Figure 1.1 Proximal femur from an older child demonstrating the cortex, medullary cavity, growth plates, apophysis, and articular surface.
Tan-white and smooth-surfaced, bones are the hardest and strongest structures of the body, being as strong as cast iron but one-third of the weight as a result of their adaptive architecture. Comparatively lightweight, rigid but not brittle, reinforced, generally asymmetric, and hollow, bones are designed to have a relatively high tensile strength, and maximum strength-to-weight ratio. These characteristics are derived from the substance of bones – all are composed of bone tissue – a specialized type of connective tissue that is a unique biphasic blend of inorganic or mineral component – calcium hydroxyapatite – and organic constituents – the cells and the proteins they synthesize.
Bones vary greatly in size and shape and these features form the basis of the classification of individual bones. The most prominent group of bones are tubular, both long and short (Fig. 1.1), and the other types include flat (bilaminar plates) and cuboid bones. Anatomically, tubular bones are further subdivided into the epiphysis, the metaphysis, and the diaphysis (Fig. 1.2) (2). The epiphysis extends from the base of the articular surface to the beginning point of significant narrowing of the bone. The metaphysis embodies the region of bone that displays a prominent reduction in diameter, and the diaphysis or shaft extends from the base of one metaphysis (the point where decrease in bone diameter ceases) to the base of the opposing metaphysis. During growth and development the metaphysis is composed of the cartilaginous growth plate also known as the physis and the adjacent primary and secondary spongiosa and the surrounding cortex. The medical and forensic determination of skeletal age and the prediction of ultimate size utilize the degree of maturation of the physes, the amount and localization of bone ossification, the formation and dimensions of the secondary ossification centers, and the degree and amount of remodeling (see below).
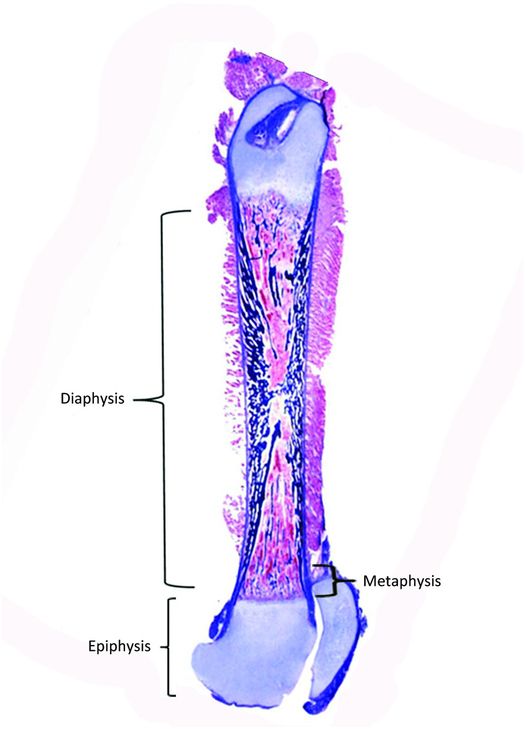
Figure 1.2 Femur from a fetus showing the epiphysis, metaphysis, and diaphysis.
Bones are covered externally by a periosteum. The periosteum is anchored to the cortex, which in turn houses the medullary canal that contains variable amounts of cancellous or trabecular bone, fatty and hematopoietic marrow, blood vessels, and nerves. The quantity and arrangement of cortical and cancellous bone is directly related to the biomechanical requirements of each bone. For example, long bones that are exposed to the largest torsional and load-bearing forces and flat bones that serve a protective function, such as the skull, are composed roughly of 80–100% cortical bone and 0–20% cancellous bone. In contrast, bones that transmit predominately weight-bearing forces, such as the vertebral bodies, consist of 80% cancellous bone and 20% cortical bone. The trabeculae of cancellous bone are arranged according to the lines of stress to which they are exposed.
Bone structure
Woven and lamellar bone
Bone tissue is categorized into woven and lamellar types based on the organization of its main structural protein – type I collagen fibers. In woven bone, the collagen fibers are arranged in a seemingly haphazard feltwork, while in lamellar bone they are deposited in parallel arrays (Fig. 1.3).
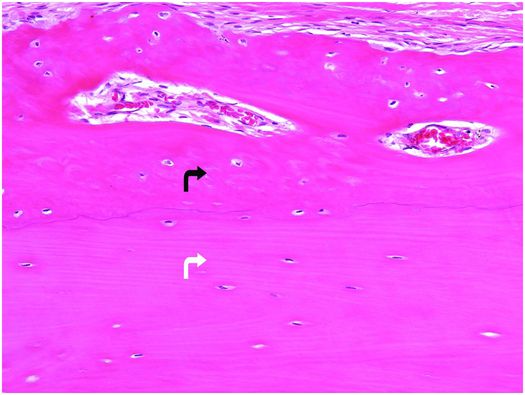
Figure 1.3 Outer portion of cortex composed of lamellar bone (white arrow) that has superiosteal reactive woven bone (black arrow) on its surface. The type I collagen fibers in the woven bone are oriented in a weave, whereas those in the underlying cortex are arranged in parallel array. The osteocytes in the woven bone are more numerous and larger than those in the lamellar bone.
Woven bone is fabricated during periods of rapid bone growth or formation. It composes parts of the developing skeleton during embryogenesis and portions of bones in the growing infant and adolescent. It may also be the predominant type of bone that is formed in a variety of reactive (fracture-callus, infection-involucrum) and neoplastic (Codman’s triangle, matrix of bone-forming neoplasms) conditions. In the adult, woven bone is always pathologic, except at tendoligamentous insertion sites, where small amounts of woven bone are often present.
Histologically, woven bone is hypercellular, and the osteocytes and their lacunae are large and appear to be distributed in a haphazard fashion as the long axes of the cells parallel the differing orientation of the neighboring collagen fibers (Fig. 1.3). Overall, this structural organization enables woven bone to resist forces equally in all directions and facilitates rapid formation, mineralization, and resorption. These factors explain why woven bone is weaker, less rigid, and more flexible than lamellar bone.
