Traditional Cultivation and Identification
1. Define bacterial cultivation and list the three most important purposes for bacterial cultivation.
2. Define bacterial media; list the four general types of media and explain the general biochemical principle for each type.
3. List the environmental conditions that are crucial in supporting bacterial in vitro growth and explain how each factor is controlled and monitored.
4. Explain the most common bacterial streaking technique, the principle associated with the technique, and how colonies are enumerated using this technique.
5. Identify the key criteria used in characterizing and reporting bacterial culture growth pertaining to the phenotypic results; differentiate genotypic and phenotypic characteristics.
6. Explain the use and chemical principle of the following enzymatic tests used in preliminary bacterial identification: catalase test, oxidase test, urease test, indole test, PYR test, and hippurate hydrolysis.
7. Define and differentiate bacterial susceptibility and resistance; give an example in how these are used to assist in the identification bacteria.
8. List the three assays used to measure metabolic pathways and provide an example of each.
9. Describe the steps required to develop “rapid” identification schemes and explain how these differ from conventional schemes.
10. List the four basic identification components common to all commercially available multitest systems.
Principles of Bacterial Cultivation
• To grow and isolate all bacteria present in a clinical specimen
• To determine which of the bacteria that grow are most likely causing infection and which are likely contaminants or colonizers
• To obtain sufficient growth of clinically relevant bacteria to allow identification, characterization, and susceptibility testing
Nutritional Requirements
As discussed in Chapter 2, bacteria have numerous nutritional needs that include different gases, water, various ions, nitrogen, sources for carbon, and energy. The source for carbon and energy is commonly supplied in carbohydrates (e.g., sugars and their derivatives) and proteins.
Phases of Growth Media
In broth media, nutrients are dissolved in water, and bacterial growth is indicated by a change in the broth’s appearance from clear to turbid (i.e., cloudy). The turbidity, or cloudiness, of the broth is due to light deflected by bacteria present in the culture (Figure 7-1). More growth indicates a higher cell density and greater turbidity. At least 106 bacteria per milliliter of broth are needed for turbidity to be detected with the unaided eye.
With appropriate incubation conditions, each bacterial cell inoculated onto the agar medium surface will proliferate to sufficiently large numbers to be observable with the unaided eye (see Figure 7-1). The resulting bacterial population is considered to be derived from a single bacterial cell and is known as a pure colony. In other words, all bacterial cells within a single colony are the same genus and species, having identical genetic and phenotypic characteristics (i.e., are derived from a single clone). Pure cultures are required for subsequent procedures used to identify and characterize bacteria. The ability to select pure (individual) colonies is one of the first and most important steps required for bacterial identification and characterization.
Media Classifications and Functions
Enrichment media contain specific nutrients required for the growth of particular bacterial pathogens that may be present alone or with other bacterial species in a patient specimen. This media type is used to enhance the growth of a particular bacterial pathogen from a mixture of organisms by providing specific nutrients for the organism’s growth. One example of such a medium is buffered charcoal-yeast extract agar, which provides l-cysteine and other nutrients required for the growth of Legionella pneumophila, the causative agent of legionnaires’ disease (Figure 7-2).
Nutritive media or supportive media contain nutrients that support growth of most nonfastidious organisms without giving any particular organism a growth advantage. Nutrient media include tryptic soy agar, or nutrient agar plates for bacteria or Sabouraud’s dextrose agar for fungi. Selective media contain one or more agents that are inhibitory to all organisms except those “selected” by the specific growth condition or chemical. In other words, these media select for the growth of certain bacteria to the disadvantage of others. Inhibitory agents used for this purpose include dyes, bile salts, alcohols, acids, and antibiotics. An example of a selective medium is phenylethyl alcohol (PEA) agar, which inhibits the growth of aerobic and facultatively anaerobic gram-negative rods and allows gram-positive cocci to grow (Figure 7-3). Selective and inhibitory chemicals included within nutritive media prevent the overgrowth of normal flora or contaminating organisms that would prevent the identification of pathogenic organisms. However, it is important to note that the use of selective media does not ensure that the inhibited organisms are not present in small quantity and may simply be too small to see.
Differential media employ some factor (or factors) that allows colonies of one bacterial species or type to exhibit certain metabolic or culture characteristics that can be used to distinguish it from other bacteria growing on the same agar plate. One commonly used differential medium is MacConkey agar, which differentiates between gram-negative bacteria that can and cannot ferment the sugar lactose (Figure 7-4).
Of importance, many media used in diagnostic bacteriology provide more than one function. For example, MacConkey agar is both differential and selective or combination media because it will not allow most gram-positive bacteria to grow. Another example is sheep blood agar. This is the most commonly used nutritive medium for diagnostic bacteriology because it allows many organisms to grow. However, in many ways this agar is also differential because the appearance of colonies produced by certain bacterial species is readily distinguishable, as indicated in Figure 5-2. Figure 7-5 shows differential hemolytic patterns by various organisms.
Summary of Artificial Media for Routine Bacteriology
Various broth and agar media that have enrichment, selective, or differential capabilities and are used frequently for routine bacteriology are listed alphabetically in Table 7-1. Anaerobic bacteriology (Section 13), mycobacteriology (Section 14), and mycology (Chapter 60) use similar media strategies; details regarding these media are provided in the appropriate chapters.
