Chapter 17 Thoracic Aortic Disease
Thoracic aortic diseases are generally surgical problems and require surgical treatment (Table 17-1). Acute aortic dissections, rupturing aortic aneurysms, and traumatic aortic injuries are surgical emergencies. Subacute aortic dissection and expanding aortic aneurysms require urgent surgical intervention. Stable thoracic or thoracoabdominal aortic aneurysms (TAAAs), aortic coarctation, or atheromatous disease causing embolization may be considered for elective surgical repair. Increased public awareness of thoracic aortic disease, early recognition of acute aortic syndromes by emergency medical personnel, improved diagnostic imaging technology for the diagnosis of thoracic aortic disease, and an aging population all contribute to the increased number of patients requiring aortic surgery. Furthermore, improvements in the surgical treatment of thoracic aortic diseases combined with increased treatment options such as endovascular stent repair have led to an increased number of patient referrals to centers specializing in the management of patients with thoracic aortic diseases. Improved treatment and survival after aortic surgical procedures often provide a cure for the original disease but have created new and unique problems. An increasing number of patients who have had prior aortic surgical procedures require reoperation for long-term complications of aortic surgery such as bioprosthetic valve or graft failure, aortic pseudoaneurysm at old vascular graft anastomosis, endocarditis, or progression of the original disease process into native segments of the thoracic aorta.
Table 17-1 Diseases of the Thoracic Aorta That Are Amenableto Surgical Treatment
Adapted from Kouchoukos NT, Dougenis D: Surgery of the aorta. N Engl J Med 336:1876, 1997.
GENERAL CONSIDERATIONS FOR THE PERIOPERATIVE CARE OF AORTIC SURGICAL PATIENTS
Patients undergoing thoracic aortic operations of any type share common considerations for the safe conduct of anesthesia and perioperative care (Table 17-2).
Table 17-2 General Considerations for the Anesthetic Care of Thoracic Aortic Surgical Patients
Preanesthetic Assessment |
Urgency of the operation (emergent, urgent, or elective) |
Pathology and anatomic extent of the disease |
Median sternotomy vs. thoracotomy vs. endovascular approach |
Mediastinal mass effect |
Airway compression or deviation |
Preexisting or Associated Medical Conditions |
Aortic valve disease |
Cardiac tamponade |
Coronary artery stenosis |
Cardiomyopathy |
Cerebrovascular disease |
Pulmonary disease |
Renal insufficiency |
Esophageal disease (contraindications to TEE) |
Coagulopathy |
Prior aortic operations |
Preoperative Medications |
Warfarin (Coumadin) |
Antiplatelet therapy |
Antihypertensive therapy |
Anesthetic Management |
Hemodynamic monitoring |
Proximal aortic pressure |
Distal aortic pressure |
Central venous pressure |
Pulmonary artery pressure and cardiac output |
Transesophageal echocardiography |
Neurophysiologic monitoring |
Electroencephalography (EEG) |
Somatosensory evoked potentials (SSEPs) |
Motor evoked potentials (MEPs) |
Jugular venous oxygen saturation |
Lumbar cerebrospinal fluid pressure |
Body temperature |
Single-lung ventilation for thoracotomy |
Double-lumen endobronchial tube |
Endobronchial blocker |
Potential for bleeding |
Large-bore intravenous access |
Blood product availability |
Antifibrinolytic therapy |
Antibiotic prophylaxis |
Postoperative Care Considerations and Complications |
Hypothermia |
Hypotension |
Hypertension |
Bleeding |
Spinal cord ischemia |
Stroke |
Renal insufficiency |
Respiratory insufficiency |
Phrenic nerve injury |
Diaphragmatic dysfunction |
Recurrent laryngeal nerve injury |
Pain management |
Anesthetic Management
The maintenance of general anesthesia can usually be accomplished with a combination of narcotic analgesics, benzodiazepine sedative hypnotics, an inhaled general anesthetic, and a nondepolarizing muscle relaxant. Anesthetics can be reduced in response to moderate hypothermia in the range of 30°C and then discontinued during deep hypothermia at 18°C and resumed on rewarming. When electroencephalographic (EEG) or somatosensory evoked potential (SSEP) monitoring is required during surgery, barbiturates or bolus doses of propofol are avoided and the dose of the inhaled anesthetic is reduced to 0.5 MAC and kept constant to prevent anesthetic-induced changes in the monitored signals. Propofol, narcotics, and neuromuscular blocking drugs can be used during SSEP monitoring. When intraoperative motor evoked potential (MEP) monitoring is required, total intravenous anesthesia with propofol in combination with remifentanil or similar narcotic without neuromuscular blockade is necessary to ensure consistent reproducible recordings and a good-quality signal. In the majority of cases, the duration of general anesthesia is designed to persist for 1 to 2 hours after patient transfer to the intensive care unit (ICU) to permit a gradual and controlled emergence from general anesthesia. If epidural analgesia is used intraoperatively, a dilute solution of local anesthetic and narcotic is preferred to prevent hypotension caused by sympathetic nervous system blockade and to prevent complete motor or sensory blockade to permit neurologic assessment of lower extremity function.1