Normally, the entire mature skeleton is composed solely of lamellar bone. In contrast to woven bone, lamellar bone is synthesized more slowly, is less cellular, and the osteocytes and their lacunae are smaller and distributed amongst the regularly oriented collagen lamellae, giving it a more orderly appearance (Fig. 1.3). Since the mineral and collagen fibers are well-organized and intimately bound to one another, lamellar bone has greater rigidity and tensile strength and less elasticity than woven bone.
Cortical bone
Cortical bone is composed of dense compact bone, and its thickness depends on its location and mechanical requirements (Fig. 1.4). Cortices are thickest in regions exposed to large torsional forces, such as the central portion of the diaphysis, and thinnest where the transmission of torsional forces is smallest, as seen adjacent to articular surfaces, within vertebral bodies, and adjacent to the subperiosteal bone collar (SPBC), which borders the physis (3, 4).
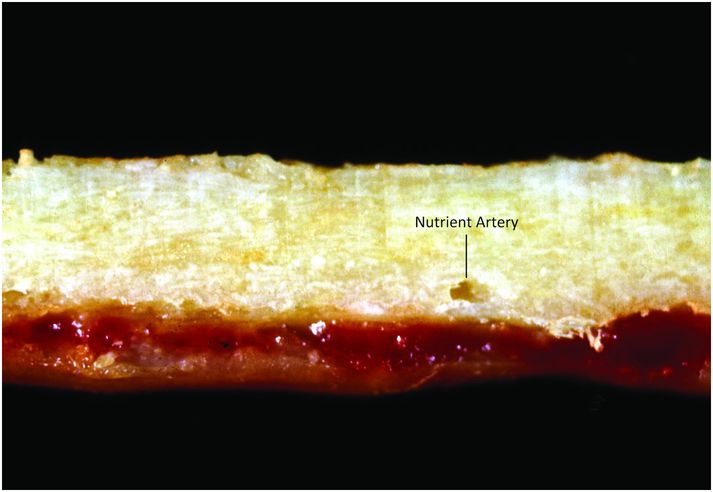
Figure 1.4 Cortical bone is dense and compact; note the foramen for the nutrient artery.
Mature cortical bone is composed of three different architectural lamellar patterns: circumferential, concentric, and interstitial (Fig. 1.5). The circumferential lamellae form outer and inner envelopes to the cortex and consist of several subperiosteal and endosteal layers that are oriented parallel to the long axis of the bone. They are the first cortical lamellae to be deposited, and in young individuals comprise almost the entire cortex. As mechanical stresses on the bone increase with age, many of the circumferential lamellae (except for several lamellae just beneath the periosteum and along the endosteum) are replaced by the concentric lamellae of the haversian systems. Haversian systems, or osteons, are initially created by osteoclastic resorption of the circumferential lamellae, and this process usually begins on the endosteal surface of the cortex, and less frequently on the periosteal surface. The accrual of layers of concentric bone over time reduces the diameter of the haversian canal so that in the end it is small and contains nutritional blood vessels and nerve twigs (Fig. 1.5). Together, these elements define a haversian or osteonal system.
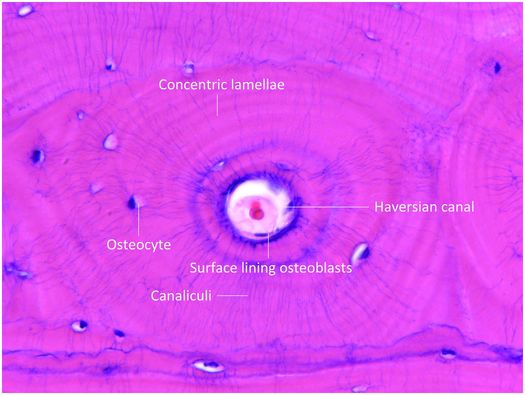
Figure 1.5 Histology of haversian system composed of the canal and its contents, concentric lamellae, osteocytes and the surface lining osteoblasts. The canaliculi containing osteocyte cell processes are prominent.
Mature haversian canals are long and cylindrical, range from 25 to 125 μm in diameter (average 50 μm), and are widest nearest the medullary cavity. They form an intricate, branching, spiraling, and interconnecting network that courses throughout the cortex. The number of haversian systems in a particular bone is variable and is determined by age, the amount of mechanical stress and weight the bone is subjected to over time, and other biologic and genetic factors.
Filling the spaces between the haversian systems is the interstitial bone. The interstitial lamellae represent the remnants of concentric lamellae of previously formed haversian systems that have become partially destroyed by osteoclastic activity. Interstitial lamellae are irregular, geometric-shaped units of lamellar bone that help “glue”, or anneal haversian systems to one another – this arrangement is important in maintaining cortical integrity. As the bone is subjected to varying forces and remodeling, newly formed haversian systems replace pre-existing interstitial lamellae and older haversian systems subsequently become newly created interstitial lamellae.
Cancellous bone
Cancellous bone is fenestrated, and is situated within the medullary cavity (Figs 1.6, 1.7). It consists of interconnecting plates and struts of trabecular bone, and its total surface area is very large, which facilitates remodeling, and the ability of the skeleton to respond rapidly to the metabolic demands of the body. Bone trabeculae are deposited according to lines of mechanical stress to provide support and distribute large weight-bearing forces along a variety of different pathways. Consequently, cancellous bone is most abundant in the weight-bearing ends of bones, such as the epiphyses and metaphyses of long bones and vertebral bodies, whereas it is present in only small quantities in the mid diaphysis of tubular bones.
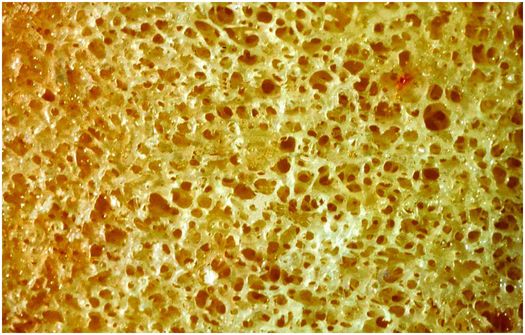
Figure 1.6 Cancellous bone is composed of interconnecting plates of trabeculae creating a large surface area for cell activity.
