagnosis, management and outcome of children with atlanto-axial rotatory fixation. Neurosurgery. 2005;57:954-972.
215 Villas C, Arriagada C, Zubieta JL. Preliminary CT study of C1-C2 rotational mobility in normal subjects. Eur Spine J. 1999;8:223-228.
216 Crockard HA, Rogers MA. Open reduction of traumatic atlanto-axial rotatory dislocation with use of the extreme lateral approach. J Bone Joint Surg Am. 1996;78:431-436.
217 Hosono N, Yonenobu K, Tada K, et al. Lateral atlantoaxial joint arthrography in atlantoaxial rotatory fixation. J Spinal Disord Tech. 2003;16:216-220.
218 Goto S, Mochizuki M, Kita T, et al. Transoral joint release of the dislocated atlantoaxial joints combined with posterior reduction and fusion for a late infantile atlantoaxial rotatory fixation: a case report. Spine. 1998;23:1485-1489.
219 Adams VI. Neck injuries. II. Atlantoaxial dislocation—a pathologic study of 14 traffic fatalities. J Forensic Sci. 1992;37:565-573.
220 Bohn D, Armstrong D, Becker L, et al. Cervical spine injuries in children. J Trauma. 1990;30:463-469.
221 McGrory BJ, Klassen RA, Chao EYS, et al. Acute fractures and dislocations of the cervical spine in children and adolescents. J Bone Joint Surg Am. 1993;75:988-995.
222 DeBeer JDV, Thomas M, Walters J, et al. Traumatic atlanto-axial subluxation. J Bone Joint Surg Br. 1988;70:652-655.
223 Floman Y, Kaplan L, Elidan J, et al. Transverse ligament rupture and atlantoaxial subluxation in children. J Bone Joint Surg Br. 1991;73:640-643.
224 Hamilton AR. Injuries of the atlantoaxial joint. J Bone Joint Surg Br. 1951;33:434-435.
225 Spitzer R, Rabinowitch JY, Wybar KC. A study of the abnormalities of the skull, teeth and lenses in mongolism. Can Med Assoc J. 1961;84:567-572.
226 Wigren A, Amici F. Traumatic atlanto-axial dislocation without neurologic disorder. J Bone Joint Surg Am. 1973;55:642-644.
227 Maiman DJ, Cusick JF. Traumatic atlantoaxial dislocation. J Neurol. 1982;18:388-392.
228 Wolf JW, Johnson RM. Cervical orthoses. In: Bailey DW, Sherk HH, editors. The Cervical Spine. Philadelphia: JB Lippincott; 1983:54-61.
229 Braakman R, Penning L. Upper cervical spine. In: Injuries of the Cervical Spine. Amsterdam: Excerpta Medica; 1971:125-157.
230 Locke GR, Gardner JI, Van Epps EF. Atlas-dens interval (ADI) in children. Am J Radiol. 1966;97:135-140.
231 Steel HH. Anatomical and mechanical considerations of the atlanto-axial articulations. J Bone Joint Surg Am. 1968;50:1481-1482.
232 Levine AM, Edwards CC. Treatment of injuries in the C1-C2 complex. Orthop Clin North Am. 1986;17:31-44.
233 Hreidarsson S, Magram G, Singer H. Symptomatic atlantoaxial dislocation in Down’s syndrome. Pediatrics. 1982;69:568-571.
234 Hungerford GD, Akkaraju V, Rawe SE, et al. Atlantooccipital and atlanto-axial dislocations with spinal cord compression in Down’s syndrome: a case report and review of the literature. Br J Radiol. 1981;54:758-761.
235 Stein SM, Kircner SG, Horev G, et al. Atlantooccipital subluxation in Down’s syndrome. Pediatr Radiol. 1991;21:121-124.
236 Burke SW, French HG, Roberts JM, et al. Chronic atlantoaxial instability in Down’s syndrome. J Bone Joint Surg Am. 1985;67:1356-1360.
237 Tishler J, Martel W. Dislocation of the atlas in mongolism. Radiology. 1965;84:904-906.
238 Aung M. Atlanto-axial dislocation in Down’s syndrome. Bull Los Angeles Neurol Soc. 1973;38:197-201.
239 Levack B, Roper AB. Dislocation in Down’s syndrome. Dev Med Child Neurol. 1984;26:122-125.
240 Semine AA, Ertel AN, Goldberg MJ, et al. Cervical-spine instability in children with Down’s syndrome (trisomy 21). J Bone Joint Surg Am. 1978;60:649-652.