The potential for blood loss and bleeding is always a consideration in operations on the thoracic aorta. The presence of intrinsic disease of the vessel wall, construction of numerous vascular anastomoses in large conducting vessels, need for extracorporeal circulation, and application of deliberate hypothermia all combine to create a situation in which blood loss and transfusion therapy are commonplace. Because blood loss can occur rapidly and unpredictably and be difficult to control, it is often prudent to have fresh frozen plasma and platelets available to provide ongoing replacement of coagulation factors during transfusion of packed red blood cells. The time delay required for laboratory testing to verify the depletion of platelets and clotting factors in the setting of ongoing blood loss is often too long to be useful as a guide for transfusion therapy. Strategies to decrease the risk of bleeding and to conserve blood include discontinuation of anticoagulation and antiplatelet therapy before surgery, antifibrinolytic therapy, the routine use of intraoperative cell salvage, biologic glue, and precise control of arterial pressure and prevention of hypertensive episodes in the perioperative period. The antifibrinolytic agents, ε-aminocaproic acid or tranexamic acid, have been safely used in the setting of thoracic aortic surgery with DHCA. The infusion of an antifibrinolytic agent should be discontinued during the period of DHCA and resumed on reperfusion. Recombinant activated factor VIIa is a synthetic hemostatic agent that promotes hemostasis by binding with tissue factor at the site of tissue injury to promote clot formation. Although experience with this agent has been limited, dramatic responses to this drug have been observed in response to coagulopathic bleeding refractory to conventional therapy in the setting of trauma, cardiac, and aortic surgery.2 In the surgical setting, recombinant activated factor VIIa has been administered intravenously in doses up to 90 μg/kg and repeated once after 2 hours. Recombinant activated factor VIIa has an estimated plasma half-life of 2.6 hours and causes a rapid decrease in the prothrombin time.
THORACIC AORTIC ANEURYSM
Most thoracic aortic aneurysms are asymptomatic and discovered incidentally through screening or as a consequence of medical workup for other cardiovascular disease (Box 17-1). The most common initial symptoms of thoracic aortic aneurysm are chest or back pain caused by aneurysmal expansion, rupture, or bony erosion. The mass effect of the aneurysm can cause hoarseness from stretching or compression of the recurrent laryngeal nerve, atelectasis from compression of the left lung, superior vena cava syndrome from compression of the superior vena cava or innominate vein, dysphagia from compression of the esophagus, or dyspnea from compression of the trachea, main stem bronchus, or pulmonary artery. Other symptoms include wheezing, cough, hemoptysis, or hematemesis. Aneurysm of the aortic root causing AR may present as dyspnea on exertion, heart failure, or pulmonary edema. Atherosclerotic aneurysms with mural thrombus may present as embolism, stroke, mesenteric ischemia, renal insufficiency, or limb ischemia.
General Surgical Considerations for Thoracic Aortic Aneurysms
The objective of surgical repair is to replace the aneurysmal segment of aorta with a tube graft to prevent morbidity and mortality as a consequence of aneurysm rupture. Indications for operative repair include the presence of symptoms refractory to medical management, evidence of rupture, an aneurysm diameter of 5.0 to 5.5 cm for an ascending aortic aneurysm, an aneurysm diameter of 6.0 to 7.0 cm for a descending thoracic aneurysm, or an increase in aneurysm diameter greater than or equal to 10 mm/yr. Earlier surgical intervention may be justified in patients with Marfan syndrome, a family history of aortic disease, or dissection. In several series, 1-, 3-, and 5-year survival was as high as 65%, 36%, and 20% for medically treated patients with thoracic aortic aneurysms, respectively. Aneurysm rupture may account for up to 32% to 47% of deaths.3
Surgical Repair of Ascending Aortic and Arch Aneurysms
The surgical options for repair of ascending aortic aneurysms depend on the presence of aortic valve disease, aneurysm of the sinuses of Valsalva, and distal extension of the aneurysm into the aortic arch. Intraoperative TEE is useful for evaluating the aortic valve to determine if a valve-sparing surgery is feasible, to determine the aortic valve annular diameter in relation to the diameter of the sinotubular junction to assess aneurysmal dilation of the aortic root, and to detect and quantify the presence of AR after valve repair. The most common aortic valve diseases associated with ascending aortic aneurysm are bicuspid aortic valve or AR caused by dilation of the aortic root (Fig. 17-1). If the aortic valve and aortic root are normal, a simple tube graft can be used to replace the ascending aorta. If the aortic valve is diseased but the sinuses of Valsalva are normal, an aortic valve replacement combined with a tube graft for the ascending aorta without need for re-implantation of the coronary arteries can be performed. If disease involves the aortic valve, aortic root, and ascending aorta, the options include tube graft of the ascending aorta in combination with aortic valve repair, reconstruction of the aortic root with sparing or repair of the aortic valve, bioprosthetic aortic root replacement, composite valve/graft conduit aortic root replacement (Bentall procedure), or replacement of the aortic root with a pulmonary autograft (Ross procedure). Replacement of the aortic root requires re-implantation of the coronary arteries or aortocoronary bypass grafting (Cabrol technique). If there is evidence of significant coronary artery disease, a combined ascending aortic aneurysm repair and coronary artery bypass grafting (CABG) may be necessary.