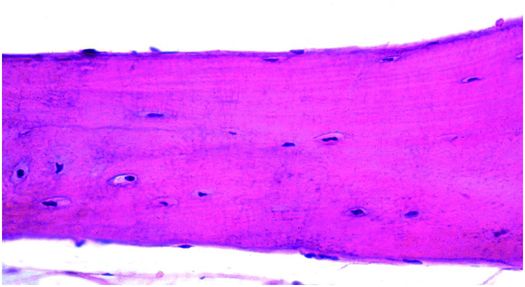
Figure 1.7 Trabeculae composed of the lamellae that are oriented in the same direction as the trabeculae.
Periosteum and perichondrium
The periosteum consists of a thin layer of tan-white connective tissue that covers the outer surface of all cortices. Where this layer of tissue overlies cartilage, such as that of the growth plate and adjacent immature epiphysis, it is known as the perichondrium. In children the periosteum is relatively loosely attached to the cortex, whereas in adults it is firmly anchored. The periosteum and perichondrium is constructed of an outer fibrous layer and an inner cellular or cambium (osteogenic) layer and these layers are most apparent during periods of rapid bone growth in children. The cambium layer is composed of fibroblasts, osteo- and chondro-progenitor cells, and developing osteoblasts and chondroblasts (Fig. 1.8). Generally, the number of progenitor cells present depends on the age of the child and the amount of bone cell activity in any particular region; they are especially numerous during periods of active bone formation. In contrast, in adults the periosteum appears largely as a fibrous layer that contains fibroblasts and broad collagen fibers that are continuous with those of the joint capsule, tendons, and muscle fascia. Collagen fibers of tendoligamentous structures pierce the periosteum and become anchored in the bone (Sharpey’s fibers).
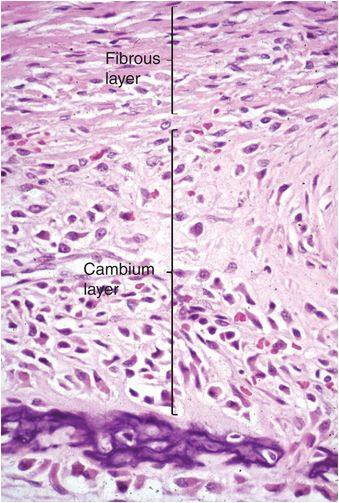
Figure 1.8 Fetal periosteum is composed of an outer fibrous layer containing collagen, and an inner cellular region known as the cambium (osteogenic) layer.
Vascular and nerve supply
Bones are very vascular and receive their blood supply from three main sources: (a) large nutrient arteries (one to two per bone); (b) metaphyseal and epiphyseal vessels; and (c) periosteal vessels. Nutrient arteries enter long bones in the diaphysis, traverse the cortex through foramina, and divide into ascending and descending branches within the medullary cavity. Smaller branch arteries, arterioles, capillaries, venules, and veins traverse the medullary cavity, nourish the fatty and hematopoietic marrow, and extend into haversian canals, where they supply the inner two-thirds of the cortex. At the ends of growing bones, small arteries give rise to a rich bed of capillary loops adjacent to the bases of the growth plates. The epiphyseal and metaphyseal vessels access bone through small apertures and provide blood flow to regions of the epiphysis and metaphysis in the mature skeleton and to the secondary centers of ossification during active enchondral ossification. The periosteal vessels are small and are believed to nourish the outer third of the cortex. The venous drainage system of bone is composed of medullary sinusoids that empty into a central venous sinus, which merges with nutrient veins.
Nonmyelinated nerves that are derived from the autonomic nervous system are the main source of innervation of bones, and their function is to control blood flow. Larger nerve branches are usually associated with arterial vessels, whereas small groups of fibers can be found adjacent to vessels in haversian systems. Nerves supplying the periosteum contain sensory elements and are responsible for the generation of bone pain.
Bone cells
The cells of bone are of different types and include the osteoblast lineage, osteoclast lineage, fibroblasts, adipocytes, smooth muscle cells found in vessel walls, endothelium, axonal processes, Schwann cells, and the hematopoietic elements. The cells responsible for the formation and remodeling of bone tissue are the osteoblast and osteoclast lineages.
Osteoblast lineage
Osteoprogenitor cells are derived from tissue-bound mesenchymal stem cells located in the peri-anlage tissue of the fetus, the periosteum, the haversian and volkman canals, and the medullary cavity. Osteoprogenitor cells are primitive committed mesenchymal cells that produce offspring that develop into osteoblasts. The process of osteoblast differentiation and maturation is complex and involves a variety of molecules and signaling pathways (5–14). Morphologically, the osteoprogenitor cells have the features of spindle cells, and do not have any distinguishing histologic characteristics.
Osteoblasts are responsible for the production, transport, and arrangement of most of the components of the organic bone matrix (osteoid). Importantly, they also regulate matrix mineralization, and use autocrine and paracrine mechanisms to influence the activity of neighboring bone cells. Osteoblasts have a lifespan that ranges from months to many years, and their metabolic state is reflected in their morphology. Osteoblasts actively synthesizing bone are polyhedral, have abundant cytoplasm that is in intimate contact with the bone-forming surface, and their nuclei are polarized away from the matrix surface (Fig. 1.9). As their synthetic activity diminishes, they become more attenuated and elongate (spindle-shaped) and remain as a cellular lining covering all bone surfaces (Fig. 1.10).
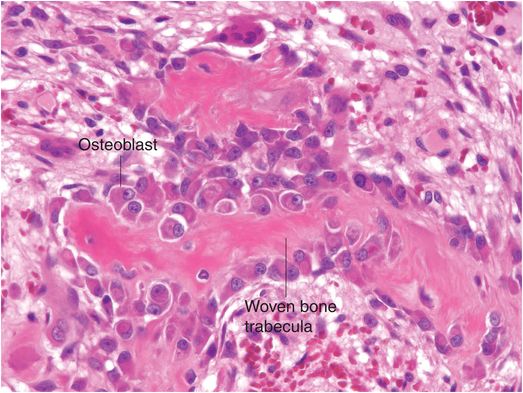
Figure 1.9 Metabolically active osteoblasts line woven bone trabeculae. The large osteoblasts have abundant cytoplasm and the nuclei tend to be oriented away from the bone-forming surface.