241 Beighton P, Craig J. Atlanto-axial subluxation in the Morquio syndrome. J Bone Joint Surg Br. 1973;55:478-481.
242 Dawson EG, Smith L. Atlanto-axial subluxation in children due to vertebral anomalies. J Bone Joint Surg Am. 1979;61:582-587.
243 Herring JA. Cervical instability in Down’s syndrome and juvenile rheumatoid arthritis. J Pediatr Orthop. 1982;2:205-207.
244 Kopits SE, Perovic MN, McKusick V, et al. Congenital atlantoaxial dislocations in various forms of dwarfism. J Bone Joint Surg Am. 1972;54:1349-1350.
245 Stevens JC, Cartlidge NEF, Saunders M, et al. Atlanto-axial subluxation and cervical myelopathy in rheumatoid arthritis. Q J Med. 1971;159:391-408.
246 Callahan RA, Johnson RM, Margolis RN, et al. Cervical facet fusion for control of instability following laminectomy. J Bone Joint Surg Am. 1977;59:991-1002.
247 Johnson R, Owen JR, Hart BA, et al. Cervical orthoses. Clin Orthop Relat Res. 1981;154:34-45.
248 Johnson R, Owen JR, Panjabi MM, et al. Immediate strength of certain cervical fusion techniques [abstract]. Orthop Trans. 1980;4:42.
249 Prolo DJ, Runnels JB, Jameson RM. The injured cervical spine: immediate and long-term immobilization with the halo. JAMA. 1973;224:591-594.
250 Baum J, Hanley E, Pullekines J. Comparison of halo complications in adults and children. Spine. 1989;14:251-252.
251 Chan RC, Schweigel JF, Thompson GB. Halo-thoracic brace immobilization in 188 patients with acute cervical spine injuries. J Neurosurg. 1983;58:508-515.
252 Mubarak SJ, Camp JF, Vuletich W, et al. Halo immobilization for cervical spine instability in the infant [abstract]. Paper presented at the Annual Meeting of the Pediatric Orthopedic Society of North America, 1988, Colorado Springs, CO.
253 Garfin SR, Bott MJ, Waters RL, et al. Complications in the use of the halo fixation device. J Bone Joint Surg Am. 1986;68:320-325.
254 Victor DI, Bresnan MJ, Keller RB. Brain abscess complicating the use of halo traction. J Bone Joint Surg Am. 1973;55:635-639.
255 Goodman ML, Nelson PB. Brain abscess complicating the use of a halo orthosis. Neurosurgery. 1987;20:27-30.
256 Hoffman GR, Merckx J, Vercauteren M. Abces cerebral consecutif a la “halo traction.”. Neurochirurgie. 1974;20:263-266.
257 Humbyrd DE, Latimer FR, Lonstein JE, et al. Brain abscess as a complication of halo traction. Spine. 1981;6:365-368.
258 Benzel E, Hadden A, Saulsbery C. A comparison of the Minerva and halo jackets for stabilization of the cervical spine. J Neurosurg. 1989;70:411-414.
259 Anderson L. Fractures of the Odontoid Process of the Axis. Philadelphia: JB Lippincott; 1983.
260 Walter BC. Cranial bone grafts for use in posterior fixation of the cervical spine. J Neurosurg. 1993;79:286-288.
261 Johnson RM, Wolf JW. Stability. In: Bailey R, Sherk HH, editors. The Cervical Spine. Philadelphia: JB Lippincott; 1983:35-53.
262 Johnson R, Southwick WO. Surgical approaches to the spine. In: Rothman R, Simeone FA, editors. The Spine. 2nd ed. Philadelphia: WB Saunders; 1981:67-147.
263 Eismont F, Bohlman HH. Posterior methylmethacrylate fixation for cervical trauma. Spine. 1981;6:347-353.
264 Whitehill R, Stowers SF, Fechner RE, et al. Posterior cervical fusions using cerclage wires, methylmethacrylate cement and autogenous bone graft: an experimental study of a canine model. Spine. 1987;12:12-22.
265 Cooper PR, Cohen A, Rosiello A, et al. Posterior stabilization of cervical spine fractures and subluxations using plates and screws. Neurosurgery. 1988;23:300-306.