TABLE 7-1
Plating Media for Routine Bacteriology
Medium | Components/Comments | Primary Purpose |
Bile esculin agar (BEA) | Nutrient agar base with ferric citrate. Hydrolysis of esculin by group D streptococci imparts a brown color to medium; sodium desoxycholate inhibits many bacteria | Differential isolation and presumptive identification of group D streptococci and enterococci |
Bile esculin azide agar with vancomycin | Contains azide to inhibit gram-negative bacteria, vancomycin to select for resistant gram-positive bacteria, and bile esculin to differentiate enterococci from other vancomycin-resistant bacteria that may grow | Selective and differential for cultivation of vancomycin-resistant enterococci from clinical and surveillance specimens |
Blood agar | Trypticase soy agar, Brucella agar, or beef heart infusion with 5% sheep blood | Cultivation of nonfastidious microorganisms, determination of hemolytic reactions |
Bordet-Gengou agar | Potato-glycerol–based medium enriched with 15%-20% defibrinated blood; contaminants inhibited by methicillin (final concentration of 2.5 µm/mL) | Isolation of Bordetella pertussis and Bordetella parapertussis |
Brain heart infusion agar or broth | Dextrose, pork brain and heart dehydrated infusions. | Cultivation of fastidious organisms. |
Buffered charcoal-yeast extract agar (BCYE) | Yeast extract, agar, charcoal, and salts supplemented with L-cysteine HCl, ferric pyrophosphate, ACES buffer, and α-ketoglutarate | Enrichment for Legionella spp. Supports the growth of Francisella and Nocardia spp. |
Buffered charcoal-yeast extract (BCYE) agar with antibiotics | BCYE supplemented with polymyxin B, vancomycin, and ansamycin, to inhibit gram-negative bacteria, gram-positive bacteria, and yeast, respectively | Enrichment and selection for Legionella spp. |
Burkholderia cepacia selective agar | Bile salts, gentamycin, ticarcillin, polymixin B, Peptone, yeast extract | For recovery of B. Cepacia from cystic fibrosis patients |
Campy-blood agar | Contains vancomycin (10 mg/L), trimethoprim (5 mg/L), polymyxin B (2500 U/L), amphotericin B (2 mg/L), and cephalothin (15 mg/L) in a Brucella agar base with sheep blood | Selective for Campylobacter spp. |
Campylobacter thioglycollate broth | Thioglycollate broth supplemented with increased agar concentration and antibiotics | Selective holding medium for recovery of Campylobacter spp. Incubated at 4° C for cold-enrichment. |
CDC anaerobe 5% sheep blood agar | Tryptic soy broth, 5% sheep blood and added nutrients | Improved growth of obligate, slow-growing anaerobes |
Cefoperazone, vancomycin, amphotericin (CVA) medium | Blood-supplemented enrichment medium containing cefoperazone, vancomycin, and amphotericin to inhibit growth of most gram-negative bacteria, gram-positive bacteria, and yeast, respectively | Selective medium for isolation of Campylobacter spp. |
Cefsulodin-irgasan-novobiocin (CIN) agar | Peptone base with yeast extract, mannitol, and bile salts; supplemented with cefsulodin, irgasan, and novobiocin; neutral red and crystal violet indicators | Selective for Yersinia spp.; may be useful for isolation of Aeromonas spp. |
Chocolate agar | Peptone base, enriched with solution of 2% hemoglobin or IsoVitaleX (BBL) | Cultivation of fastidious microorganisms such as Haemophilus spp., Brucella spp. and pathogenic Neisseria spp. |
Chromogenic media | Organism-specific nutrient base, selective supplements and chromogenic substrate | Chromogenic media are designed to optimize growth and differentiate a specific type of organism. Chromagars are routinely used in the identification of yeasts, methicillin-resistant Stapylococcus aureus (MRSA), and a variety of other organisms. |
Columbia colistin-nalidixic acid (CNA) agar | Columbia agar base with 10 mg colistin per liter, 15 mg nalidixic acid per liter, and 5% sheep blood | Selective isolation of gram-positive cocci |
Cystine-tellurite blood agar | Infusion agar base with 5% sheep blood; reduction of potassium tellurite by Corynebacterium diphtheriae produces black colonies | Isolation of C. diphtheriae |
Eosin methylene blue (EMB) agar (Levine) | Peptone base containing lactose; eosin Y and methylene blue as indicators | Isolation and differentiation of lactose-fermenting and non–lactose-fermenting enteric bacilli |
Gram-negative broth (GN) | Peptone base broth with glucose and mannitol; sodium citrate and sodium desoxycholate act as inhibitory agents | Selective (enrichment) liquid medium for enteric pathogens |
Hektoen enteric (HE) agar | Peptone base agar with bile salts, lactose, sucrose, salicin, and ferric ammonium citrate; indicators include bromthymol blue and acid fuchsin | Differential, selective medium for the isolation and differentiation of Salmonella and Shigella spp. from other gram-negative enteric bacilli |
Loeffler’s medium | Animal tissue (heart muscle), dextrose, eggs and beef serum, and sodium chloride | Isolation and growth of Corynebacterium |
MacConkey agar | Peptone base with lactose; gram-positive organisms inhibited by crystal violet and bile salts; neutral red as indicator | Isolation and differentiation of lactose fermenting and non–lactose-fermenting enteric bacilli |
MacConkey sorbitol agar | A modification of MacConkey agar in which lactose has been replaced with d-sorbitol as the primary carbohydrate | For the selection and differentiation of E. coli O157:H7 in stool specimens |
Mannitol salt agar | Peptone base, mannitol, and phenol red indicator; salt concentration of 7.5% inhibits most bacteria | Selective differentiation of staphylococci |