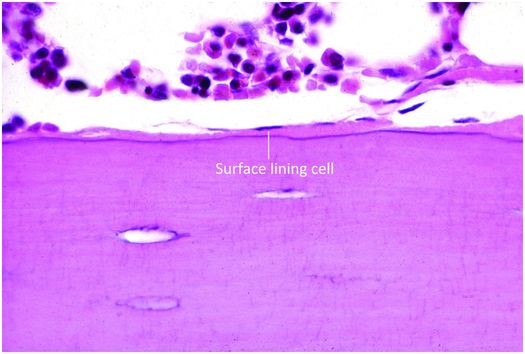
Figure 1.10 Quiescent osteoblasts, known as surface lining cells, covering trabeculae.
Osteoblasts enveloped by bone matrix are known as osteocytes (Fig. 1.5), and they are the most numerous cell type of the osteoblast lineage (15). The number, size, shape, and position of osteocytes vary according to the type of bone they inhabit. In woven bone, they are numerous, large, and plump, and are comparatively fewer in number, smaller, and more elongate in lamellar bone (Fig. 1.3). Osteocytes reside in lacunar spaces that house the cell body, nucleus, and surrounding scant cytoplasm. Osteocytes have numerous long and delicate cytoplasmic processes that extend beyond the lacuna, and traverse the matrix through small channels termed canaliculi (Fig. 1.5). This arrangement creates a very large surface area of contact between the osteocyte and the matrix and extracellular fluid that bathes each cell. Osteocyte cell processes connect to those of neighboring osteocytes and to surface osteoblasts via gap junctions. Gap junctions are specialized to facilitate the transfer of small molecules and biologically generated electrical potentials from one cell to another. In this manner, osteocytes communicate with one another and form a complex and integrated network throughout bone tissue.
The repertoire of biologic activity possessed by osteocytes helps them maintain bone tissue, and allows bone to be responsive to the mechanical and metabolic requirements of the body. As mechanosensory cells they translate mechanical forces into biologic activity (16–18). The detection of physical forces stimulates osteocytes to produce and release intercellular messengers that target surface lining cells, precursor cells, and osteoclasts (16–19). These cells, in turn, respond by remodeling the bone regionally as the structure and mass of the bone is altered according to the demands of the external physical environment. Osteocytes also generate and respond to microfluxes in ion concentrations and mediate the exchange of calcium and other ions between the bone matrix and extracellular fluid. In certain conditions, they may even be able to rapidly release calcium and phosphorus from the mineralized matrix by a process termed osteocytic osteolysis, and this manifests histologically as enlarged lacunar spaces (20). Additionally, osteocytes produce fibroblast growth factor-23, a hormone essential in the regulation of serum phosphorus as it modulates the reabsorption of this element in the renal tubule (17).
Osteoclasts
Osteoclasts are multinucleated cells responsible for the resorption of bone and cartilage. The matrix they break down must be mineralized for the process to be initiated and completed. Osteoclasts are mobile cells that have a lifespan of only several weeks. By the time they are recognizable by light microscopy they are fully differentiated and biologically active and reside within resorption pits (Howship’s lacunae) formed by their digestion of mineralized bone matrix (Fig. 1.11).
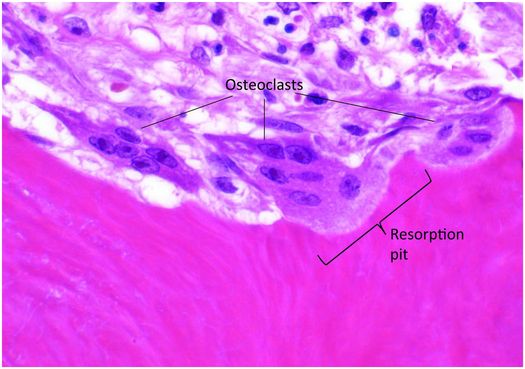
Figure 1.11 Three multinucleated osteoclasts reside in their respective resorption pits, which appear as scallops on the bone surface.
Osteoclasts are 40–100 μm in diameter and are polarized, with one portion of the cell membrane intimately attached to the bone and the remainder exposed to the extracellular fluid in its microenvironment. The cytoplasm in the vicinity of the resorbing surface is rich in lysosomes containing a variety of enzymes, and when their contents are released into the resorption pit, the actual process of bone digestion begins. Once osteoclast activity concludes and the cell moves to another targeted site, macrophages migrate into the base of the resorption pit and break down the organic remnants.
Osteoclasts are derived from mononuclear, hematopoietic progenitor cells of the granulocytic-macrophage colony-forming (GM-CFU) and macrophage colony-forming units (M-CFU) (21). The process of formation and activation of osteoclasts is controlled by a paracrine system that includes the receptor activator for nuclear factor κβ (RANK), RANK ligand (RANKL), and osteoprotegerin (OPG) (22–25).
RANK is a member of the tumor necrosis factor (TNF) family of receptors expressed mainly on cells of macrophage/monocytic lineage, such as preosteoclasts. When this receptor binds its specific ligand (RANKL), which is expressed by osteoblasts and marrow stromal cells through cell-to-cell contact, a series of signal cascades is activated and osteoclastogenesis is initiated. Another member of the TNF family of receptors that can block the actions of RANKL, is OPG, a soluble protein produced by a number of tissues, including bone, hematopoietic marrow cells, and immune cells. OPG inhibits osteoclastogenesis by acting as a decoy receptor that binds to RANKL, thus blocking the interaction of RANK with RANKL. The communication between bone cells and these molecules permits osteoblasts and stromal cells to control osteoclast development. This ensures the tight coupling of bone formation and resorption vital to the success of the skeletal system and provides a mechanism for a wide variety of biologic mediators (hormones, cytokines, and growth factors) to influence the homeostasis of bone tissue.
Skeletal growth and development: bone formation, growth, modeling, and remodeling
Beginning in the embryo until the stage that adult stature is attained, the bones of the body undergo a significant increase in size, refinement of shape, and enhancement of contour. Being rigid, bone cannot grow interstitially and only enlarges by the apposition of new bone on its surface. In contrast, cartilage has the capacity for both appositional and interstitial growth; it increases its substance and enlarges in all dimensions by adding new cells that elaborate freshly synthesized extracellular matrix internally, and on its surfaces. These characteristics of bone and cartilage form the foundation of the two major processes of formation and growth of the skeleton known as enchondral and intramembranous ossification. In enchondral ossification a cartilage model is replaced by bone and in intramembranous ossification bone tissue is formed directly by precursor cells residing in a membranous layer of fibrous tissue.