266 Gallie WE. Fractures and dislocations of the cervical spine. Am J Surg. 1939;10:495-499.
267 Griswold DM, Albright JA, Schiffman E, et al. Atlanto-axial fusion for instability. J Bone Joint Surg Am. 1978;60:285-292.
268 Levy ML, McComb JG. C1-C2 fusion in children with atlantoaxial instability and spinal cord compression: technical note. Neurosurgery. 38, 1996. 221-217
269 Apfelbaum RI. Posterior C1-2 screw fixation for atlantoaxial instability. Neurosurg Oper Atlas. 1995;4:19-28.
270 Grob D, Crisco JJIII, Panjabi MM, et al. Biomechanical evaluation of four different posterior atlantoaxial fixation techniques. Spine. 1992;17:480-490.
271 Dickman CA, Sonntag VKH. Posterior C1-C2 transarticular screw fixation for atlantoaxial arthrodesis. Neurosurgery. 1998;43:275-281.
272 Grob D, Jeanneret B, Aebi M, et al. Atlanto-axial fusion with transarticular screw fixation. J Bone Joint Surg Br. 1991;73:972-976.
273 Dickman CA, Sonntag VKH, Papadopoulos SM, et al. The interspinous method of posterior atlantoaxial arthrodesis. J Neurosurg. 1991;74:190-198.
274 Wang J, Vokshoor A, Kim S, et al. Pediatric atlantoaxial instability: management with screw fixation. Pediatr Neurosurg. 1999;30:70-78.
275 Gluf WM, Brockmeyer DL. Atlantoaxial transarticular screw fixation: a review of surgical indications, fusion rate, complications and lessons learned in 67 pediatric patients. J Neurosurg Spine. 2005;2:164-169.
276 Madawi AA, Casey ATH, Solanki GA, et al. Radiological and anatomical evaluation of the atlantoaxial transarticular screw fixation technique. J Neurosurg. 1997;86:961-968.
277 Paramore CG, Dickman CA, Sonntag VKH. The anatomical suitability of the C1-2 complex for transarticular screw fixation. J Neurosurg. 1996;85:221-224.
278 Dickman CA, Crawford NR, Paramore CG. Biomechanical characteristics of C1-C2 cable fixations. J Neurosurg. 1996;85:316-322.
279 Heywood AW, Learmonth ID, Thomas M. Internal fixation for occipito-cervical fusion. J Bone Joint Surg Br. 1988;70:708-711.
280 Brattstrom H. Surgery in atlanto-axial dislocation in rheumatoid arthritis. Reconstr Surg Traumatol. 1981;18:16-29.
281 Cregan JC. Internal fixation of the unstable rheumatoid cervical spine. Ann Rheum Dis. 1966;25:242-252.
282 Dickman CA, Sonntag VK, Marcotte PJ. Techniques of screw fixation of the cervical spine. BNI Q. 1992;8:9-26.
283 Fehlings MG, Errico T, Cooper P, et al. Occipitocervical fusion with a five-millimeter malleable rod and segmental fixation. Neurosurgery. 1993;32:198-207.
284 Kerschbaumer F. Rheumatoid instability. In: Bauer R, Kerschbaumer F, Poisel S, editors. Atlas of Spinal Operations. New York: Thieme; 1993:347-376.
285 Roy-Camille R, Saillant G, Mazel C. Internal fixation of the unstable cervical spine by a posterior osteosynthesis with plates and screws. In: Society CSR, editor. The Cervical Spine. 2nd ed. Philadelphia: JB Lippincott; 1989:390-403.
286 Sonntag VK, Dickman CA. Craniocervical stabilization. Clin Neurosurg. 1993;40:243-272.
287 Sonntag VK, Dickman CA. Occipitocervical instrumentation. In: Hitchon PW, Traynells VC, Rengachary SS, editors. Techniques in Spinal Fusion and Stabilization. New York: Thieme; 1995:107-121.
288 Sumi M, Kataoka O, Ikeda M, et al. Atlantoaxial dislocation: a follow-up study of surgical results. Spine. 1997;22:759-764.
289 Brockmeyer DL, Apfelbaum RI. A new occipitocervical fusion construct in pediatric patients with occipitocervical instability. Neurosurgery (Spine). 1999;90:271-275.
290 Jofe MH, White AA, Panjabi MM. Kinematics. In: Bailey RW, Sherk HH, editors. The Cervical Spine. Philadelphia: JB Lippincott; 1983:23-35.