New York City (NYC) agar | Peptone agar base with cornstarch, supplemented with yeast dialysate, 3% hemoglobin, and horse plasma; antibiotic supplement includes vancomycin (2 µg/mL), colistin (5.5 µg/mL), amphotericin B (1.2 µg/mL), and trimethoprim (3 µg/mL) | Selective for Neisseria gonorrhoeae; also supports the growth of Ureaplasma urealyticum and some Mycoplasma spp. |
Phenylethyl alcohol (PEA) agar | Nutrient agar base. Phenylmethanol inhibits growth of gram-negative organisms | Selective isolation of aerobic gram-positive cocci and bacilli and anaerobic gram-positive cocci and negative bacilli |
Regan Lowe | Charcoal agar supplemented with horse blood, cephalexin, and amphotericin B | Enrichment and selective medium for isolation of Bordetella pertussis |
Salmonella-Shigella (SS) agar | Peptone base with lactose, ferric citrate, and sodium citrate; neutral red as indicator; inhibition of coliforms by brilliant green and bile salts | Selective for Salmonella and some Shigella spp. |
Schaedler agar | Peptone and soy protein base agar with yeast extract, dextrose, and buffers; addition of hemin, l-cystine, and 5% blood enriches for anaerobes | Nonselective medium for the recovery of anaerobes and aerobes Selective for Campylobacter and Helicobacter spp. |
Selenite broth | Peptone base broth; sodium selenite toxic for most Enterobacteriaceae | Enrichment of isolation of Salmonella spp. |
Skirrow agar | Peptone and soy protein base agar with lysed horse blood; vancomycin inhibits gram-positive organisms; polymyxin B and trimethoprim inhibit most gram-negative organisms | Selective for Campylobacter spp. |
Streptococcal selective agar (SSA) | Contains crystal violet, colistin, and trimethoprim- sulfamethoxazole in 5% sheep blood agar base | Selective for Streptococcus pyogenes and Streptococcus agalactiae |
Tetrathionate broth | Peptone base broth; iodine and potassium iodide, bile salts, and sodium thiosulfate inhibit gram-positive organisms and Enterobacteriaceae | Selective for Salmonella and Shigella spp. except S. typhi. |
Thayer-Martin agar (modified Thayer Martin) |
Blood agar base enriched with hemoglobin and supplement B; contaminating organisms inhibited by colistin, nystatin, vancomycin, and trimethoprim | Selective for N. gonorrhoeae and N. meningitidis. Supports the growth of Francisella and Brucella spp. |
Thioglycollate broth | Pancreatic digest of casein, soy broth, and glucose enrich growth of most microorganisms; includes reducing agents thioglycolate, cystine, and sodium sulfite; semisolid medium with a low concentration of agar reducing oxygen diffusion in the medium | Supports growth of anaerobes, aerobes, microaerophilic, and fastidious microorganisms |
Thiosulfate citrate-bile salts (TCBS) agar | Peptone base agar with yeast extract, bile salts, citrate, sucrose, ferric citrate, and sodium thiosulfate; bromthymol blue acts as indicator | Selective and differential for Vibrio spp. |
Todd-Hewitt broth supplemented with antibiotics (LIM) | Todd-Hewitt, an enrichment broth for streptococci, is supplemented with nalidixic acid and gentamicin or colistin for greater selectivity; thioglycollate and agar reduce redox potential | Selection and enrichment for Streptococcus agalactiae in female genital specimens |
Trypticase soy broth (TSB) | All-purpose enrichment broth that can support the growth of many fastidious and nonfastidious bacteria | Enrichment broth used for subculturing various bacteria from primary agar plates |
Xylose lysine desoxycholate (XLD) agar | Yeast extract agar with lysine, xylose, lactose, sucrose, and ferric ammonium citrate; sodium desoxycholate inhibits gram-positive organisms; phenol red as indicator | Isolation and differentiation of Salmonella and Shigella spp. from other gram-negative enteric bacilli |
Brain-Heart Infusion.
Brain-heart infusion (BHI) is a nutritionally rich medium used to grow various microorganisms, either as a broth or as an agar, with or without added blood. Key ingredients include infusion from several animal tissue sources, added peptone (protein), phosphate buffer, and a small concentration of dextrose. The carbohydrate provides a readily accessible source of energy for many bacteria. BHI broth is often used as a major component of the media developed for culturing a patient’s blood for bacteria (see Chapter 69), for establishing bacterial identification, and for certain tests to determine bacterial susceptibility to antimicrobial agents (see Chapter 12).
Hektoen Enteric (HE) Agar.
Hektoen enteric (HE) agar contains bile salts and dyes (bromthymol blue and acid fuchsin) to selectively slow the growth of most nonpathogenic gram-negative bacilli found in the gastrointestinal tract and allow Salmonella spp. and Shigella spp. to grow. The medium is also differential because many non-enteric pathogens that do grow will appear as orange to salmon-colored colonies. This colony appearance results from the organism’s ability to ferment the lactose in the medium, resulting in the production of acid, which lowers the medium’s pH and causes a change in the pH indicator bromthymol blue. Salmonella spp. and Shigella spp. do not ferment lactose, so no color change occurs and their colonies maintain the original blue-green color of the medium. As an additional differential characteristic, the medium contains ferric ammonium citrate, an indicator for the detection of H2S, so that H2S-producing organisms, such as Salmonella spp., can be visualized as colonies exhibiting a black precipitate (Figure 7-6).