Enchondral ossification and growth plate cartilage
The increase in length of tubular bones in embryos and prepubertal children occurs as growing cartilage is replaced by bone, with the majority of the growth derived from the cartilage anlage (cartilage model of the future bone) and growth plate (physis). The cartilage anlage originally develops in the early stages of embryogenesis from mesenchymal cells that form cellular condensations at the sites of future bones (26, 27). These mesenchymal cells differentiate into chondrocytes that produce a cartilage model or anlage of the forthcoming bone (Fig. 1.12). This process commences at a specific time for individual bones, and the temporal sequence of anlage formation is the same in all humans. Surrounding the newly formed cartilage anlage are several layers of mesenchymal cells that form the perichondrium, which gives rise to progenitor cells that differentiate into chondrocytes (Fig. 1.12). Subsequently, a specific portion of the perichondrium transforms into periosteum to initiate ossification. This process first occurs in the mid portion of the cartilaginous shaft where mesenchymal stem cells in the perichondrium begin to produce a layer of osteoblasts that deposit a collar of woven mineralized bone on the surface of the cartilage model. This anatomic site is known as the primary center of ossification (Fig. 1.13).
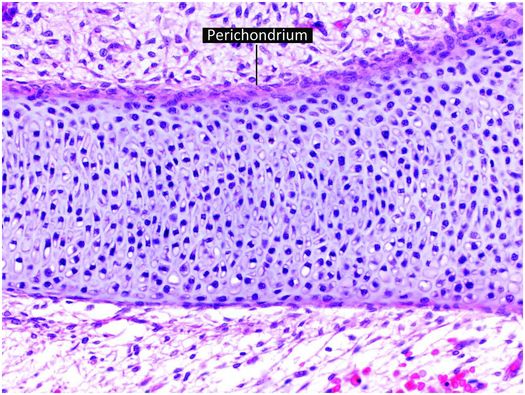
Figure 1.12 Cartilage anlage composed of hyaline cartilage. The perichondrium covers the surface of the structure.
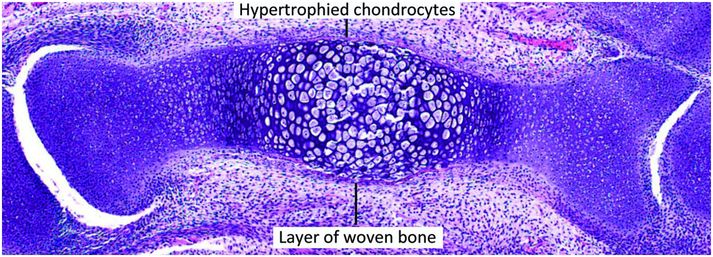
Figure 1.13 Cartilage anlage of a metacarpal containing a primary center of ossification in an early stage of development. In the primary center the chondrocytes are hypertrophied and surrounded peripherally by a very thin layer of woven bone.
Concurrently, the chondrocytes in the interior of the cartilaginous shaft that are encased by the periosteal shell of bone begin to hypertrophy and swell (Figs. 1.13, 1.14). Apoptosis ensues and the surrounding matrix mineralizes. During this time the periosteal vessels give rise to a capillary network that, with the aid of osteoclastic (chondroclastic) resorption, penetrates the woven bone of the primary center of ossification into the mineralized cartilage. The capillaries are the precursors to the future nutrient vessels and are accompanied by pluripotent mesenchymal cells that give rise to immature osteoprogenitor and osteoclast progenitor cells.
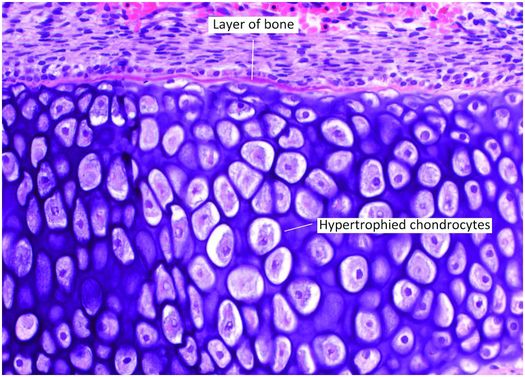
Figure 1.14 Primary center of ossification that has recently formed. A delicate layer of bone rimmed by osteoblasts covers the central region of hypertrophied chondrocytes.
Osteoclasts derived from this tissue continue to bore into the cartilaginous core of the bone leaving residual longitudinally oriented struts of matrix. These bars of cartilage act as scaffolding for newly formed bone deposited by osteoblasts originating from mesenchymal stem cells. Composed of a central cartilaginous core covered by a layer of woven bone, these first trabeculae form the primary spongiosa. Progressive digestion of cartilage creates spaces that coalesce and form the medullary cavity (Fig. 1.15). The medullary cavity is initially filled with loose connective tissue, but eventually becomes occupied by varying amounts of fat and hematopoietic elements. This complex process of cartilage replacement by bone progresses toward both ends as the bone lengthens.
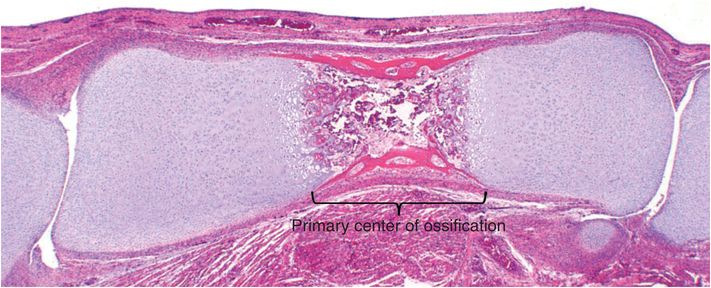
Figure 1.15 Well-formed primary center of ossification moving towards both ends of the bones creating a medullary cavity.