Neuroprotection Strategies for Temporary Interruption of Cerebral Blood Flow
Thoracic aortic surgery requiring temporary interruption of cerebral perfusion has been associated with the highest incidence of brain injury in comparison to other cardiac operations. Many reports assessing the incidence of neurologic injury were based on retrospective data collection or clinically diagnosed stroke. These reports suggest an incidence of stroke in the range of 7% to 9%. Studies that included a more detailed neurologic assessment and neuropsychological testing have found that neurologic deficits were more frequent in older patients and those subjected to DHCA for >25 minutes.4
Two major mechanisms are believed to explain the high incidence of stroke and neurocognitive dysfunction related to thoracic aortic operations. The first is cerebral ischemia or infarction caused by hypoperfusion or the need for temporary circulatory arrest during reconstruction of the aortic arch. The second is cerebral ischemia or infarction caused by cerebral embolization as a consequence of extracorporeal circulation or intrinsic vascular disease. Arterial emboli causing stroke can originate from multiple sources during thoracic aortic operations and include air introduced into the circulation from open cardiac chambers, vascular cannulation sites, or arterial anastomosis. Atherosclerotic or particulate debris may be released during clamping and unclamping of the aorta, the creation of anastomoses in the ascending aorta and aortic arch, or the excision of severely calcified and diseased cardiac valves. CPB may result in the generation of platelet aggregates, fat particles, and other microparticulate debris. The turbulent high-velocity blood flow out of the aortic cannula employed for CPB may also dislodge atherosclerotic debris within the aorta. Retrograde blood flow through a diseased descending thoracic aorta as a consequence of CPB conducted with femoral artery cannulation may cause retrograde cerebral embolization. For all these reasons, strategies to provide neurologic protection are important in the conduct of thoracic aortic operations (Box 17-2).
Deep Hypothermic Circulatory Arrest
The brain is exquisitely susceptible to ischemic injury within minutes after the onset of circulatory arrest because it has a high metabolic rate, continuous requirement for metabolic substrate, and limited reserves of high-energy phosphates. In the 1970s, deep hypothermia and circulatory arrest were introduced to protect the brain from ischemic injury for operations on the aortic arch requiring temporary interruption of cerebral blood flow. The physiologic basis for deep hypothermia as a neuroprotection strategy is to decrease cerebral metabolic rate and oxygen demands to increase the period of time that the brain can tolerate circulatory arrest. Existing evidence indicates that autoregulation of cerebral blood flow is maintained during deliberate hypothermia with alpha-stat blood gas management. In adults, a 10°C decrease in body temperature decreases cerebral metabolic rate by an average factor of 2.6, a factor commonly referred to as the Q10 ratio. Assuming an ischemic tolerance of 3 to 5 minutes under normothermic conditions, a Q10 ratio of 2.6 predicts an ischemic tolerance in the range of 20 to 34 minutes at a brain temperature of 17°C or 53 to 88 minutes at a brain temperature of 7°C. More recent estimations of cerebral metabolism in adults undergoing DHCA suggest an ischemic tolerance of 30 minutes at 15°C and 40 minutes at 10°C.5 Direct measurement of cerebral metabolites and brainstem electrical activity in adults undergoing DHCA with RCP at 14°C indicated the onset of cerebral ischemia after only 18 to 20 minutes. It is also possible that hypothermia provides brain protection through mechanisms other than the reduction in cerebral metabolic rate. Despite an incomplete understanding of the mechanism and efficacy of hypothermia for cerebral protection, the large body of experimental evidence and clinical experience with the deliberate hypothermia suggest that it is the single most important intervention for preventing neurologic injury in response to circulatory arrest.
Retrograde Cerebral Perfusion
In 1990, RCP was reported as a means to deliver metabolic substrate to the brain during operations involving the aortic arch that required the interruption of ACP. RCP is performed by infusing cold oxygenated blood into the superior vena cava cannula at a temperature of 8°C to 14°C via the CPB machine. The internal jugular venous pressure is maintained at less than or equal to 25 mmHg to prevent cerebral edema. Internal jugular venous pressure is measured from the introducer port of the internal jugular venous cannula at a site proximal to the superior vena cava perfusion cannula and zeroed at the level of the ear. The patient is positioned in 10 degrees of Trendelenburg to decrease the risk of cerebral air embolism and prevent trapping of air within the cerebral circulation in the presence of an open aortic arch. RCP flow rates of 200 to 600 mL/min can usually be achieved. The potential benefits of RCP for neuroprotection include prolonging the safe period of DHCA by providing some metabolic substrate delivery to the brain, flushing embolic material from the cerebral arterial vasculature to decrease the risk of cerebral embolization on resuming ACP, and providing a means to maintain cerebral hypothermia during DHCA.6,7
DESCENDING THORACIC AND THORACOABDOMINAL AORTIC ANEURYSMS
Thoracic aortic aneurysms and TAAAs are classified according to the anatomic extent of the aneurysmal segment. The most commonly used classification scheme is that described by Crawford that categorizes aneurysm extent into four major groups (Fig. 17-2). Extent I TAAA involves the entire descending thoracic aorta from the origin of the left subclavian artery down to the level of the diaphragm ending above the renal arteries. Extent II TAAA involves the entire descending thoracic aorta with extension across the diaphragm into and through the abdominal aorta all the way to the aortic bifurcation. Extent III TAAA involves the distal half of the descending thoracic aorta, crosses the diaphragm, and involves most of the abdominal aorta. Extent IV TAAA is confined to the upper abdominal aorta.