MacConkey Agar.
MacConkey agar is the most frequently used primary selective and differential agar. This medium contains crystal violet dye to inhibit the growth of gram-positive bacteria and fungi, and it allows many types of gram-negative bacilli to grow. The pH indicator, neutral red, provides this medium with a differential capacity. Bacterial fermentation of lactose results in acid production, which decreases medium pH and causes the neutral red indicator to give bacterial colonies a pink to red color. Non–lactose-fermenters, such as Shigella spp., remain colorless and translucent (see Figure 7-4).
Phenylethyl Alcohol (PEA) Agar.
Phenylethyl alcohol (PEA) agar is essentially sheep blood agar that is supplemented with phenylethyl alcohol to inhibit the growth of gram-negative bacteria. Five percent sheep blood in PEA provides nutrients for common gram-positive cocci such as enterococci, streptococci, and staphylococci (see Figure 7-3). PEA agar, although it contains sheep blood, should not be used in the interpretation of hemolytic reactions.
Thioglycollate Broth.
Gram-negative, facultatively anaerobic bacilli (i.e., those that can grow in the presence or absence of oxygen) generally produce diffuse, even growth throughout the broth, whereas gram-positive cocci demonstrate flocculation or clumps. Strict aerobic bacteria (i.e., require oxygen for growth), such as Pseudomonas spp., tend to grow toward the surface of the broth, whereas strict anaerobic bacteria (i.e., those that cannot grow in the presence of oxygen) grow at the bottom of the broth (Figure 7-7).
Xylose-Lysine-Desoxycholate (XLD) Agar.
As with HE agar, xylose-lysine-desoxycholate (XLD) agar is selective and differential for Shigella spp. and Salmonella spp. The salt, sodium desoxycholate, inhibits many gram-negative bacilli that are not enteric pathogens and inhibits gram-positive organisms. A phenol red indicator in the medium detects increased acidity from carbohydrate (i.e., lactose, xylose, and sucrose) fermentation. Enteric pathogens, such as Shigella spp., do not ferment these carbohydrates, so their colonies remain colorless (i.e., the same approximate pink to red color of the un-inoculated medium). Even though they often ferment xylose, colonies of Salmonella spp. are also colorless on XLD, because of the decarboxylation of lysine, which results in a pH increase that causes the pH indicator to turn red. These colonies often exhibit a black center that results from Salmonella spp. producing H2S. Several of the nonpathogenic organisms ferment one or more of the sugars and produce yellow colonies (Figure 7-8).
Preparation of Artificial Media
Media Sterilization.
Delicate media components that cannot withstand steam sterilization by autoclaving (e.g., serum, certain carbohydrate solutions, certain antibiotics, and other heat-labile substances) can be sterilized by membrane filtration. Passage of solutions through membrane filters with pores ranging in size from 0.2 to 0.45 µm in diameter will not remove viruses but does effectively remove most bacterial and fungal contaminants. Finally, all media, whether purchased or prepared, must be subjected to stringent quality control before being used in the diagnostic setting (for more information regarding quality control see Chapter 79).
Cell Cultures.
The cultures for growth of these bacteria comprise layers of living cells growing on the surface of a solid matrix such as the inside of a glass tube or the bottom of a plastic flask. The presence of bacterial pathogens within the cultured cells is detected by specific changes in the cells’ morphology. Alternatively, specific stains, composed of antibody conjugates, may be used to detect bacterial antigens within the cells. Cell cultures may also detect certain bacterial toxins (e.g., Clostridium difficile cytotoxin). Cell cultures are most commonly used in diagnostic virology. Cell culture maintenance and inoculation is addressed in Chapter 66.
Environmental Requirements
Methods for Providing Optimum Incubation Conditions
Although heating blocks and temperature-controlled water baths may be used occasionally, incubators are the primary laboratory devices used to provide the environmental conditions required for cultivating microorganisms. The conditions of incubators can be altered to accommodate the type of organisms to be grown. This section focuses on the incubation of routine bacteriology cultures. Conditions for growing anaerobic bacteria (Section 13), mycobacteria (Section 14), fungi (Chapter 59), and viruses (Chapter 65) are covered in other areas of the text.
Bacterial Cultivation
Isolation of Bacteria From Specimens
The cultivation of bacteria from infections at various body sites is accomplished by inoculating processed specimens directly onto artificial media. The media are summarized in Table 7-1 and incubation conditions are selected for their ability to support the growth of the bacteria most likely to be involved in the infectious process.
To enhance the growth, isolation, and selection of etiologic agents, specimen inocula are usually spread over the surface of plates in a standard pattern so that individual bacterial colonies are obtained and semi-quantitative analysis can be performed. A commonly used streaking technique is illustrated in Figure 7-9. Using this method, the relative numbers of organisms in the original specimen can be estimated based on the growth of colonies past the original area of inoculation. To enhance isolation of bacterial colonies, the loop should be flamed for sterilization between the streaking of each subsequent quadrant.
Streaking plates inoculated with a measured amount of specimen, such as when a calibrated loop is used to quantify colony-forming units (CFUs) in urine cultures, is accomplished by spreading the inoculum down the center of the plate. Without flaming the loop, the plate is then streaked side to side across the initial inoculum to evenly distribute the growth on the plate (Figure 7-10). This facilitates counting colonies by ensuring that individual bacterial cells will be well dispersed over the agar surface. Typically a calibrated loop of 1 µL is used for urine cultures. However, in situations where a lower count of bacteria may be present such as a suprapubic aspiration, a 10 µL loop may be needed to identify the lower count of organisms. The number of colonies identified on the plate is multiplied by the dilution factor in order to determine the number of colony-forming units per millimeter in the original specimen (103 for a 1 µL loop and 102 for a 10 µL loop). In addition, to standardize the interpretation of colony count, a laboratory should have guidelines for the reporting of organisms based on the number and types of organisms present. A sample standardized method is outlined in Procedure 73-1.
