7 The spinal cord and peripheral nerves
Case 7.1
Questions
• 7.1.1 Describe the neural pathways involved in transmitting this girl’s pain from her leg to her brain.
• 7.1.2 Suggest a list of at least five differential diagnostic possibilities for this girl’s presentation.
• 7.1.3 Describe the presentation of CRPS and discuss the similarities and differences with this case.
Anatomy of the spinal cord
The spinal cord develops as a contiguous structure with the rest of the neuraxis, arising from the ventricular layer of ependymal cells and maintains the basic dorsal and ventral segregation of sensory and motor function as the brainstem (see Chapter 2). The result of this is that most afferent (sensory) information arrives in the dorsal aspects of the cord and the efferent (motor) information exits from the ventral aspects of the cord (see Fig. 2.9A). As the spinal cord matures during embryonic development the dorsal/ventral segregation becomes more defined and by about 3 months post-conception two discrete cellular areas can be determined: the alar lamina, which is located dorsally and contains the neurons that will receive the afferent (sensory) information, and the basal lamina, which is located ventrally and contains the neurons that will supply the efferent (motor) outflow from the spinal cord (Fig. 7.1).
The spinal cord has the following functions
1. Is the final common pathway for the somatomotor system.
2. Conveys somatosensory information from the body to higher centres.
3. Contains preganglionic ANS neurons under segmental/suprasegmental control.
4. Mediates spinal and segmental reflexes.
5. Contains central pattern generators for rhythmic movement gait and posture maintenance.
For the first 3 months of embryonic development the spinal cord and the vertebral column develop at the same pace and are roughly equal in length. During the rest of embryonic development the vertebra column grows in size faster than the spinal cord, resulting in the spinal cord terminating about two-thirds of the way down the vertebral column (Chusid 1982). The length of the spinal cord, which is usually between 42 and 45 cm, can show significant variation between individuals with the end result affecting the level of termination of the spinal cord (Barson 1970). The variation in the termination of the spinal cord can range from the lower third of the twelfth vertebra to the disc between the second and third lumbar vertebra (Jit & Charnalia 1959). The spinal cord terminates by converging into a cylindrical funnel-shaped structure referred to as the conus medullaris, from the distal end of which extends a thin filament, the filum terminale, to its attachment on the first coccygeal segment. The spinal nerve roots radiating from the spinal cord and the dorsal root ganglion neuron’s central projections form a structure referred to as the cauda equina as they traverse the distance, through the spinal canal, between the spinal cord termination point and their exit vertebral foramina in the spinal column (Fig. 7.2).
The volume of the spinal cord is dependent on the number of neurons and axons that it contains at any one point. Because of the increase in afferent input and efferent output that occurs at the level of the cervical and lumbar cord levels, due to the innervation of the arms and legs, the spinal cord expands in circumference, resulting in the cervical and lumbar enlargements.
On cross-sectional views of the spinal cord, the dorsal or posterior median sulcus, which is continuous with a projection of connective tissue that penetrates the posterior aspect of the cord, the dorsal median septum, symmetrically divides the dorsal cord into two halves. Ventrally, the ventral median fissure performs a similar function so that a line connecting it and the dorsal median sulcus effectively divides the spinal cord into left and right symmetrical halves. It is convenient to divide the white matter of the spinal cord into regions referred to as funiculi, so that each half of the spinal cord contains a dorsal or posterior funiculus, a posterior lateral and anterior lateral funiculus, and a ventral or anterior funiculus (Fig. 7.3). In the mature spinal cord the embryonic alar and basal plates, with a few exceptions, maintain their distribution of sensory and motor segregation. These areas can be outlined quite accurately by the funicular divisions just described (Fig. 7.4).
The grey matter of the spinal cord is composed of a high proportion of neurons, neuroglia, and blood vessels
Centrally the butterfly-shaped grey matter of the cord is divided in the midline by the central canal. The grey matter passing dorsally to the central canal is referred to as the posterior grey commissure and the grey matter passing ventrally to the central canal is referred to as the anterior grey commissure. Arising from the area of the ventral lateral sulci are the ventral roots of the spinal cord, which just as they exit the vertebral foramina combine with the afferent axons of the dorsal root ganglion neurons as they enter the vertebral foramina to form the root of the spinal nerve. Entering the spinal cord at the dorsal lateral sulcus are the sensory dorsal roots, completing their journey to the cord from the dorsal root ganglion cells (Fig. 7.5). The areas of grey matter that give rise to or receive the afferent and efferent input resemble the shape of a horn and are thus termed the anterior and posterior horns. The anterior horn does not extend through the anterior funiculus and reach the surface of the cord. The posterior horn projects much more deeply into the dorsal funiculus and except for a small band of translucent neurons, the substantia gelatinosa, it would extend to the posterior surface of the cord. A small angular projection from the intermediate areas of the cord forms the lateral horn of grey matter that only occurs between the levels of the first thoracic to the second lumbar segment. This lateral outgrowth of grey matter houses many of the presynaptic neurons of the sympathetic nervous system.
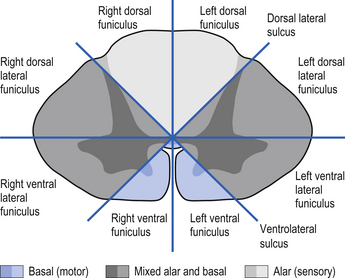
Figure 7.5 The various structures and nerve fibre pathways in a cross-sectional view of the spinal cord.
The neurons of the grey matter form a complex intermingled array involving multiple synaptic connections with many of the axons crossing the midline via the anterior and posterior commissures. Some of the neurons are intrasegmental and their axons and dendrites remain within the same segment of the spinal cord as the neuron soma. Other neurons are intersegmental and their axons and dendrites spread over many segments both rostrally and caudally. In many parts of the neuraxis groups of neurons, usually with a related functional activity, cluster together into nuclei or, when large enough, ganglia. Several nuclei have been identified in the grey matter of the spinal cord. The most predominant neurons in the ventral grey areas are the large multipolar neurons whose axons emerge from the spinal cord to form the anterior horn, and contribute to the spinal nerves, to ultimately innervate the skeletal muscles of the body. These neurons are also referred to as alpha-efferents or alpha motor neurons. Also present in large numbers in the anterior horn are slightly smaller neurons whose axons supply the intrafusal fibres of the muscle spindle called gamma-efferents or gamma motor neurons (Fig. 7.6).