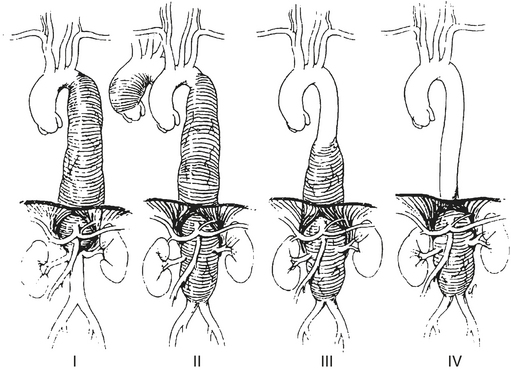
Figure 17-2 Crawford classification of extent of thoracoabdominal aortic aneurysm.
(From Coselli JS: Descending thoracoabdominal aortic aneurysms. In Edmunds LH [ed]: Cardiac Surgery in the Adult. New York, McGraw-Hill, 1997, p 1232.)
Simple Aortic Cross-Clamp Technique
In 1965, Crawford developed the technique of aortic cross-clamping for TAAA repair and subsequently reported a mortality rate of 8.9% in more than 600 TAAA cases repaired with the simple cross-clamp technique. Mortality and paraplegia were related to the position and length of the resected aorta, the condition of the patient at the time of presentation, and the duration of time that the aorta was cross-clamped. A major disadvantage of the simple cross-clamp technique is the obligatory ischemic period to the body and organs distal to the aortic cross-clamp. For this reason, when the simple cross-clamp technique is used, the surgeon must clamp and sew as quickly as possible to limit ischemia time to the spinal cord and distal aortic territory. Theincidence of paraplegia and renal failure has been observed to correlate with the duration of the aortic cross-clamp time and was increased significantly for aortic cross-clamp times greater than 30 minutes. Other disadvantages associated with cross-clamping the descending thoracic aorta include proximal aortic hypertension, bleeding from arterial collaterals, and hemodynamic instability on reperfusion. Proximal aortic hypertension during the period that the aorta is cross-clamped may be poorly tolerated in patients with abnormal left ventricular function, regurgitant cardiac valve disease, or ischemic coronary artery disease. Blood loss as a result of bleeding from arterial collateral vessels can be minimized by the use of intraoperative red blood cell salvaging. Hemodynamic instability during release of the aortic cross-clamp and reperfusion often has to be managed with correction of metabolic acidosis, rapid intravascular volume expansion, vasopressor therapy, or the intermittent reapplication and gradual release of the aortic cross-clamp. Adjuncts to protect end-organ function from ischemia during aortic cross-clamping include mild deliberate hypothermia and selective cooling of the spinal cord. Despite the physiologic consequences, the aortic cross-clamp technique remains a relatively simple technique with proven clinical outcomes and is still favored by many surgeons (Table 17-3).
Table 17-3 Advantages and Disadvantages of Distal Perfusion Techniques for Thoracic or Thoracoabdominal Aortic Reconstruction
Potential Advantages |
Partial Left-Sided Heart Bypass
Partial left-sided heart bypass with extracorporeal circulation is a method of controlling both proximal aortic and distal aortic perfusion during aortic cross-clamping for TAAA repair. The technique of partial left-sided heart bypass involves cannulation of the left atrium from a left thoracotomy incision usually via a left pulmonary vein. Oxygenated blood from the left atrium is directed through the extracorporeal circuit with a centrifugal pump and into an arterial cannula placed in either the distal aorta, iliac artery, or femoral artery.8 The extracorporeal circuit used to provide partial left-sided heart bypass can be modified in a number of ways to include or not include a heat exchanger, membrane oxygenator, or venous reservoir. Heparin requirements for partial left-sided heart bypass range from minimal to no heparin when heparin-coated circuits without oxygenators are used to full systemic anticoagulation with activated coagulation time greater than 400 seconds when circuits with membrane oxygenators and heat exchangers are used. During partial left-sided heart bypass, the mean arterial pressure in the proximal aorta monitored by a radial arterial catheter is generally maintained in the range of 90 mmHg. Bypass flow rates in the range of 1.5 to 2.5 L/min are usually necessary to maintain a distal aortic mean arterial pressure in the range of 60 to 70 mm Hg measured through a femoral arterial catheter.9 Sequential advancement of the distal aortic cross-clamp during partial left-sided heart bypass after successive reattachment of intercostal arteries, mesenteric artery, and the renal arteries to the graft permits segmental reconstruction of the thoracoabdominal aorta to decrease end-organ ischemia. Advantages of partial left-sided heart bypass include the ability to control proximal aortic and distal aortic perfusion pressures, control systemic temperature with a heat exchanger, provide reliable perfusion to the lower body and mesenteric organs, permit selective cannulation and antegrade perfusion of mesenteric branch vessels, and preserve lower extremity somatosensory and motor nerve function for intraoperative neurophysiologic monitoring. Two contemporary clinical series have suggested that use of partial left-sided heart bypass was protective against postoperative paraplegia.10 Disadvantages to partial left-sided heart bypass include increased expense, increased complexity, and requirement for systemic anticoagulation (see Table 17-3).