Evaluation of Colony Morphologies
Relative Quantities of Each Colony Type.
The predominance of a bacterial isolate is often used as one of the criteria, along with direct smear results, organism virulence, and the body site from which the culture was obtained, for establishing the organism’s clinical significance. Several methods are used for semiquantitation of bacterial quantities including many, moderate, few or a numerical designation (4+, 3+, 2+) based on the number of colonies identified in each streak area (Table 7-2).
TABLE 7-2
Semi-Quantitation Grading Procedure for Bacterial Isolates on Growth Media
NUMBER OF COLONIES VISIBLE IN EACH QUADRANT | ||||
Score | #1 (Initial Quadrant) | #2 | #3 | #4 |
1+ | Less than 10 | |||
2+ | Less than 10 | Less than 10 | ||
3+ | Greater than 10 | Greater than 10 | Less than 10 | |
4+ | Greater than 10 | Greater than 10 | Greater than 10 | Greater than 5 |
Note: This is a general guideline. Individual laboratories may vary in the methods used for quantitation.
Colony Characteristics.
• Colony size (usually measured in millimeters or described in relative terms such as pinpoint, small, medium, large)
• Colony shape (includes form, elevation, and margin of the colony [Figure 7-11])
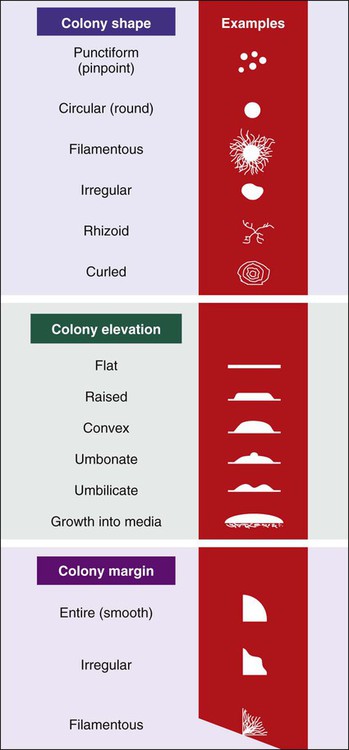
• Colony surface appearance (e.g., glistening, opaque, dull, dry, transparent)
• Changes in agar media resulting from bacterial growth (e.g., hemolytic pattern on blood agar, changes in color of pH indicators, pitting of the agar surface; for examples, see Figures 7-3 through 7-8)
• Odor (certain bacteria produce distinct odors that can be helpful in preliminary identification)
Gram Stain and Subcultures.
Following characterization of growth on primary plating media, all subsequent procedures for definitive identification require the use of pure cultures (i.e., cultures containing one strain of a single species). If sufficient inocula for testing can be obtained from the primary media, then a subculture is not necessary, except as a precaution to obtain more of the etiologic agent if needed and to ensure that a pure inoculum has been used for subsequent tests (i.e., a “purity” check). However, frequently the primary media do not yield sufficient amounts of bacteria in pure culture and a subculture step is required (Figure 7-12).
Principles of Identification
• Determining the clinical significance of a particular pathogen (e.g., is the isolate a pathogen or a contaminant?)
• Guiding physician care of the patient
• Determining whether laboratory testing for detection of antimicrobial resistance is warranted
• Determining the type of antimicrobial therapy that is appropriate
• Determining whether the antimicrobial susceptibility profiles are unusual or aberrant for a particular bacterial species
• Determining whether the infecting organism is a risk for other patients in the hospital, the public, or laboratory workers (i.e., is the organism one that may pose problems for infection control, public health, or laboratory safety?)
• Collecting epidemiologic data to monitor the control and transmission of organisms
Organism Identification Using Genotypic Criteria
Genotypic identification methods involve characterization of some portion of a bacterium’s genome using molecular techniques for DNA or RNA analysis. This usually involves detecting the presence of a gene, or a part thereof, or an RNA product that is specific for a particular organism. In principle, the presence of a specific gene or a particular nucleic acid sequence unique to the organism is interpreted as a definitive identification of the organism. The genotypic approach is highly specific and often very sensitive. Specificity refers to the percentage of patients without disease that will test negative for the presence of the organism. Sensitivity indicates the percentage of patients in whom the organism is present who actually test positive. With the ever-expanding list of molecular techniques being developed, the genetic approach to organism identification will continue to grow and become more integrated into diagnostic microbiology laboratory protocols (for more information regarding molecular methods, see Chapter 8).