The neuron groupings in the posterior horns involve four main nuclei, two of which extend through the length of the cord and two that are present only at selective levels of the cord. The substantia gelatinosa of Rolando extends throughout the cord and composes the extreme tip of the dorsal horn. These neurons are involved with signal processing of afferent information from the dorsal root ganglion neurons and are thought to play an essential role in the initial processing of pain due to extensive connections with incoming axons destined to form the spinothalamic tracts (Fig. 7.6).
Spinal cord development
• Medulla spinalis extends from the upper border of atlas to the conus medullaris opposite the L1–L2 disc.
• Filum terminale extends to the tip of the coccyx.
• Cord shows cervical and lumbar enlargements.
• In early embryonic development the cord is as long as the vertebral canal but as development proceeds it lags behind the vertebral column.
Internal structure of the spinal cord
• Spinal cord consists of a core of neuropil (grey matter) surrounded by an outer axon fibre layer, the white matter.
• The white matter decreases in proportion as the spinal cord lengthens except at the cervical and lumbar enlargements.
• Grey matter is composed of neuron cell bodies, dendrites, and efferent and afferent axons of the neurons.
A second nuclear group that extends throughout the spinal cord is located ventral to the substantia gelatinosa and is referred to as the dorsal funicular group or the nucleus proprius (Fig. 7.6). Lying ventral to the nucleus proprius in the basal region of the dorsal horn and extending from the eighth cervical region of the cord to the fourth lumbar region of the cord is the nucleus dorsalis or Clark’s nucleus (Fig. 7.6). Finally, a small group of nuclei known as the visceral grey area, or nucleus centrobasalis, is present only in the lower cervical and lumbosacral segments of the cord.
Rexed’s laminae can be used to classify functional aspects of grey matter
In the 1950s and early 1960s an architectural scheme was developed to classify the structure of the spinal cord, based on the cytological features of the neurons in different regions of the grey substance. It consists of nine laminae (I–IX) that extend throughout the cord, roughly paralleling the dorsal and ventral columns of the grey substance, and a tenth region (lamina X) that surrounds the central canal (Rexed 1964) (Fig. 7.8).
A brief description of the functional characteristics of these laminae follows.
Laminae I–IV
These areas are considered the main receiving junctions for primary afferent information. This region is characterised by complex multisynaptic networks of both intra- and intersegmental neurons. Many of the pathways cross the midline of the cord and ascend or descend in contralateral tracts (Fig. 7.9).
Laminae V and VI
Various rexed laminae can be referred to by more than one name
Laminae | Names |
---|---|
I | Lamina marginalis |
Layer of Waldeyer | |
II and III | Substantia gelatinosa |
III and IV | Nucleus proprius |
V | Deiter’s nucleus |
VII | Clark’s column |
Thoracic nucleus | |
X | Substantia gelatinosa centralis |
These areas receive proprioceptive primary afferent information as well as descending collateral input from axons of the corticospinal and reticulospinal tracts. This area is probably very involved in multimodal integration and regulation of movement (Fig. 7.9).
Lamina VII
The medial aspect of this area contains numerous complex networks of connections between propriospinal neurons. This area is probably involved in the integration of the complex propriospinal reflex network of the spinal cord concerned with both movement and autonomic function (Fig. 7.9).
Lamina VIII
This area receives collateral projections from adjacent laminae, medial longitudinal fasciculus, and vestibulospinal and reticulospinal tracts and profuse projections from the contralateral lamina VIII region. Output of this area influences both ipsilateral and contralateral neuron pools through both direct projections to the alpha motor neurons and projections to the gamma motor neuron pools (Fig. 7.9).
The white matter of the spinal cord is composed of axon fibre tracts
Axon fibre tracts of the dorsal funiculus
The dorsal columns are composed of the medially located fasciculus gracilis and the more laterally located fasciculus cuneatus (Figs 7.10, 7.11, 7.12). These pathways transport information from receptors in the periphery about fine and discriminative touch, conscious proprioception, pressure, two-point discrimination, and vibration sense. The primary afferent axons enter the spinal cord grey matter through the dorsal horn and synapse on the neurons in laminae V and VI. The secondary afferents ascend in the ipsilateral dorsal columns. Information from the lower limb and trunk is carried in the gracile funiculus and information from the upper limb and hand by the cuneate funiculus and synapse ipsilaterally in the gracile and cuneate nuclei of the caudal medulla.
Axon fibre tracts of the lateral funiculus
The anterolateral system contains the fibre tracts of the spinothalamic tract and some of the fibres comprising the spinoreticular and spinomesencephalic tracts. These last two pathways provide the afferent limb for neuroendocrine and limbic responses to nociception (Figs 7.11 and 7.13).
The ventral spinocerebellar pathway conveys information about the ongoing status of interneuronal pools in the spinal cord to the cerebellum. It therefore provides continuous monitoring of ascending and descending information concerning locomotion and posture. The neurons of this tract originate in laminae V–VII between L2 and S3. Their projection axons decussate to the other side so that they ascend in the contralateral anterolateral funiculus. These fibres then decussate again via the superior cerebellar peduncle to synapse on neurons in the anterior part of the ipsilateral cerebellum (see Fig. 7.11).
The primary afferent cell bodies are located in the DRG and their central processes synapse with the above-mentioned neurons near the entry level or after ascending for a short distance in the dorsal columns. Secondary afferents ascend in the ipsilateral dorsolateral funiculus, lateral to the corticospinal tracts, and enter the ipsilateral cerebellum via the inferior cerebellar peduncle. Via this pathway, the cerebellum is provided with ongoing information about joint and muscle activity in the trunk and limbs. The cuneocerebellar pathway carries the same type of information from the upper limb and cervical spine via the cuneate fasciculus of the dorsal columns (see Fig. 7.11).
Motor pathways
The anterior and lateral corticospinal tracts are important spinal tracts in the control of volitional movement. The fibre tracts are composed of axons from many different areas of the cortex as well as about 50% of their axons from unidentified areas. These tracts contain about 50% of their axon projections from the large pyramidal neurons of the primary motor cortex and association motor cortex. The lateral corticospinal tract descends in the spinal cord anterolateral to the posterior horn of grey matter and medial to the posterior spinocerebellar tract (Figs 7.11 and 7.14). It contains a large number of motor axon projections from cortical areas 1–4 and 6 to the hands, arms, legs, and feet. Its defining role is to convey motor signals to the ventral horn cells (VHCs) at the lower aspect of the cervical and lumbosacral enlargements of the spinal cord, thus controlling distal limb movements and coordinating distal and proximal muscles to achieve specific trajectories in space. Each corticomotoneuronal cell can achieve these complex goals by synapsing on interneuronal cells that communicate with whole groups of VHCs.