Cardiopulmonary Bypass and Deep Hypothermic Circulatory Arrest
Full CPB and DHCA has been described for TAAA repair with acceptable morbidity.11 DHCA is necessary for construction of the proximal graft anastomosis if the aneurysm extends into the distal aortic arch or if the proximal descending aorta cannot be cross-clamped because the aorta is heavily calcified or cross-clamping compromises flow in the left common carotid artery. DHCA has also been advocated as an alternative method to protect the spinal cord and mesenteric organs from ischemia before the reattachment of branch vessels. If full CPB and DHCA are planned for TAAA repair through a left thoracotomy incision, preoperative echocardiography or intraoperative TEE is necessary to evaluate for the presence of AR so that a left ventricular vent can be inserted during surgery to prevent overdistention of the left ventricle with the onset of asystole during deliberate hypothermia. DHCA has the advantage of providing a bloodless surgical field for open anastomosis. Potential disadvantages of CPB and DHCA is the limited safe period for circulatory arrest, risk of stroke from cerebral embolization caused by retrograde perfusion of the aortic arch through a diseased descending thoracic aorta, increased duration of CPB for deliberate hypothermia and rewarming, and possibly increased risk of bleeding from hypothermia.
Endovascular Stent Graft Repair of Thoracic Aortic Aneurysms
Endovascular stent grafts are fabric or synthetic tube grafts reinforced by a wire frame that can be collapsed within a catheter for delivery and deployment within the aortic lumen. The endovascular stent graft is designed to be deployed within the aorta to span the length of the aneurysm and exclude blood flow into the aneurysm cavity. Endovascular stent graft repair for thoracic aortic aneurysms has been reported for the repair of isolated descending thoracic aortic aneurysms, repair of distal aortic arch aneurysms with partial arch reconstruction, and a few cases of transverse aortic arch aneurysms with dissection.12 Endovascular stent repair requires the existence of a 1-cm-long nontapered region of aorta on either end of the aneurysm, often called the aneurysm neck, to provide a landing zone for each end of the graft. Furthermore, aneurysms that span essential aortic branch vessels require extra-anatomic bypass of those vessels before endovascular stent grafting.
Paraplegia after Thoracoabdominal Aortic Aneurysm Repair
The most devastating complication of TAAA repair is postoperative paraplegia caused by spinal cord ischemia and infarction. Although the exact pathophysiology and clinical events that lead to paraplegia after TAAA repair are incompletely understood, temporary interruption of distal aortic perfusion and sacrifice of intercostal and segmental arteries to accomplish TAAA repair are central events that predispose the spinal cord to ischemia and subsequent infarction. The incidence of paraplegia or paraparesis after TAAA repair based on clinical series reported in the literature varies widely and ranges from 2.9% to 32%. Factors believed to contribute or influence the risk of postoperative paraplegia include the extent of the TAAA, acute presentation, aneurysm rupture, the duration of ischemia as a consequence of aortic cross-clamping, the loss of critical intercostal arteries, the presence of dissection, the surgical technique used to accomplish the repair, and the use of techniques to protect the spinal cord.13 The extent of the neurologic deficit in paraplegia or paraparesis varies from patient to patient but typically extends from the lumbosacral cord to the mid- to high thoracic level. Postoperative paraplegia is particularly poorly tolerated in elderly patients, is associated with respiratory insufficiency, and has a mortality rate that ranges from 63% to 100% depending on the length of follow-up. Although controversial, it is likely that perioperative management strategies directed at the detection and treatment of spinal cord ischemia have the potential to decrease the risk of paraplegia after TAAA repair (Table 17-4.)
Table 17-4 Strategies to Decrease the Risk of Paraplegia from Spinal Cord Ischemia after Thoracic or Thoracoabdominal Aortic Procedures
Minimize Aortic Cross-Clamp Time |
Distal aortic perfusion |
Passive shunt (Gott) |
Partial left-sided heart bypass |
Partial cardiopulmonary bypass |
Deliberate Hypothermia |
Mild-to-moderate systemic hypothermia (32°C to 35°C) |
Deep hypothermic circulatory arrest (14°C to 18°C) |
Selective spinal cord hypothermia (epidural cooling, 25°C) |
Increase Spinal Cord Perfusion Pressure |
Re-implantation of critical intercostal and segmental arterial branches |
Lumbar cerebrospinal fluid drainage (CSF pressure ≤ 10 mmHg) |
Arterial pressure augmentation (MAP ≥ 85 mmHg) |
Intraoperative Monitoring of Lower Extremity Neurophysiologic Function |
Somatosensory evoked potentials (SSEPs) |
Motor evoked potentials (MEPs) |
Postoperative Neurologic Assessment for Early Detection of Delayed-Onset Paraplegia |
Serial neurologic examinations |
Pharmacologic Neuroprotection |
Glucocorticoid |
Barbiturate or central nervous system depressants |
Magnesium sulfate |
Mannitol |
Naloxone |
Lidocaine |
Intrathecal papaverine |
In immediate-onset paraplegia, the patient is paraplegic on emergence from general anesthesia after TAAA repair. Immediate-onset paraplegia is likely a consequence of spinal cord ischemia leading to infarction that occurred at some time during surgery while the patient was under general anesthesia. In contrast to delayed-onset paraplegia, recovery or response to treatment in immediate-onset paraplegia has not been consistently demonstrated. The lack of improvement in response to measures to improve spinal cord perfusion in immediate-onset paraplegia indicate that irreversible injury had already occurred by the time paraplegia was diagnosed. For this reason, strategies to prevent immediate-onset paraplegia are directed toward protection of the spinal cord from ischemia and infarction during surgery (Box 17-3). Intraoperative efforts to protect the spinal cord from ischemia include augmentation of the arterial pressure, lumbar CSF drainage, provision of distal aortic perfusion with partial left-sided heart bypass, minimization of the ischemic time, deliberate hypothermia, segmental reconstruction of the aorta, re-implantation of intercostal arteries, or pharmacologic neuroprotection. The objective of using intraoperative neurophysiologic monitoring during general anesthesia is to detect spinal cord ischemia to permit immediate interventions to improve spinal cord perfusion during general anesthesia. Distal aortic perfusion maintains spinal cord function during aortic cross-clamping and improves the ability to monitor spinal cord integrity during surgery with SSEPs or MEPs.