In most long bones, a similar process develops subsequently in the epiphysis, and this region is known as the secondary center of ossification (Fig. 1.16). In the secondary center of ossification the maturation and replacement of the cartilage anlage is identical to that which occurs in the diaphysis, except that the maturation proceeds from the center centrifugally, toward the periphery. This means that the growing area of the secondary center is, at first, a sphere. Continual growth of the primary and secondary centers of ossification results in the mergence of their reserve zones. As this occurs, a horizontal plate of bone is deposited along the base of the secondary center, which demarcates it from the primary center of ossification; henceforth, the centrifugal growth of the epiphysis becomes hemispheric. The cartilage located at the base of the true articular cartilage is responsible for progressive epiphyseal enlargement, and it has the architectural organization of growth plate cartilage. Variation in the subarticular growth results in concordance of the shapes of the ends of articulating bones. The epiphysis receives its nutrition primarily from blood vessels within the bone and its adjacent periosteum, whereas the true articular cartilage is nourished by synovial fluid.
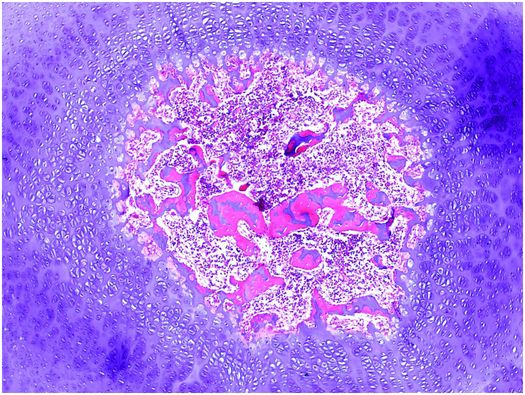
Figure 1.16 Secondary center of ossification has developed in the epiphysis. Growth is occurring in a centrifugal fashion.
A secondary-like center of ossification also appears in the apophyseal cartilage, located on the surface of the bone (Fig. 1.1). It is responsible for the formation of apophyseal bone – some examples are the iliac crests, the greater and lesser trochanters of the femur, and the humeral and tibial tuberosities.
Once the plate of bone that separates the secondary and primary centers of ossification from one another is deposited, the final form of the growth plate is attained. The periphery of the physis is encircled by perichondrium known as the ring of Lacroix and it merges with the periosteum adjacent to the metaphysis and with the perichondrium that surrounds the epiphysis (Fig. 1.17) (28). This structure helps protect against injury and provides precursor cells for lateral expansion of the growth plate. The anatomic zone that extends from the junction of the epiphyseal cartilage and the reserve zone of the growth plate to the junction of the proliferative and hypertrophic zone, where there is frequently a subtle increase in diameter of the physis, is called “the groove of Ranvier” (Fig. 1.17) (3, 26). The appearance of the groove varies according to the specific growth plate anatomic region.
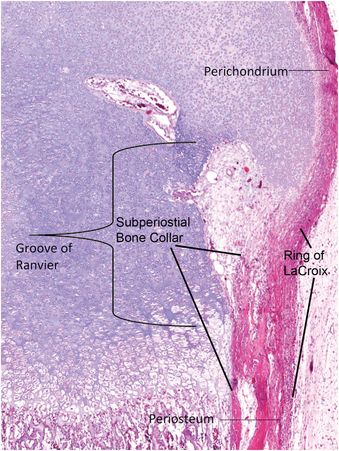
Figure 1.17 Perichondrium extends from the epiphysis to the base of the growth plate where it merges with the periosteum. The portion that spans the growth plate is known as the ring of Lacroix and beneath it is the SPBC. The anatomic zone that extends from the junction of the epiphyseal cartilage and the reserve zone of the growth plate to the junction of the proliferative and hypertrophic zone, where there is frequently a subtle increase in diameter of the physis, is called “the groove of Ranvier.”
The perichondrium adjacent to the distal portion of the growth plate deposits an encompassing rim of bone known as the subperiosteal bone collar (SPBC) (Fig. 1.18) (3). The length of the SPBC is variable; in one study of a 3-month-old infant the lateral SPBC of the distal tibia measured 2.5 mm and medially it was 1.7 mm long (29). The SPBC acts as a lateral buttress providing mechanic support to the physis and often has a distinct step-off with the obliquely oriented metaphyseal cortex with which it is in contiguity (3, 4, 30, 31). Radiographic visualization of the SPBC is a normal finding in children between the ages of one month and two years (3). The natural step-off contour at its junction with the metaphyseal cortex should not be mistaken for a fracture; sometimes it may appear as a small bony spur that hugs the periphery of the growth plate (32).
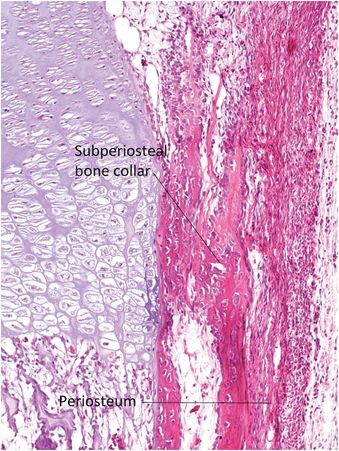
Figure 1.18 Magnified region of Fig. 1.17. The SPBC forms beneath the periosteum and borders the lateral aspect of the growth plate that supports it. The SPBC merges with the adjacent metaphyseal cortex.
The growth plate with its inherent chondrocyte proliferation, matrix elaboration, and hypertrophy in the direction of bone growth are the primary determinants of linear bone enlargement (2). This process is coordinated, and tightly regulated by secreted growth factors (Table 1.1) that stimulate chondrocyte-specific transcription factors (SOX9, GLI2/2, and RUNX2), and systemic hormones (Table 1.2), all of which interact to orchestrate and ensure the continuous elongation and eventual closure of the physis (2, 26, 33–37).