Axons from the projection neurons in the cortex descend in the internal capsule of the cerebrum through the cerebral peduncle of the mesencephalon and continue through the ventral areas of the pons until they enter the pyramids of the medulla oblongata. As the fibres descend in the medulla, about 68% of the fibres cross to form the lateral corticospinal tracts in the lateral funiculus of the contralateral side of the spinal cord; about 30% of the fibres do not cross and form the anterior corticospinal tracts in the ventral funiculus of the ipsilateral side of the spinal cord. The remaining fibres continue as uncrossed fibres of the lateral corticospinal tracts (Fulton & Sheenan 1935).
Most of the axons of both the anterior and lateral corticospinal tracts synapse on interneurons located in laminae IV to VII of the grey matter of the spinal cord (Nyberg-Hansen 1969). Most physiological evidence suggests that the majority of the corticospinal fibres of both tracts act to facilitate flexor groups of muscles and inhibit extensor groups of muscles, which is the opposite effect observed by projections of the vestibulospinal tracts. Injuries involving the corticospinal tracts affect the motor control of the peripheral muscles differently at different levels of the neuraxis. Injuries above the medulla decussation affect the contralateral peripheral muscles. Injuries below the decussation affect the peripheral muscles ipsilateral to the lesion. It must be remembered that not all of the corticospinal fibres cross the midline so that a lesion to a motor cortical area on one side of the corticospinal tract above the decussation will affect the motor control on both sides of the body to a certain extent. This contributes to the understanding of the ipsilateral pyramidal paresis observed when a decreased cortical function (hemisphericity) occurs.
Vestibulospinal tract
The vestibulospinal tracts, lateral and medial, descend in the ventral funiculus, and mediate reflexes (vestibulospinal) that enable an individual to maintain balance and posture despite the effect of gravity and changes in the centre of mass due to movement of the trunk, head, and limbs. The lateral segments, the lateral vestibulospinal tract, descend from the lateral vestibular nucleus uncrossed and exert modulatory effects on the ipsilateral anterior column neurons in the grey matter through the length of the cord. The medial segments of this pathway, the medial vestibulospinal tracts, arise from the medial and inferior vestibular nuclei and descend first in the medial longitudinal fasciculus before entering the vestibulospinal tracts of the cord. This pathway is both crossed and uncrossed and only projects to the cervical and thoracic levels of the cord and as such is probably only involved with upper limb and neck movements (Figs 7.11 and 7.15).
Tectospinal tract
The fibres of this tract originate from the deep layers of the contralateral superior colliculus in the dorsal midbrain (mesencephalon). The tectospinal tract crosses in the dorsal tegmental decussation of the midbrain, which is ventral to the oculomotor nucleus and the medial longitudinal fasciculus (MLF). It maintains a close relationship with the MLF until it reaches the level of the internal arcuate fibres at the decussation of the medial lemniscus. At this point it passes laterally so that it comes to lie in the ventral lateral white matter of the spinal cord. It then descends in the contralateral medial ventral funiculus of the spinal cord, synapsing on interneurons in laminae VI to VIII that communicate with the alpha and gamma motor neurons of the cervical spine (Szentagothai 1948) (Fig. 7.16).
Rubrospinal tract
The rubrospinal pathway has been rumoured to be vestigial in humans because of the evolutionary advancement of the corticospinal pathways; however, its presence in primates probably indicates it will eventually be found in humans as well and an open mind needs to prevail. It originates mainly from the large (magnocellular) neurons of the red nucleus in the rostral mesencephalon, decussates slightly more caudally, and descends just ventrally to the corticospinal fibres in the dorsolateral funiculus of the spinal cord contralaterally (Fig. 7.17).
The rubrospinal tract acts much like the corticospinal tracts in that it affects enhancement flexor tone and inhibition of extensor tone, especially in the proximal limb muscles.
The history of motor function of the spinal nerve
The anterior spinal nerve roots contain only motor fibres and posterior roots only sensory fibres.
• Charles Bell’s work of 1811 contains the first reference to experimental work on the motor functions of the ventral spinal nerve without, however, establishing the sensory functions of the dorsal roots. In 1822 François Magendie definitively discovered that the anterior root is motor and that the dorsal root is sensory.
• Magendie announced that ‘section of the dorsal root abolishes sensation, section of ventral roots abolishes motor activity, and section of both roots abolishes both sensation and motor activity’.
• This discovery has been called ‘the most momentous single discovery in physiology after Harvey’. In the same volume of Journal de physiologie expérimentale et de pathologie, Magendie gave experimental proof of the Bell–Magendie Law.
• Magendie proved Bell’s Law by severing the anterior and posterior roots of spinal nerves in a litter of puppies. Stimulation of the posterior roots caused pain. Magendie sums it up: ‘Charles Bell had had, before me, but unknown to me, the idea of separately cutting the spinal roots; he likewise discovered that the anterior influences muscular contractility more than the posterior does. This is a question of priority in which I have, from the beginning, honored him. Now, as for having established that these roots have distinct properties, distinct functions, that the anterior ones control movement, and the posterior ones sensation, this discovery belongs to me’ (F. Magendie (1847) Comptes rendus hebdomadaires des séances de l’Académie des sciences, 24: 3).
Reticulospinal tract
The reticulospinal pathways can be divided into the medial or pontoreticular and lateral or medulloreticular spinal tracts. The pontoreticular neuron projections comprise the medial reticulospinal pathways and are predominately ipsilateral. They project to interneurons of laminae VII and VIII where they act to excite VHCs on the same side of origin. Some fibres do cross one or two spinal segments above their target destinations but the main modulating effects remain ipsilateral to the neurons of origin. The lateral reticulospinal pathways arise from the neurons in the medullary areas of the reticular formation and in particular from the nucleus reticularis gigantocellularis region. The projections have been found in a variety of fibre tracts in the white matter of the cord, but for the most part travel medial to the corticospinal tracts with a small tract occasionally travelling lateral to the corticospinal tracts in the lateral funiculus. In contrast with the medial reticulospinal tracts, the projections of the lateral tract are largely crossed with some ipsilateral representation (see Fig. 7.16). Projections from each half of the medullary reticular formation exert an inhibitory effect on spinal cord neurons bilaterally, probably through the activities of inhibitory interneurons (Renshaw cells) of lamina VII of the spinal cord. These projections also modulate the effects of afferent impulses arriving in these areas of the cord (Nyberg-Hansen 1965). The loss of inhibitory projections to the spinal cord from the cortex has been thought to play an important role in spasticity observed in lesions of the cord, brainstem, or cortex. However, extrapyramidal, reticulospinal inhibitory dysfunction is also thought to be an important contributing factor. The differential activation of VHC groups by reticulospinal projections (e.g. locomotor and inhibitory systems) in combination with the effects of lesions of the corticospinal projections as previously discussed leads to a characteristic weakness or ‘soft’ weakness pattern in the limbs in response to spinal cord lesions, brain damage, or hemisphericity.