Delayed-onset paraplegia or paraparesis is the onset of spinal cord ischemia several hours or days after TAAA repair in a patient who awakes after surgery without evidence of neurologic dysfunction. The syndrome of delayed-onset paraplegia indicates that the spinal cord was successfully protected during TAAA repair but remains vulnerable to ischemia and infarction in the early postoperative period. The abrupt onset of paraplegia or paraparesis in a patient with an initially intact neurologic examination after surgery suggests that immediate interventions to improve spinal cord perfusion may be effective for reversing spinal cord ischemia and preventing or limiting the extent of subsequent infarction. The clinical presentation of delayed-onset paraplegia or paraparesis can be variable and include progressive loss of lower extremity motor strength or sensation. Findings are sometimes asymmetric, affecting one side more than the other. The cause or events provoking delayed-onset paraplegia are not fully understood, but a number of reports suggest that it is often preceded by hypotension. Strategies to prevent and treat delayed-onset paraplegia are directed at preventing hypotension after TAAA repair, early recovery from general anesthesia to permit neurologic assessment and detection of delayed-onset paraplegia, serial monitoring of lower extremity motor and sensory function, and lumbar CSF drainage for the initial 24 to 72 hours after surgery if a lumbar CSF catheter was placed during surgery (Box 17-4). Success in the treatment of delayed-onset paraplegia or paraparesis has been reported in response to immediate interventions directed at increasing the spinal cord perfusion pressure. Full or partial recovery of neurologic function after delayed-onset paraplegia has been reported in response to lumbar CSF drainage, arterial pressure augmentation, and intravenous naloxone used alone or in combination. Reports suggested that early or immediate implementation of treatment at the onset of symptoms was more effective.14 Considering the morbidity and mortality associated with permanent paraplegia after TAAA repair, all reasonable attempts to treat delayed-onset paraplegia can be justified.
Lumbar Cerebrospinal Fluid Drainage
Lumbar CSF drainage is a recognized adjunct for the prevention and treatment of paraplegia caused by spinal cord ischemia after TAAA repair. The physiologic rationale for lumbar CSF drainage is that reduction of lumbar CSF pressure improves spinal cord perfusion pressure. It is also believed that CSF drainage counters an abnormal increase in lumbar CSF pressure in response to aortic cross-clamping, reperfusion, increased central venous pressure, or spinal cord edema. Interpreting the contribution of CSF drainage for the prevention and treatment of postoperative paraplegia has been difficult because any incremental benefit of lumbar CSF drainage must be considered in the context of heterogeneous patient populations, differences in the surgical technique, and lack of control of the arterial pressure. A randomized, controlled trial of 150 patients undergoing extent I and extent II TAAA repair using a uniform surgical technique employing distal aortic perfusion with partial left-sided heart bypass demonstrated that lumbar CSF drainage was associated with an 80% risk reduction of postoperative paraplegia. Two clinical series suggest that lumbar CSF drainage used in combination with arterial pressure augmentation to increase spinal cord perfusion pressure was effective for the treatment of delayed-onset paraplegia when the intervention was employed immediately after the onset of symptoms.9,14
Intraoperative Neurophysiologic Monitoring
The objective of intraoperative neurophysiologic monitoring is to permit the detection of spinal cord ischemia during surgery while the patient is under general anesthesia. The ability to detect intraoperative spinal cord ischemia may permit immediate interventions to improve spinal cord perfusion and prevent immediate-onset postoperative paraplegia. Two neurophysiologic monitoring techniques have been used to detect intraoperative spinal cord ischemia during TAAA repair. SSEP monitoring is performed by applying electrical stimuli to peripheral nerves and recording the evoked potential that is generated at the level of the peripheral nerves, spinal cord, brainstem, thalamus, and cerebral cortex. MEP monitoring is performed by applying paired stimuli to the scalp and recording the evoked potential that is generated in the anterior tibialis muscle.15 Paraplegia caused by spinal cord ischemia causes a decrease in amplitude or disappearance of lower extremity evoked potentials compared with the upper extremity evoked potentials. Comparing the lower to the upper extremity evoked potentials during surgery is useful to distinguish changes caused by spinal cord ischemia from changes caused by the systemic effects of anesthetic agents, hypothermia, or electrical interference. SSEP monitoring can usually be accomplished using a balanced anesthetic technique with narcotic, muscle relaxant, benzodiazepine, and/or propofol and by keeping the inhaled anesthetic concentration less than 0.5 MAC. MEP monitoring requires total intravenous anesthesia without neuromuscular blockade.