Insulin-like growth factor 1 and 2 |
Indian hedgehog |
WNT |
Bone morphogenic proteins |
Fibroblast growth factors |
Vascular endothelial growth factor |
C-type naturietic peptide |
Transforming growth factor beta |
Glycosylphosphatidylinositol |
Growth hormone |
Thyroid hormone |
Parathyroid hormone-related protein |
Estrogen |
Testosterone |
Glucocorticoids |
In the growth plate the chondrocytes are arranged in merging regions that correspond to different stages of chondrocyte proliferation and maturation (Fig. 1.19). As the cells pass through different stages of their lifespan they do not literally move within the matrix but mature in the position they occupy when first formed. The stages of maturation are reflected in their morphology and these features form the basis of their division into different zones including: (a) a region of resting or reserve chondrocytes located nearest the ends of the bone; (b) a region of proliferating chondrocytes that become arranged in spiral columns; (c) a region of chondrocyte hypertrophy; (d) a region of chondrocyte apoptotic necrosis and matrix mineralization; and (e) a region of cartilage resorption by osteoclasts.
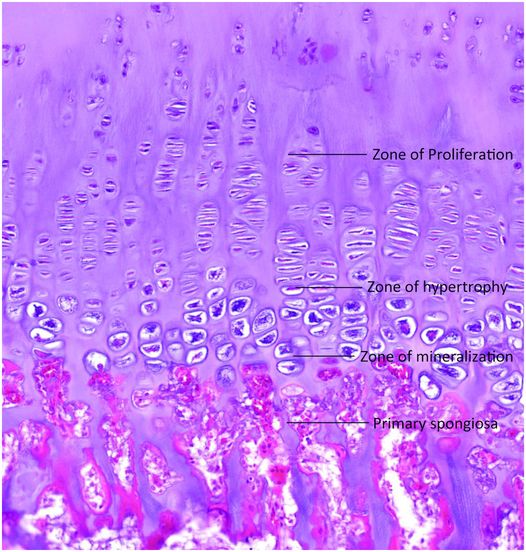
Figure 1.19 Well-formed growth plate extending from the superior aspect of the zone of proliferation and including the zones of hypertrophy and mineralization. The primary spongiosa are in continuity with the base of the plate.
The chondrocytes in the reserve zone are relatively small, round, or oval, surrounded by abundant matrix, and those at its base give rise to those that form the zone of proliferation. The chondrocytes in the zone of proliferation are flattened, undergo cell division, become arranged in spiral columns, and elaborate extracellular matrix (26). In the zone of hypertrophy the chondrocytes enlarge with the cytoplasmic volume increasing 10 times (26), resulting in a transverse diameter of up to 30 μm, and the cells model the surrounding matrix as they increase in size (Fig. 1.20) (2). In the zone of mineralization or calcifying cartilage (sometimes considered a sub-region of the hypertrophic zone), the chondrocytes secrete matrix vesicles that are derived from the cell membrane, and they control mineralization of the surrounding extracellular matrix (2, 26, 38). Three to five longitudinally oriented chondrocytes are associated with the calcified matrix, and this area measures approximately 90–150 μm in length (39). This mineralized region of the physis has also been designated the histologic zone of provisional calcification (hZPC) (40). As the matrix mineralizes the chondrocytes undergo rapid necrosis in the last row of lacunae before the ossification front. This process is associated with the release of cytokines, which attracts the in-growth of endothelial-lined blood vessels and osteoclasts that tunnel into the mineralized matrix, digesting the transverse septae of matrix that separates the chondrocytes from one another (Fig. 1.21). As a result, the vertically oriented intercolumnar struts of mineralized cartilage remain (2, 34, 35); their orientation is determined by the pre-existing columnar arrangement of the chondrocytes in the proliferative and hypertrophied zones and parallels the long axis of the bone (Figs 1.19–1.21). These struts of cartilage become the scaffolding for newly deposited bone.
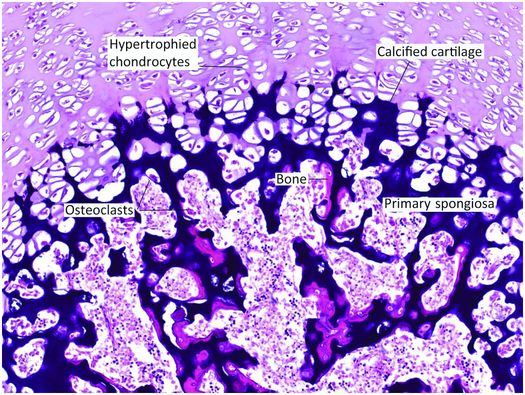
Figure 1.20 Undecalcified section of the base of the growth plate. The zone of hypertrophy contains enlarged chondrocytes and merges with the zone of mineralization in which the intervening cartilage matrix is calcified and appears dark purple in this micrograph. Osteoclasts resorb portions of cartilage leaving behind struts that act as the scaffolding for osteoblasts, which deposit newly formed bone that is pink in this image. The structures that contain the cartilage cores covered by bony surfaces adjacent to the growth plate are known as the primary spongiosa.
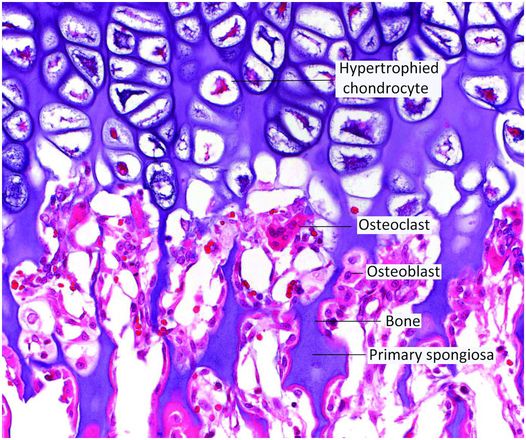
Figure 1.21 Decalcified section of the base of the growth plate showing osteoclasts resorbing portions of the zone of mineralization. This process is vital for the formation of the primary spongiosa. The amount of marrow space surrounding the primary spongiosa is limited in quantity.
In a growing long bone the rate of resorption of mineralized cartilage at the chondro-osseous junction (COJ) is balanced by the rate of chondrocyte proliferation in the zone of proliferation. Studies in the rat have shown that chondrocytes spend an average of 4 days in the proliferating zone and at least 48 hours in the zone of hypertrophy (41). The analogous time course in humans is unknown, but experimental studies have shown that up to eight chondrocytes in an individual column of chondrocytes undergo necrosis per day in rapidly growing rat bones (42), and that five or six die per day in four-week-old pigs (41). Growth of bones in humans and pigs are known to be similar to one another; therefore, it is reasonable to estimate that in newborn humans 4–7 chondrocytes undergo necrosis per day per column of chondrocytes. Studies have found that in the costochondral junction (CCJ) of newborn infants there are 12.6 ± 1.0 chondrocytes per column, and of these 39.6 ± 6.9% are proliferating chondrocytes, and that the number of chondrocytes per column decreases with age (43).