Interstitiospinal tract
The fibres of this tract arise from the interstitial nucleus and descend in the medial longitudinal fasciculus. They extend into the spinal cord from the MLF into the ipsilateral fasciculus proprius, which terminates on a network of interneurons located in the dorsal horn. These interneurons are thought to participate in intersegmental coordination of various muscles.
Spinal cord reflexes
Local spinal cord reflex circuits are also important in volitional movement in that descending motor pathways converge on interneurons to allow complex movement patterns to occur – i.e. corticomotoneuronal cells of the brain alter the trajectory of a limb in space by activating these reflex circuits involving agonist, antagonist, synergist, and neighbouring joint muscle groups. Feedback and feedforward mechanisms are employed by the cerebellum to assist plastic changes in the brain and spinal cord. Some stereotypical reflexes mediated by the spinal cord are state- or phase-dependent. For example, activation of Golgi tendon organs (GTOs) in the soleus and gastrocnemius muscles will trigger a different set of interneurons in the spinal cord, depending on whether the individual is in a state of locomotion or is non-ambulatory, and whether the individual is in the swing or stance phase of gait (Fig. 7.18).
Laminar organisation in the spinal cord is not complete or as accurate as previously thought
Laminar organisation in the spinal cord is not as complete or as accurate as previously thought. The traditional understanding of the laminar distribution of pathways in the white matter of the spinal cord was that the projections to and from the most distal areas of the body were more lateral in the spinal cord except in the dorsal columns where the reverse occurs. Some variability of these laminar patterns has been demonstrated; however, the general pattern in dorsal column, spinothalamic, and corticospinal tracts is important to understand from a clinical perspective.
The spinal nerves
The spinal nerves represent the neural division of the embryological somite and contain motor, sensory, and autonomic components. The spinal nerves separate into dorsal and ventral rami as they exit the vertebral foramina (Fig. 7.21). The somatic component of the spinal nerve contains the motor nerves to skeletal muscle and the afferent information from a variety of receptors. The visceral component contains the afferent and efferent fibres of the autonomic nervous system.
The dorsal and ventral rami of the spinal nerves than continue to separate into smaller and smaller peripheral nerves, all of which contain both afferent and efferent fibres of the somatic and visceral components. The anatomy of the visceral or autonomic division is discussed in Chapter 8. The functional distribution of the muscular and sensory divisions, including dermatomes and motor actions of peripheral nerves, has been discussed in Chapter 4.
Peripheral nerve fibre classification
Erlanger and Gasser (1937) divided peripheral nerve fibres based on velocity of conduction. These are the three peaks seen on a compound nerve conduction velocity study and can be classified as follows.
Afferent type A fibres include:
Aα—cutaneous, joint, muscle spindle, and large alimentary enteroreceptors;
Aβ—Merkel discs, pacinian corpuscles, Meissner corpuscles, Ruffini endings; and
Aγ—thermoreceptors and nociceptors in dental pulp, skin, and connective tissue.
Lloyd’s classification is based on fibre diameters ranging from 22 to 1.5 μm for myelinated fibres and 2–0.1 μm for non-myelinated fibres. Only afferent fibres were classified, and these were arranged into four groups. Myelinated fibres are divided into group I, II, and III, and non-myelinated fibres compose group IV (Table 7.1). Group or type I fibres, which have diameters ranging from 12 to 22 μm, are further divided into groups Ia and Ib. Group Ia fibres are larger, are heavily myelinated, and transmit information from muscle spindles and joint mechanoreceptors. Group Ib fibres are smaller, are moderately myelinated, and transfer information from Golgi tendon organs, and some joint mechanoreceptors.
1. They have the greatest nerve conduction velocity.
2. They are the most sensitive to hypoxia.
3. They are the most sensitive to compression.
4. They are the most sensitive to thermal changes.
5. They are the most sensitive to bacteraemia and viral toxaemia.
The nociceptive C fibres are the most sensitive to chemicals such as anaesthetic agents.
Compression of nerves results in retrograde chromatolysis and transneural degeneration
Compression of a peripheral nerve affects the largest nerve fibres first: thus the Ia afferents and the alpha motor neurons. Compression, therefore, produces both sensory and motor losses because they both involve large axon types in proportion to the number of axons damaged. In a compression axonopathy, one cannot exist without the other, which is of diagnostic value. This concept can be extrapolated to all nerve fibres of a specific diameter under compression. For example, if a patient perceives pain, the type C nociceptive fibres are intact. This knowledge can be extrapolated to also mean that the type C autonomic fibres must also be intact.
Central cord syndrome (syringomyelia)
• It is a disease of the spinal cord.
• It is associated with gliosis and cavitation of the spinal cord.
• Lower cervical roots are most commonly affected but lumbar and brainstem may also be involved.
• It is thought to occur because of inappropriate nest formation of glial cells in the central portion of the cord.
• Swelling of the cell due to failure of ionic pumps and loss of internal negativity, both allowing an influx of hydrated sodium;
• Eccentricity of the nucleus due to decreased tubulin, the supporting intracellular protein;
• Decreased rough endoplasmic reticulum and Nissl substance due to decrease of protein replication; and
Wallerian degeneration occurs in six stages
1. Transection or transection-like event occurs to the axon, which results in a decreased axoplasmic flow and cessation of nutrient supply to the distal axon.
2. Dissolution of axon occurs within 2 days of the transection and breakdown of the axon into clumps within the myelin sheath starts to occur.
3. Secondary demyelination starts to occur and the myelin sheath starts to disintegrate at various points along its length referred to as ‘Schmidt– Lantermann clefts’ because of axon degeneration.
4. Resorption of the axon remains starts to occur via Schwann cell auto-phagocytosis of myelin/axon debris. The debris is phagocytosed and digested by lysosomal activity into neutral lipid and transferred to macrophages for further degradation and removal. This process may take up to 3 months for completion.
5. Schwann cell proliferation starts to occur with the myelin debris acting as a mitogen. The rapidly developing Schwann cells form a column of cells called the ‘bands of Bungner’.
6. Axonal regeneration will occur under the appropriate environmental conditions. If axonal regeneration fails, then endoneural fibrosis starts to occur, which results in atrophy of the Schwann cells and fibrosis of the endoneurium.