Selective Spinal Cord Cooling
In addition to deep hypothermia and moderate systemic hypothermia, selective cooling of the spinal cord by the infusion of cold saline into the epidural space has been described as a technique to protect the spinal cord from ischemia during TAAA repair. The original technique for selective spinal cord cooling described the infusion of 4°C saline at flow rates up to 33 mL/min through a standard 4-Fr epidural catheter inserted at the T11-T12 vertebral interspace. A second 4-Fr thermister-tipped catheter inserted 4 cm into the subarachnoid space at the L3-L4 vertebral interspace was used to measure CSF temperature, measure CSF pressure, and act as a conduit for CSF drainage. A CSF temperature of around 26°C was achieved after the infusion of an average of 489 mL of iced saline into the epidural space over 50 minutes. CSF hypothermia was maintained by the additional infusion of 347 mL of iced saline into the epidural space while the aorta was cross-clamped. CSF was intermittently drained from the subarachnoid catheter to maintain a mean arterial to CSF pressure gradient of 30 to 50 mmHg to ensure spinal cord perfusion. The technique of selective spinal cord cooling is predominantly used in combination with the clamp-and-sew technique but has also been described for use in cases performed with CPB.16 The clinical experience with selective spinal cord cooling has been limited to only a few institutions. A case series of 170 patients undergoing TAAA repair with this technique reported a postoperative paraparesis or paraplegia rate of 7%. A potential problem with the technique was excessive CSF pressures in response to epidural infusion that may have contributed to the development of a proximal cord compression syndrome in two individual patients.
Postoperative Analgesia after Thoracoabdominal Aortic Aneurysm Repair
The usual management of epidural anesthesia and analgesia may need to be modified when applied to the patient undergoing TAAA repair because the complications of epidural analgesia are difficult to distinguish from postoperative paraplegia caused by spinal cord ischemia.17 The epidural analgesia regimen should be formulated to minimize interference with the ability to monitor lower extremity neurologic function and not cause sympathetic blockade that may contribute to postoperative hypotension. For example, bupivacaine 0.05% combined with fentanyl, 2 μg/mL, can be administered via a patient-controlled epidural analgesia infusion pump at a basal rate of 4 to 8 mL/hr initiated after surgery and after the patient exhibits normal neurologic function. Bolus administration of concentrated local anesthetic through the epidural catheter should be discouraged to avoid sympathetic blockade and associated hypotension. The epidural catheter can be inserted before, at the time of surgery, or in the postoperative period. Hemorrhagic complications attributed to epidural catheter insertion or removal have been rare, but care should be exercised to verify that the patient is not being treated with antiplatelet or anticoagulant drugs and that coagulation parameters are satisfactory before insertion and during removal of the epidural catheter.
AORTIC DISSECTION
Aortic dissection is caused by a tear in the intima of the aorta, exposing the underlying diseased medial layer to the pulsatile pressure of the blood within the aortic lumen. Blood exiting the true lumen of the aorta into the medial layer of the vessel through the intimal tear causes the intima to separate or dissect circumferentially and longitudinally within the aorta, creating a true and false lumen contained by the adventitia.18 The aortic dissection may remain localized to an isolated segment of the aorta at the primary entry site at the original intimal tear. Often, the aortic dissection extends distally along the length of the aorta starting at the primary entry site, but it can also propagate proximally or in a retrograde direction. As the dissection propagates along the length of the vessel, the dissection can extend into the aortic branch vessels, cause occlusion of branch vessels resulting in malperfusion syndromes, or the intima may shear at the site of branch vessels creating multiple fenestrations. Propagation of the dissection into the aortic root can cause AR. The development of a false lumen and weakening of the aortic wall caused by the dissection is often accompanied by dilation and expansion in the diameter of the aorta.
Thoracic aortic dissections can be classified according to the location and extent of the aortic dissection. There are two generally accepted classification schemes for thoracic aortic dissections (Table 17-5).