Organism Identification Using Phenotypic Criteria
Phenotypic criteria are based on observable physical or metabolic characteristics of bacteria—that is, identification is through analysis of gene products rather than through the genes themselves. The phenotypic approach is the classic approach to bacterial identification, and most identification strategies are still based on bacterial phenotype. Other characterizations are based on the antigenic makeup of the organisms and involve techniques based on antigen-antibody interactions (for more information regarding immunologic diagnosis of infectious diseases, see Chapter 10). However, most of the phenotypic characterizations used in diagnostic bacteriology are based on tests that establish a bacterial isolate’s morphology and metabolic capabilities. The most commonly used phenotypic criteria include the following:
• Microscopic morphology and staining characteristics
• Macroscopic (colony) morphology, including odor and pigmentation
• Environmental requirements for growth
Microscopic Morphology and Staining Characteristics
Microscopic evaluation of bacterial cellular morphology, as facilitated by the Gram stain or other enhancing methods discussed in Chapter 6, provides the most basic and important information on which final identification strategies are based. Based on these findings, most clinically relevant bacteria can be divided into four distinct groups: gram-positive cocci, gram-negative cocci, gram-positive bacilli, and gram-negative bacilli (Figure 7-13). Some bacterial species are morphologically indistinct and are described as “gram-negative coccobacilli,” “gram-variable bacilli,” or pleomorphic (i.e., exhibiting various shapes). Still other morphologies include curved or rods and spirals.
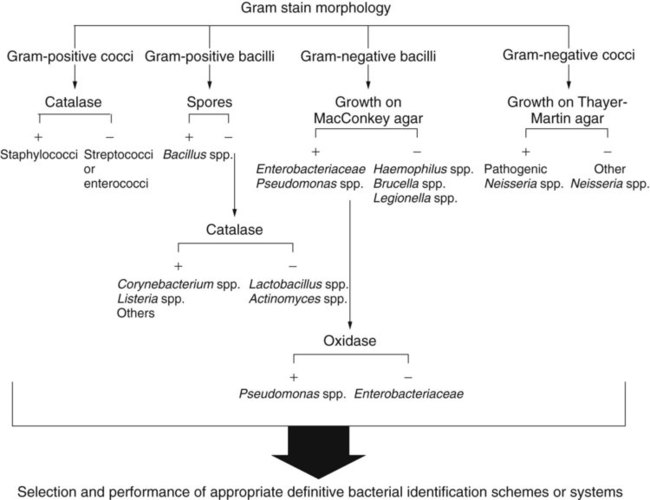
Even without staining, examination of a wet preparation of bacterial colonies under oil immersion (1000× magnification) can provide clues as to possible identity. For example, a wet preparation prepared from a translucent, alpha-hemolytic colony on blood agar may reveal cocci in chains, a strong indication that the bacteria are probably streptococci. Also, the presence of yeast, whose colonies can closely mimic bacterial colonies but whose cells are generally much larger, can be determined (Figure 7-14).
In most instances, schemes for final identification are based on the cellular morphologies and staining characteristics of bacteria. To illustrate, an abbreviated identification flowchart for commonly encountered bacteria is shown in Figure 7-13 (more detailed identification schemes are presented throughout Part III); this flowchart simply illustrates how information about microorganisms is integrated into subsequent identification schemes that are based on the organism’s nutritional requirements and metabolic capabilities. In certain cases, staining characteristics alone are used to definitively identify a bacterial species. Examples are mostly restricted to the use of fluorescent-labeled specific antibodies and fluorescent microscopy to identify organisms such as Legionella pneumophila and Bordetella pertussis.
Resistance or Susceptibility to Antimicrobial Agents
The ability of an organism to grow in the presence of certain antimicrobial agents or specific toxic substances is widely used to establish preliminary identification information. This is accomplished by using agar media supplemented with inhibitory substances or antibiotics (for examples, see Table 7-1) or by directly measuring an organism’s resistance to antimicrobial agents that may be used to treat infections (for more information regarding antimicrobial susceptibility testing, see Chapter 12).
Directly testing a bacterial isolate’s susceptibility to a particular antimicrobial agent may be a very useful part of an identification scheme. Many gram-positive bacteria (with a few exceptions, such as certain Enterococci, Lactobacillus, Leuconostoc, and Pediococcus spp.) are susceptible to vancomycin, an antimicrobial agent that acts on the bacterial cell wall. In contrast, most clinically important gram-negative bacteria are resistant to vancomycin. Therefore, when organisms with uncertain Gram stain results are encountered, susceptibility to vancomycin can be used to help establish the organism’s Gram “status.” Any zone of inhibition around a vancomycin-impregnated disk after overnight incubation is usually indicative of a gram-positive bacterium (Figure 7-15). With few exceptions (e.g., certain Chryseobacterium, Moraxella, or Acinetobacter spp. isolates may be vancomycin susceptible), truly gram-negative bacteria are resistant to vancomycin. Conversely, most gram-negative bacteria are susceptible to the antibiotics colistin or polymyxin, whereas gram-positive bacteria are frequently resistant to these agents.
Nutritional Requirements and Metabolic Capabilities
Establishing Enzymatic Capabilities.
As discussed in Chapter 2, enzymes are the driving force in bacterial metabolism. Because enzymes are genetically encoded, the enzymatic content of an organism is a direct reflection of the organism’s genetic makeup, which, in turn, is specific for individual bacterial species.
Single Enzyme Tests.
Several tests are commonly used to determine the presence of a single enzyme. These tests usually provide rapid results because they can be performed on organisms already grown in culture. Of importance, these tests are easy to perform and interpret and often play a key role in the identification scheme. Although most single enzyme tests do not yield sufficient information to provide species identification, they are used extensively to determine which subsequent identification steps should be followed. For example, the catalase test can provide pivotal information and is commonly used in schemes for gram-positive identifications. The oxidase test is of comparable importance in identification schemes for gram-negative bacteria (see Figure 7-13).
Catalase Test.