The process of axonal regeneration occurs in three stages
1. The reactive stage involves the formation of a proximal axon stump. The formation of a proximal axon stump requires sealing of the axon stump, swelling of proximal stump, and the demyelination of one proximal internodal segment. Neuronal cell body undergoes central chromatolysis, which involves swelling of the cell due to failure of the sodium/potassium ionic pumps. This results in an increase in sodium concentration on the inside of the cell, which attracts water molecules by osmosis and swells the neuron. Eccentricity of nucleus occurs because of a decrease in the proteins necessary to manufacture microtubules and microfilaments, secondary to reduced rough endoplasmic reticulum volume, which maintains the shape and structure of the neuron including the central location of the nucleus. A reduction in both mitochondrial production levels and population also occurs.
2. The regenerative phase involves the regrowth of the distal axon. Axon regrowth usually occurs via terminal or collateral sprouting of the axon.
3. The remyelination phase starts to occur when the newly forming axon reaches 2 μm in diameter; then the axon starts to attract Schwann cells and the first myelin starts to form in the region of the bands of Bungner. The new axon sheath is thinner and has shorter internodal spaces than the original axon.
Fibrillations and fasciculations on electromyography (EMG)
• Fibrillations are spontaneous motor fibre contractions detectable via EMG because of muscle fibre irritability.
• Fasciculations are spontaneous quivering movements visible to the naked eye because of spontaneous motor unit firing.
• Muscle disease often results in the production of small polyphasic units on EMG.
Primary demyelination can occur via two main mechanisms
1. The direct destruction of myelin occurs in diseases such as Guillain–Barré polyneuropathy (GBP) —The hallmark of GBP is an autoimmune attack by sensitised macrophages on the myelin sheath with sparing of the Schwann cells.
2. The metabolic impairment of the Schwann cell—An example of this type of metabolic dysfunction can be seen with the exposure to diphtheria toxin. This toxin, which is manufactured by the bacterium Corynebacterium diphtheriae, acts to poison the respiratory mechanisms of the mitochondria, resulting in the inhibition of protein synthesis in Schwann cells, leading to segmental demyelination.
Classification of nerve injuries
For classifying neurotmesis, it may be better to use the Sunderland system. In Sunderland’s classification, peripheral nerve injuries are arranged in ascending order of severity. In first-degree injury conduction along the axon is physiologically interrupted at the site of injury, but the axon is not actually disrupted (neuropraxia). In second-degree injury axonal disruption is present but the integrity of the endoneural tube is maintained (axonotmesis). Further degrees of injuries (third, fourth, fifth) are based on the increasing degrees of anatomic disruption of the fibres with or without rupture of the ensheathing membrane, until the final fifth-degree injury where total anatomical rupture of the whole nerve occurs (neurotmesis).
Diagnosis of nerve lesions
A complete diagnosis of a traumatic nerve lesion should include identification of the following:
Clinical examination of nerve injuries
1. Motor signs—Note any muscles paralysed distal to the lesion and the wasting of muscles. The muscle actions should be graded using the following scale:
2. Sensory signs—Sensory signs should be noted under both subjective and objective criteria. Subjective criteria can be obtained by asking the patient to describe the distribution of pain, tingling, or burning sensations and noting the responses. Objective criteria can be obtained by utilising clinical tests to evoke a response from the patient such as blunting or loss of sensation to pinprick, cotton wool touch, and temperature.
3. Sudomotor signs—Sudomotor signs include involuntary responses to stimuli such as blushing. Anhidrosis can be detected by the area of dry skin it causes due to absence of sweating.
4. Vasomotor signs—Vasomotor signs such as cold or warm hands and feet can be used to gauge autonomic tone.
5. Trophic changes—Trophic changes can be detected by examining the skin for smoothness and shiny areas, ulceration, and subcutaneous tissue atrophy.
6. Reflexes—Loss of tendon reflexes can indicate afferent or efferent nerve damage or motor unit damage.
7. Recovery signs—Look and test for signs of recovery. The presence of Tinel’s sign may indicate both damage and recovery in a nerve pathway.
The perception of pain
Pain is a multidimensional phenomenon dependent on the complex interaction of several areas of the neuraxis. The link between pain and injury seems so obvious that it is widely believed that pain is always the result of physical damage, and that the intensity of pain felt is proportional to the severity of the injury. For the most part, this relationship between pain and injury holds true in that a mild injury produces a mild pain, and a large injury produces great pain. However, there are many situations where this relationship fails to hold up. For example, some people are born without the ability to feel pain even when they are seriously injured. This condition is referred to as congenital analgesia. There are also people who experience severe pains not associated with any known tissue damage or that persist for years after injuries have apparently healed (Melzack & Wall 1996).
Injury without pain
Congenital analgesia
People who are born without the ability to feel pain often sustain extensive burns, bruises, and lacerations during childhood. They frequently bite deeply into their tongues during chewing and learn only with great difficulty to avoid inflicting severe wounds on themselves. Usually, these people show severe pathological changes in the weight-bearing joints, especially of the hips, knees, and spine, which are attributed to the lack of protection to joints usually given by the sensation of pain. The condition of a joint that degenerates because of failure to feel pain is called the ‘Charcot’ or neurotrophic joint. It has long been known that if the nerves that normally innervate a joint are missing or defective, a condition in which the joint surfaces become damaged and the ligaments and other tissues become stretched and unstable develops. In many cases of congenital analgesia the cause remains a mystery (Melzack & Wall 1996). Histological and physical examination of the nerves and nerve activities surrounding this loss of pain show no abnormal nerve activity or abnormal concentrations of cerebrospinal endorphins.
Episodic analgesia
Cases of congenital analgesia are rare. Much more common is the condition most have experienced at one time or another, that of sustaining an injury, but not feeling pain until many minutes or hours afterwards. Injuries may range from minor cuts and bruises to severe broken bones and even the loss of a limb. Soldiers in the heat of battle frequently described situations in which an injury has not produced pain. In studies performed on these injured soldiers, it was found that they were not in a state of shock nor were they totally unable to feel any pain, for they complained as vigorously as a normal man at an inept nurse performing vein punctures. Their lack of ability to feel pain was attributed to their sense of relief or euphoria at having escaped alive from the field of battle (Melzack & Wall 1996).
There are six important characteristics of episodic pain:
1. The condition has no relation to the severity or location of injury. It may occur with small skin cuts on an arm or leg or with the arm or leg blown off by explosives.