DeBakey Classification |
Type I: Involvement of entire aorta (ascending, arch, and descending) |
Type II: Confined to the ascending aorta |
Type III: Intimal tear originating in the descending aorta with either distal or retrograde extension |
Type IIIA: Intimal tear originating in the descending aorta with extension distally to the diaphragm or proximally into the aortic arch |
Type IIIB: Intimal tear originating in the descending aorta with extension below the diaphragm or proximally into the aortic arch |
Stanford Classification |
Type A: Involvement of the ascending aorta or aortic arch regardless of the site of origin or distal extent |
Type B: Confined to the descending aorta distal to the origin of the left subclavian artery |
Type A Aortic Dissection
Aortic dissections that involve the ascending aorta (Stanford type A) are considered surgical emergencies. From 60% to 70% of patients presenting with aortic dissection will have a Stanford type A dissection. According to an international registry for acute aortic dissection, mortality rates for patients with Stanford type A aortic dissection managed without surgery were estimated to be 1% to 2% per hour after the initial symptom onset and 1% per hour thereafter for the first 48 hours, 60% by day 6, 74% by 2 weeks, and 91% by 6 months.19 When managed with surgery, the mortality rate for type A aortic dissection was 26%. The causes of death and morbidity attributed to type A aortic dissection included rupture of the ascending aorta causing cardiac tamponade, myocardial ischemia or infarction when the dissection involves the coronary ostia, heart failure caused by acute AR, stroke caused by malperfusion of the aortic arch branch vessels, mesenteric malperfusion causing renal failure or ischemic bowel, or limb ischemia.20 Aortic dissection can also rupture into the right atrium, the right ventricle, or the left atrium causing intracardiac shunting with congestive heart failure. An aortic dissection can be considered chronic after 2 weeks, because mortality tends to level off at that time. Late complications of type A aortic dissection include worsening AR, aneurysm formation, or aortic rupture.
SUMMARY
1. Horlocker T.T., Wedel D.J., Benzon H., et al. Regional anesthesia in the anticoagulated patient: Defining the risk. Reg Anesth Pain Med. 2004;29(suppl 1):1.
2. Stratmann G., Russell I.A., Merrick S.H. Use of recombinant factor VIIa as a rescue treatment for intractable bleeding following repeat aortic arch repair. Ann Thorac Surg. 2003;76:2094.
3. Nienaber C.A., Eagle K.A. Aortic dissection: New frontiers in diagnosis and management: II. Therapeutic management and follow-up. Circulation. 2003;108:772.
4. Harrington D.K., Bonser M., Moss A., et al. Neuropsychometric outcome following aortic arch surgery: A prospective randomized trial of retrograde cerebral perfusion. J Thorac Cardiovasc Surg. 2003;126:638.
5. McCullough J.N., Zhang N., Reich D.L., et al. Cerebral metabolic suppression during hypothermic circulatory arrest in humans. Ann Thorac Surg. 1895;67:1999.
6. Pochettino A., Cheung A.T. Pro: Retrograde cerebral perfusion is useful for deep hypothermic circulatory arrest. J Cardiothorac Vasc Anesth. 2003;17:764.
7. Reich D.L., Uysal S. Con: Retrograde cerebral perfusion is not an optimal method of neuroprotection in thoracic aortic surgery. J Cardiothorac Vasc Anesth. 2003;17:768.
8. O’Connor C.J., Rothenberg D.M. Anesthetic considerations for descending thoracic aortic surgery: II. J Cardiothorac Vasc Anesth. 1995;9:734.
9. Cheung A.T., Weiss S.J., McGarvey M.L., et al. Interventions for reversing delayed-onset postoperative paraplegia after thoracic aortic reconstruction. Ann Thorac Surg. 2002;74:413.
10. Estrera A.L., Miller C.C.3rd, Huynh T.T., et al. Neurologic outcome after thoracic and thoracoabdominal aortic aneurysm repair. Ann Thorac Surg. 2001;72:1225.
11. Kouchoukos N.T., Masetti P., Rokkas C.K., et al. Hypothermic cardiopulmonary bypass and circulatory arrest for operations on the descending thoracic and thoracoabdominal aorta. Ann Thorac Surg. 2002;74:S1885.
12. Dietl C.A., Kasirajan K., Pett S.B., et al. Off-pump management of aortic arch aneurysm by using an endovascular thoracic stent graft. J Thorac Cardiovasc Surg. 2003;126:1181.
13. LeMaire S.A., Miller C.C.3rd, Conklin L.D., et al. Estimating group mortality and paraplegia rates after thoracoabdominal aortic aneurysm repair. Ann Thorac Surg. 2003;75:508.
14. Ackerman L.L., Traynelis V.C. Treatment of delayed-onset neurological deficit after aortic surgery with lumbar cerebrospinal fluid drainage. Neurosurgery. 2002;51:1414.
15. Jacobs M.J., Elenbaas T.W., Schurink G.W., et al. Assessment of spinal cord integrity during thoracoabdominal aortic aneurysm repair. Ann Thorac Surg. 1864;74:2002.
16. Motoyoshi N., Takahashi G., Sakurai M., et al. Safety and efficacy of epidural cooling for regional spinal cord hypothermia during thoracoabdominal aneurysm repair. Eur J Cardiothorac Surg. 2004;25:139.
17. Bong C.L., Samuel M., Ng J.M., et al. Effects of preemptive epidural analgesia on post-thoracotomy pain. J Cardiothorac Vasc Anesth. 2006;19:786.
18. Nienaber C.A., Eagle K.A. Aortic dissection: New frontiers in diagnosis and management: I. From etiology to diagnostic strategies. Circulation. 2003;108:628.
19. Hagan P.G., Nienaber C.A., Isselbacher E.M., et al. The international registry of acute aortic dissection (IRAD): New insights into an old disease. JAMA. 2000;283:897.
20. Ehrlich M., Schillinger M., Grabenwoger M., et al. Predictors of outcome and transient neurologic dysfunction following surgical treatment of acute type A dissections. Circulation. 2003;108(suppl II):II.