Because the catalase test is key to the identification scheme of many gram-positive organisms, interpretation must be done carefully. For example, staphylococci are catalase-positive, whereas streptococci and enterococci are negative; similarly, the catalase reaction differentiates Listeria monocytogenes and corynebacteria (catalase-positive) from other gram-positive, non–spore-forming bacilli (see Figure 7-13).
Oxidase Test.
The test is initially used for differentiating between groups of gram-negative bacteria. Among the commonly encountered gram-negative bacilli, Enterobacteriaceae, Stenotrophomonas maltophilia, and Acinetobacter spp. are oxidase-negative, whereas many other bacilli, such as Pseudomonas spp. and Aeromonas spp., are positive (see Figure 7-13). The oxidase test is also a key reaction for the identification of Neisseria spp. (oxidase-positive).
Oxidation and Fermentation Tests.
As discussed in Chapter 2, bacteria use various metabolic pathways to produce biochemical building blocks and energy. For most clinically relevant bacteria, this involves utilization of carbohydrates (e.g., sugar or sugar derivatives) and protein substrates. Determining whether substrate utilization is an oxidative or fermentative process is important for the identification of several different bacteria.
As shown in Figure 7-16, when acid production is detected in both tubes, the organism is identified as a glucose fermenter because fermentation can occur with or without oxygen. If acid is only detected in the open, aerobic tube, the organism is characterized as a glucose-oxidizer. As a third possibility, some bacteria do not use glucose as a substrate and no acid is detected in either tube (a nonutilizer). The glucose fermentative or oxidative capacity is generally used to separate organisms into major groups (e.g., Enterobacteriaceae are fermentative; Pseudomonas spp. are oxidative). However, the utilization pattern for several other carbohydrates (e.g., lactose, sucrose, xylose, maltose) is often needed to help identify an organism’s genus and species.
Amino Acid Degradation.
Organisms are inoculated into the tube medium that is then overlaid with mineral oil to ensure anaerobic conditions (see Chapter 13). Early during incubation, bacteria utilize the glucose and produce acid, resulting in a yellow coloration of the pH indicator. Organisms that can decarboxylate the amino acid then begin to attack the substrate and produce the amine product, which increases the pH and changes the indicator back from yellow to purple (if bromcresol purple is the pH indicator used; red if phenol red is the indicator). Therefore, after overnight incubation, a positive test is indicated by a purple color and a negative test (i.e., lack of decarboxylase activity) is indicated by a yellow color. With each amino acid tested, a control tube of the glucose-containing broth base without amino acid is inoculated. The standard’s (control) color is compared with that of the tube containing the amino acid following incubation.
Establishing Inhibitor Profiles.
• Growth in the presence of various NaCl concentrations (identification of Enterococci and Vibrio spp.)
• Susceptibility to Optochin and solubility in bile (identification of Streptococcus pneumoniae)
• Ability to hydrolyze esculin in the presence of bile (identification of Enterococci spp. in combination with NaCl)
Principles of Phenotype-Based Identification Schemes
As shown in Figure 7-13, growth characteristics, microscopic morphologies, and single test results are used to categorize most bacterial isolates into general groups. However, the definitive identification to species requires use of schemes designed to produce metabolic profiles of the organisms. Identification systems usually consist of four major components (Figure 7-17):
• Selection and inoculation of a set (i.e., battery) of specific metabolic substrates and growth inhibitors
• Incubation to allow substrate utilization to occur or to allow growth inhibitors to act
• Determination of metabolic activity that occurred during incubation
• Analysis of metabolic profiles and comparison with established profile databases for known bacterial species to establish definitive identification
Incubation for Substrate Utilization
Rapid Identification
Still other rapid identification schemes are based on antigen-antibody reactions, such as latex agglutination tests, that are commonly used to quickly and easily identify certain beta-hemolytic streptococci and S. aureus (for more information regarding these test formats, see Chapter 10).
Analysis of Metabolic Profiles
The metabolic profile obtained with a particular bacterial isolate is essentially the phenotypic fingerprint, or signature, of that organism. Typically, the profile is recorded as a series of pluses (+) for positive reactions and minuses (–) for negative or nonreactions (Figure 7-18). Although this profile by itself provides little information, microbiologists can compare the profile with an extensive identification database to establish the identity of that specific isolate.
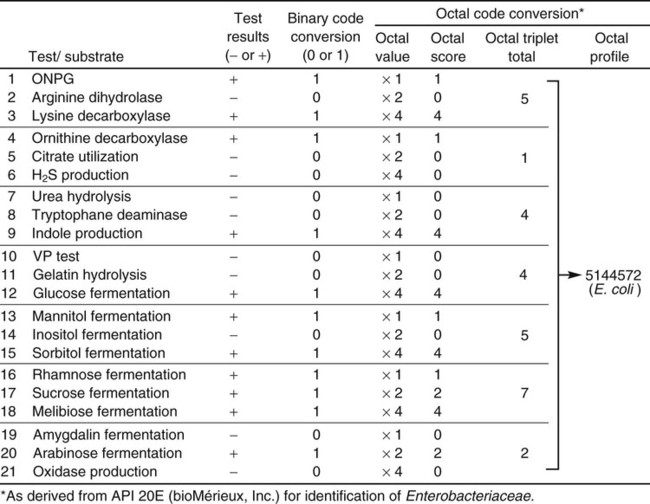
Identification Databases
The first step in developing a database is to accumulate many bacterial strains of the same species. Each strain is inoculated to an identical battery of metabolic tests to generate a positive-negative test profile. The cumulative results of each test are expressed as a percentage of each genus or species that possesses that characteristic. For example, suppose that 100 different known E. coli strains and 100 known Shigella spp. strains are tested in four biochemicals, yielding the results illustrated in Table 7-3. In reality, many more strains and tests would be performed. However, the principle—to generate a database for each species that contains the percentage probability for a positive result with each test in the battery—is the same.