2. It has no simple relationship to the circumstances. It may occur in the heat of battle, or when a carpenter cuts off the tip of his finger, trying to make an accurate cut.
3. The victim can be fully aware of the nature of the injury and of its consequences and still feel no pain.
4. The analgesia is instantaneous. The victim does not first feel pain and then bring it under control. These people are not confused, distracted, or in shock. They understand the extent of their injury and may even touch the injured area, and still do not feel pain.
5. The analgesia has a limited time course: usually by the next day all these people are in pain.
6. The analgesia is localised to the injury. People may complain about other more minor injuries at other locations on the body.
Pain without injury
The mechanism of pain without cause is thought to occur through central pain mechanisms. In central pain, an arm or a leg that apparently has nothing wrong with it can hurt so much or feel so strange that patients struggle to describe the pain or the feelings that they perceive (Boivie 2005). Central pain syndrome is a neurological condition caused by damage to or dysfunction of the central nervous system (CNS), which includes the brain, thalamus, brainstem, and spinal cord. The thalamus, in particular, has been implicated as a causative lesion site in as high as 70% of cases presenting with central pain (Bowsher et al. 1998). The characteristics of central pain include steady burning, cold, pins and needles, and lacerating or aching pain although no one characteristic is pathognomonic (Bowsher 1996). Central pain can be associated with breakthrough pain and decreased discriminative sensation. Onset can be delayed, particularly after stroke. There are considerable differences in the prevalence of central pain among the various disorders associated with it. The highest incidence of central pain occurs in multiple sclerosis (MS), stroke, syringomyelia, tumour, epilepsy, brain or spinal cord trauma, and Parkinson’s disease (Boivie 1999; Siddall et al. 2003; Osterberg et al. 2005).
1. At what level of the neuraxis has the damage occurred?—This requires a full neurological exam as outlined in Chapter 4.
2. Is the damage reversible?—Evaluating the response of effectors to stimulation aimed at the relevant areas of the neuraxis can give an indication as to whether the lesion can be reversed.
3. At what level of the neuraxis has central sensitisation or reorganisation occurred? —A careful analysis of the results of the neurological exam will establish the level of the lesion in the neuraxis.
4. What options are available to influence these processes?—Several approaches are available for treatment alternatives (see Chapter 17).
Pain disproportionate to the severity of injury
The kidney may, under certain conditions, concentrate some components in the urine so that these compounds precipitate out of the urine and form small kidney stones or renal calculi. Small pieces of the stones break off and pass into the ureter that leads from the kidney to the bladder. In size, they are not more than twice the size of the normal diameter of the normal ureter. Pressure builds up behind the plug formed by the stone, tending to drive it into the ureter and, as a result, the muscle in the wall of the ureter goes into localised strong contraction. This band of contraction moves down the ureter to produce peristaltic waves to drive the stone down. During this process called ‘passing a stone’ agonising spasms of pain sweep over the patient in such a way that even the toughest and most stoical of characters usually collapse. The patient is pale with a racing pulse, knees drawn up, with a rigid abdomen and motionless. Even crying out because of the pain is restrained because all movement exaggerates the pain. As the stone passes into the bladder there is immediate and complete relief of the pain resulting in an exhausted patient. The reason for describing this event here is that in physiological terms, and mechanical terms, this is a rather trivial event. Furthermore, it occurs in a structure which is poorly innervated when compared to other areas of the body. This process of passing kidney stones is described by the patient as painful beyond any expectation that pain can reach such intensity (Melzack & Wall 1996).
Hyperalgesia is the term used to describe an excessive response to noxious stimulation. Hyperalgesia can be classified as either primary or secondary in nature. Primary hyperalgesia results from the release of various chemicals at the site of injury, leading to sensitisation of nociceptive afferents. Secondary hyperalgesia involves collateral branches of the nociceptive afferents at the level of the spinal cord, which results in the regions surrounding the site of injury becoming more sensitive to pain.
Pain after healing of an injury
Occasionally, people who have experienced amputations of limbs may feel the presence of that limb even though the limb has been amputated. This is known as phantom pain (Melzack & Wall 1996). This shows that in certain cases pain may persist long after all apparent physical healing has occurred.
The term phantom limb was introduced by Silas Weir Mitchell. It is used to describe malrepresentation of actual limb position or existence following amputation or nerve blocks and is recognised by the patient as an ‘illusion’ rather than being the patient’s delusion (Ramachandran & Hirstein 1998). Phantoms occur in 90–98% of all amputees almost immediately, but less commonly in children.
The reduction in activity experienced by cortical neurons as a result of sensory deprivation reduces the amounts of the inhibitory neurotransmitter γ-aminobutyric acid (GABA) released from interneurons, which in turn may allow previously weak synapses to become disinhibited. These normally suppressed inputs probably originate from long-range horizontal collaterals of pyramidal neurons located in cortex adjacent to the area of cortex that has lost its afferent stimulus due to the removal of the limb (Ramachandran & Hirstein 1998). Conversely, high levels of GABA brought about by persistent intense sensory input can cause weak synapses to become even more strongly inhibited, resulting in a surround inhibition of cortical areas not of immediate concern. In other words, the extensive use of the fingers of the left hand, as would be the case in learning to play the guitar, will ‘focus’ the cortical area representing the fingers of the left hand and inhibit adjacent areas of cortex representing the elbow and shoulder.
Tinnitus, which is the subjective sensation of noise in the ears, has also been referred to as a phantom auditory sensation that may occur due to reorganisation of the auditory cortex following some degree of deafferentation from the cochlea of the inner ear. Cochlear lesions resulting in loss of a specific range of frequencies can lead to reorganisation of the auditory cortex due to replacement of the corresponding cortical areas with neighbouring areas of sound representation (Sexton 2006).
The anatomy of pain
Several pathways that originate from neurons in the spinal cord and project to higher centres in the neuraxis have nociceptive components (Willis & Coggeshall 2004; Willis & Westlund 2004). These pathways include the following:
1. Spinothalamic tract (STT) receives axons from neurons in laminae I and V–VII of the contralateral cord and project to the thalamus ipsilateral to the tract (Fig. 7.23). This tract has traditionally been recognised as the most important tract for the transmission of nociceptive information. The STT is thought to contribute to motivational and affective aspects of pain as well (Fig. 7.24). The axons of neurons in lamina I terminate on a number of nuclei in the thalamus including the ventroposterior lateral (VPL) nucleus, the ventral posterior inferior (VPI) nucleus, and the central lateral nucleus in the medial thalamus (Zhang et al. 2000).
2. Spinoreticular tract receives axons from neurons in laminae VII and VIII. The tracts ascend bilaterally in the anterolateral system (Figs 7.25 and 7.26).
3. Spinomesencephalic tracts receive axons from neurons in laminae I and V and ascend in the anterolateral system bilaterally to synapse in the mesencephalic reticular formation and periaqueductal grey areas (Figs 7.25 and 7.26).
4. Spinohypothalamic tracts receive axons from neurons in laminae I, V, and VIII, and project to supraspinal autonomic centres responsible for complex neuroendocrine and cardiovascular responses.
5. Postsynaptic dorsal column (PSDC) receives the majority of its axons from neurons in laminae III and IV but does receives additional axons from lamina X as well (Al-Chaer et al. 1996; Willis & Coggeshall 2004). The projections from the PSDC first synapse on neurons in the dorsal column nuclei. Axons from the dorsal column nuclei cells project to the contralateral thalamus via the medial lemniscal tracts and to the brainstem (Wang et al. 1999).
6. Spinocervical tracts receive axons from neurons in laminae III and IV and project to synapse on neurons of the lateral cervical nucleus.
7. Spinoparabrachial tract is a component of the spinomesencephalic tract that projects to the parabrachial nuclei and amygdala. This contributes to the affective component of pain.
Descending control of spinal projection neurons are mediated through pathways that descend from supraspinal areas into the spinal cord. Inhibition of STT in the spinal cord occurs through projections from the para-aqueductal grey (PAG), nucleus raphe magnus, medullary reticular formation, anterior pretectal nucleus, ventrobasal thalamus, and postcentral gyrus. Excitation of the STT neurons can occur through stimulus from the motor cortex and isolated areas of the medullary reticular formation (Figs 7.25 and 7.26).
Pain…: Good or evil?
From our above discussions, it seems that pain can serve three purposes:
1. Pain can occur before a serious injury, as when one steps on a hot or otherwise potentially damaging object. This has a real survival value. It produces immediate withdrawal or some other action that prevents further injury.
2. Pain can also prevent further injury and act as the basis for learning to avoid injurious objects or situations, which may occur at a later time.
3. Pain due to damaged joints, abdominal infections or diseases, or serious injuries may also set limits on activity and enforce rest, which are often essential for the body’s natural recuperative and disease-fighting mechanisms to work.
However, one also perceives pains that serve no useful survival value, such as the phantom pain described earlier. It is also commonly observed that very severe disease processes may develop to a very extensive state before pain is experienced by the individual (Melzack & Wall 1996). Why in this case did our sensation of pain fail us?
The psychology of pain
1. Previous experiences and how well they are remembered;
2. One’s ability to understand the cause of pain;
3. One’s ability to grasp its consequences; and
4. Even the culture in which one is brought up plays an essential role in how one feels and responds to pain.
The above facts lead to the conclusion that the perception of pain cannot be defined simply in terms of a particular kind of stimuli; rather, the perception of pain is a highly personal experience depending on cultural learning, the meaning of the situation, and other factors unique to each individual in any given situation. There are a variety of stressors known to affect the perception of pain (Melzack & Wall 1996). These include ethnic/cultural values, age, environment, support systems, anxiety, and stress.
A number of recent studies have implicated the cingulate gyrus as the functional link between pain and emotional interactions (Rainville et al. 1997; Sawamoto et al. 2000). It is now known that the cingulate gyrus participates in pain and emotion processing. It has four regions, with associated subregions, and each makes a qualitatively unique contribution to brain functions. These regions and subregions are the subgenual (sACC) and pregenual (pACC) anterior cingulate cortex, the anterior midcingulate (aMCC) and posterior midcingulate cortex (pMCC), the dorsal posterior (dPCC) and ventral posterior cingulate cortex (vPCC), and the retrosplenial cortex (RSC) (Vogt et al. 2006).
1. The pACC is involved in unpleasant experiences and directly drives autonomic outputs.
2. The aMCC is involved in fear, prediction of negative consequences, and avoidance behaviours through the rostral cingulate motor area.
3. The pMCC and dPCC are not involved in emotion but are driven by short-latency somatosensory signals that mediate orientation of the body in space through the caudal cingulate motor area.
Pain thresholds
It is often believed that variations in pain experienced for person-to-person is due to different pain thresholds. There are four different thresholds related to pain, and it is important to distinguish between them.
1. Sensation threshold—this is the lowest stimulus value at which a sensation, such as tingling or heat, is first reported by the subject;
2. Pain perception threshold—this is the lowest stimulus value at which the person reports that the stimulation feels painful;
3. Pain tolerance—this is the lowest stimulus level at which the subject withdraws or asks to have the stimulation stopped; and
4. Encouraged pain tolerance—this is the same as the above, but the person is encouraged to tolerate higher levels of stimulation.
The importance of the meaning associated with the pain-producing situation is made particularly clear in experiments carried out by Pavlov on dogs. Dogs normally react violently when they are exposed to electric shocks to one of their paws. Pavlov found, however, that when he consistently presented food to a dog after each shock the dog developed an entirely new response. Immediately after each shock the dog would salivate, wag its tail, and turn eagerly towards the food dish. The electric shock now fails to evoke any responses indicative of pain and has become a signal meaning that food was on the way. This type of conditioned behaviour was observed as long as the same paw was shocked. If the shocks were applied to another paw the dog reacted violently. This study shows very convincingly that stimulation of the skin is localised, identified, and evaluated before it produces perceptual experience and overt behaviour (Melzack & Wall 1996). The meaning of the stimulus acquired during earlier conditioning modulates the sensory input before it activates brain processes that underlie perception and response.
Pain can be caused by nociceptive or neuropathic mechanisms
Inflammatory pain is related to tissue damage. Damage to neurons can result in the release of neurotransmitters, and neuropeptides that can result in neurogenic inflammation. The proinflammatory substance prostaglandin E2 (PGE2) is released from damaged neurons and other cells. PGE2 is a metabolite of arachidonic acid via the cyclo-oxygenase pathway. The cyclo-oxygenase enzyme can be blocked by the use of nonsteroidal anti-inflammatory drugs (NSAIDs) and aspirin and is thought to be the mechanism by which these medications exert their effect. Bradykinin, which is also an extremely active proinflammatory and pain-activating substance, is also released when tissue is damaged. Bradykinin activates Ad and C fibres directly and causes synthesis and release of prostaglandins from nearby cells.
Descriptions of pain
Chronic pain
Placebo effect
The gold standard in best practice therapeutics is that the treatment should significantly outperform the placebo effect in order to be considered a viable option for therapy. In the treatment of pain, production of analgesia or loss of pain sensation is the desired effect (Zhuo 2005).