TABLE 7-3
BIOCHEMICAL PARAMETER | ||||
Organism | Lactose | Sucrose | Indole | Ornithine |
Escherichia | 91 | 49 | 99 | 63 |
Shigella | 1 | 1 | 38 | 20 |
Use of the Database to Identify Unknown Isolates
To exemplify this step in the identification process, a binary code conversion system that uses the numerals 0 and 1 to represent negative and positive metabolic reactions, respectively, is used as an example (although other strategies are now used). As shown in Figure 7-18, using binary code conversion, a 21-digit binomial number (e.g., 101100001001101111010, as read from top to bottom in the figure) is produced from the test result. This number is then used in an octal code conversion scheme to produce a mathematic number (octal profile [see Figure 7-18]). The octal profile number is used to generate a numerical profile distinctly related to a specific bacterial species. As shown in Figure 7-18, the octal profile for the unknown organism is 5144572. This profile would then be compared with database profiles to determine the most likely identity of the organism. In this example, the octal profile indicates the unknown organism is E. coli.
Confidence in Identification.
For example, unknown organism X is tested against the four biochemicals listed in Table 7-3 and yields results as follows: lactose (+), sucrose (+), indole (–), and ornithine (+). Based on the results of each test, the percentage of known strains in the database that produced positive results are used to calculate the percentage probability that strain X is a member of one of the two genera (Escherichia or Shigella) given in the example (Table 7-4). Therefore, if 91% of Escherichia spp. are lactose-positive (see Table 7-3), the probability that X is a species of Escherichia based on lactose alone is 0.91. If 38% of Shigella spp. are indole positive (see Table 7-3), then the probability that X is a species of Shigella based on indole alone is 0.62 (1.00 [all Shigella] – 0.38 [percent positive Shigella] = 0.62 [percent of all Shigella that are indole negative]). The probabilities of the individual tests are then multiplied to achieve a calculated likelihood that X is one of these two genera. In this example, X is more likely to be a species of Escherichia, with a probability of 357:1 (1 divided by 0.0028; see Table 7-4). This is still a very unlikely probability for correct identification, but only four parameters were tested, and the indole result was atypical. As more parameters are added to the formula, the importance of just one test decreases and the overall pattern prevails.
TABLE 7-4
BIOCHEMICAL PARAMETER | ||||
Organism | Lactose | Sucrose | Indole | Ornithine |
X | + | + | – | + |
Escherichia | 0.91 | 0.49 | 0.01 | 0.63 |
Shigella | 0.01 | 0.01 | 0.62 | 0.20 |
Probability that X is Escherichia = 0.91 × 0.49 × 0.01 × 0.63 = 0.002809.
Probability that X is Shigella = 0.01 × 0.01 × 0.62 × 0.20 = 0.000012.
Commercial Identification Systems
Advantages and Examples of Commercial System Designs
Commercially available identification systems have largely replaced compilations of conventional test media and substrates prepared in-house for bacterial identification. This replacement has mostly come about because the design of commercial systems has continuously evolved to maximize the speed and optimize the convenience with which all four identification components shown in Figure 7-17 can be achieved. Because laboratory workload has increased, conventional methodologies have had difficulty competing with the advantages of convenience and updated databases offered by commercial systems. Table 13-1 lists and describes the most common manual and automated bacterial identification systems available.
Another approach is to have substrates dried in plastic cupules that are arranged in series on strips into which a suspension of the test organism is placed (Figure 7-19). For some of these systems, use of a heavy inoculum or use of substrates whose utilization is not dependent on extended bacterial multiplication allows results to be available after 4 to 6 hours of incubation.
Still other identification battery formats have been designed to more fully automate several aspects of the identification process. One example is the use of “cards” that are substantially smaller than most microtiter trays or cupule strips (Figure 7-20). Analogous to the microtiter tray format, these cards contain dried substrates in tiny wells that are resuspended upon inoculation.
Commercial systems are often categorized as either automated or manual. As shown in Table 13-1, various aspects of an identification system can be automated, and these usually include, in whole or in part, the inoculation steps, the incubation and reading of tests, and the analysis of results. However, no strict criteria exist that state how many aspects must be automated for a whole system to be classified as automated. Therefore, whether a system is considered automated can be controversial. Furthermore, regardless of the lack or level of automation, the selection of an identification system ultimately depends on system accuracy and reliability, whether the system meets the needs of the laboratory, and limitations imposed by laboratory financial resources.
Overview of Commercial Systems
Various multitest bacterial identification systems (as listed in Table 13-1) are commercially available for use in diagnostic microbiology laboratories, and the four basic identification components outlined in Figure 7-17 are common to them all. However, different systems vary in their approach to each component. The most common variations involve the following:
• Types and formats of tests included in the test battery
• Method of inoculation (manual or automated)
• Required length of incubation for substrate utilization; this usually depends on whether utilization requires bacterial growth
• Method for detecting substrate utilization and whether detection is manual or automated
• Method of interpreting and analyzing results (manual or computer assisted), and if computer assisted, the extent to which assistance is automated
The general features of some commercial identification systems are summarized in Table 13-1. More specific information is available from the manufacturers.