As mentioned above, endogenous analgesia systems are a likely component of the placebo effect. The anterior cingulate gyrus (ACC) has been found to be involved in this placebo analgesia. It has been theorised that ACC activation is responsible for facilitating descending inhibitory systems. However, electrical stimulation or glutamate synaptic activation in the ACC has actually been observed to increase nociceptive reflexes at the level of the spinal cord, and enhanced synaptic transmission and long-term plasticity have been found in ACC neurons after tissue injury, which suggests that the ACC may actually enhance any existing nociceptive effects. This would act in the opposite of the placebo effect and actually increase pain! Several theories have been advanced to incorporate these findings into a model that still allows the involvement of the ACC as a component in the generation of the placebo effect (Zhuo 2005).
The first theory involves the inhibition of pain-producing neurons in the ACC. Many neurons in the ACC respond to acute pain and the amount of this activation is related to pain unpleasantness. Activation of inhibitory neuron in the ACC can affect the excitability of these neurons by releasing GABA onto their postsynaptic receptors. Consequently, the excitability of ACC neurons is reduced, and neurons respond less to noxious stimuli.
Complex regional pain syndromes
• Reflex sympathetic dystrophy syndrome (RSD/RSDS);
• Sympathetically maintained pain;
• Sympathetically independent pain;
CRPS I—Consists of pain, sensory abnormalities, abnormal sweating and blood flow, abnormal motor system function and trophic changes (thickening of the skin and nails, coarse thin hair growth), and atrophy of the superficial and deep tissues (skin, muscle, bone). The most common form is RSD and may not present with an identifiable nerve injury.
CRPS II—Same as CRPS I but presents with an identifiable nerve injury. Symptoms include burning pain made worse by light touch, temperature changes, or motion of the limb. These findings are most common in the foot or hand following partial injury to the nerve. The affected area appears cool, reddish, and clammy. The superficial and deep tissue structures may also begin trophic changes.
In some cases the sympathetic nervous system has been implicated as an important component in sustaining the pain. These abnormal changes in the sympathetic nervous system seem to be responsible in some patients for constant pain signals to the brain, which alters the cortical areas of their brain responsible for pain and sensory reception of those areas of the body. Abnormal function of the sympathetic nervous system can also lead to movement disorders. Recent evidence, however, does not support that the pain of CRPS is solely sympathetically mediated; therefore, a thorough investigation examining the central integrated state of all levels of the neuraxis should be undertaken to determine the true nature of the patient’s persistent or severe pain syndrome. An updated theory concerning the mechanism behind CRPS is that it is caused by supersensitivity of sympathetic nerve neurotransmitters and their metabolites (Rowbotham et al. 2006). Patients with CRPS have been found to have decreased concentrations of norepinephrine in the venous effluent of the affected limb. This suggests that it is not due to increased output of the sympathetic nervous system. CRPS patients have increased concentrations of bradykinin and other local non-specific inflammatory mediators. Sympathetic inhibition may lead to up-regulation of beta-adrenergic receptors on the peripheral nociceptive fibres, making the afferents more sensitive to normal or lower levels of the neurotransmitter. It is more common to observe an initial increase in skin temperature followed by a chronically decreased skin temperature and trophic changes later in the course of the condition. The generation and maintenance of central sensitisation are dependent on the actions of transmitter/receptor systems in the peripheral cord. Activation of receptor systems and second messenger systems leads to changes in receptor sensitivity, which increases the excitability of neurons (Schaible et al. 2002). This is a form of physiological wind-up.
This process can be summarised into six steps:
1. Sensitisation of C-nociceptors after initial pain-evoked sympathetic reflex vasoconstriction, which results in the activation of glutamatinergic N-methyl-D-aspartate (NMDA) receptors that cause increased Ca++ ion flux into the neurons which activated second messengers and increase sensitivity of the neuron to stimulus (Neugebauer et al. 1993);
2. Nociceptors up-regulate expression of alpha-1 adrenoreceptors, which increases the action of catecholamines on the neuron;
3. Activation by tonic release of norepinephrine by sympathetic efferents, which bind to the increased receptor population and magnify the response;
4. Receptive field changes of central projecting pain neurons, resulting in increased pools of neuron activation and inappropriate spread of the initial stimulus;
5. Activity-dependent neuronal plasticity, allowing the system to function over long periods (Schaible & Grubb 1993); and
6. Central sensitisation, resulting in wind-up of pain neurons.
Paediatric patients present unique challenges. For example, children have not had sufficient time to develop the psychosocial skills necessary to cope with the pain and suffering due to CRPS. The fear and anxiety that this syndrome produces in a child leads to a further lowering in the child’s pain threshold, making activities of normal life even more painful. The presence of these seemingly unexplainable symptoms has led to a great deal of confusion and frustration among children and their families. Another theory is that CRPS is caused by a triggering of the immune response, which leads to the characteristic inflammatory symptoms of redness, warmth, and swelling in the affected area (Romanelli & Esposito 2004).
General features of CRPS
Motor disorders
• Sense of weakness with complex motor tasks (79%): ‘Give-way’;
• Difficulty initiating movements;
• Limited range of motion: wrist; ankle;
• More common with nerve lesions;
• Irregular myoclonic jerks, dystonia, or muscle spasm (30%); and
Case 7.1
7.1.3
The presentation CRPS includes the following signs and symptoms which usually occur following an injury of some sort to a peripheral limb:
General features
Case 7.2
7.2.1
Muscle spindle receptors in the muscles and the joint receptors in the joints relay information to the spinal cord concerning movement and proprioception. The ventral spinocerebellar pathway conveys information about the ongoing status of interneuronal pools in the spinal cord to the cerebellum. It therefore provides continuous monitoring of ascending and descending information concerning locomotion and posture. The neurons of this tract originate in laminae V–VII between L2 and S3. Their projection axons decussate to the other side so that they ascend in the contralateral anterolateral funiculus. These fibres then decussate again via the superior cerebellar peduncle to synapse on neurons in the anterior part of the ipsilateral cerebellum (Fig. 7.12).
References
Melzack R., Wall P.D. The Challenge of Pain. New York: Penguin, 1996.
Neugebauer V., Lucke T., Schaible H.G. N-Methyl-D. J. Neurophysiol.. 1993;70:1365-1377.
Schaible H.G., Grubb B.D. Afferent and spinal mechanisms of joint pain. Pain. 1993;55:5-54.
Zhuo M. Central inhibition and placebo analgesia. Mol. Pain. 2005;1:21.