The rate of growth differs for each physis and is greatest in the growth plate of the distal femur, followed by that of the proximal tibia. Generally, the faster the growth rate of the physis, the greater the contribution of growth is derived from cell hypertrophy and the smaller the contribution is from matrix synthesis (35). In diseases in which mineralization of the cartilage is delayed (e.g., rickets), removal of the cartilage is impaired, and the zone of hypertrophy becomes massively and irregularly thickened. While most tubular long bones have two epiphyseal growth plates, other bones (such as the ribs and some of the phalanges, carpals, tarsals, metacarpals, and metatarsals) have only a single physis.
Once enchondral ossification is underway in the growth plate, the primary spongiosa becomes well developed and measures approximately 250 µm in longitudinal dimension (Figs. 1.19–1.21) (39). It is estimated that 60% of the zone of calcified cartilage is resorbed by osteoclasts and 40% is preserved in the form of the cartilage cores of the primary spongiosa (44, 45). The fact that these calcified cartilage cores are in continuity with the zone of calcification of the growth plate which interdigitates and merges with the remaining cartilage mass of the growth plate is thought to help protect the growth plate from side slippage (46). The marrow spaces between the individual newly formed trabeculae of the primary spongiosa are relatively limited in quantity (Figs 1.19–1.21) and this impacts the radiographic appearance of this anatomic region. For it is mainly the combination of the mineralized cartilage in the primary spongiosa and the adjacent zone of mineralization of the growth plate that produces the discrete homogeneous opacity along the COJ known as the radiologic ZPC (rZPC) (Fig. 1.22) (40). The rZPC has been measured to be 416 µm long by high-resolution micro-CT or less than 1 mm in length by plain radiography (29, 47). This contrasts with the hZPC dimension of 118 µm. The discordant radiologic and histologic dimensions of the ZPC have been studied in depth in fetal piglets (40).
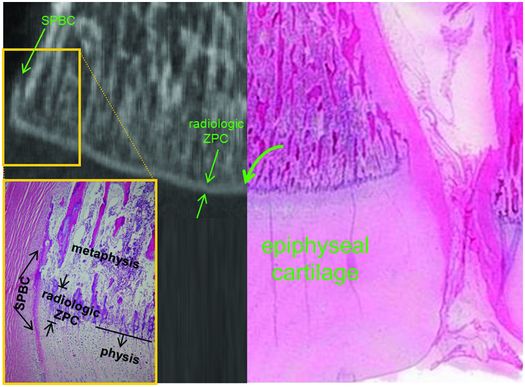
Figure 1.22 Micro-CT with histologic correlate of the region of the left distal tibial COJ. Composite showing the histology of the lateral half of the tibia as well as the coronal micro-CT reformatted image of the medial half of the bone illustrating the spatial relationship of the physis and the rZPC. Note the rZPC corresponds histologically to the densely staining cartilage rich region of the metaphyseal primary spongiosa (curved arrow). Low power photomicrograph of the boxed area demonstrates the SPBC and the rZPC spatially localized in the histologic section. (Reprinted with permission from Tsai A, McDonald AG, Rosenberg AE, Gupta R, Kleinman PK. High-resolution CT with histopathologic correlates of the classic metaphyseal lesion of infant abuse. Pediatr Radiol. 2014;44(2):124–40.)
The fate of the primary spongiosa is either complete osteoclastic resorption or continued deposition of bone on their surfaces while the cartilage is being resorbed (39). These growing trabeculae are known as the secondary spongiosa, and they are comparatively larger in size and fewer in number, and there is an associated increase in the volume of the intervening marrow space. The region that encompasses the relatively abrupt change from the primary to secondary spongiosa is the trabecular transition zone (Fig. 1.23) (29, 40).
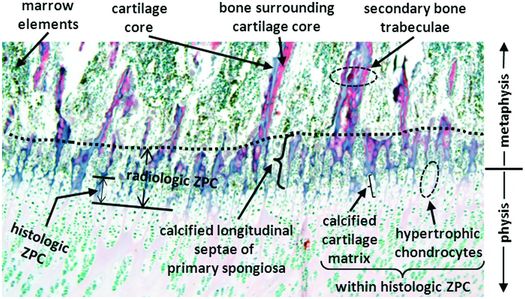
Figure 1.23 Histologic correlate of rZPC showing the region of the COJ. Note the sharp transition in density and thickness of the longitudinal bony trabeculae within the metaphysis (dashed line), which corresponds spatially to the metaphyseal margin of the rZPC in the trabecular transition zone. (Reprinted with permission from Tsai A, McDonald AG, Rosenberg AE, Gupta R, Kleinman PK. High-resolution CT with histopathologic correlates of the classic metaphyseal lesion of infant abuse. Pediatr Radiol. 2014;44(2):124–40.)
Dramatic changes also occur in the cortex during this time period of rapid growth. Increase in bone diameter is accompanied by subperiosteal bone deposition and bone resorption along the endosteum so that the cortical thickness remains proportionally uniform and the medullary cavity enlarges. The bone that first forms the cortex is woven in nature; but within the first several years of life, the fabricated bone is lamellar in architecture. Variation in the rate of formation and resorption alters the shape of the bone, and this process is most pronounced in the region just distal to the base of the growth plate, known as the “cut back” zone. The “cut back” zone, which forms the metaphysis, contains numerous subperiosteal osteoclasts that reduce the diameter of the bone to that of the diaphysis, and this results in “funnelization” of the bone.
The metaphysis is defined as the widened end of a long bone, situated between the physis and the diaphysis (Fig. 1.2). This region contains abundant trabecular bone composed of the primary and secondary spongiosa in children and mature lamellar trabeculae in the adult. The demarcation between the end of the physis and the beginning of the metaphysis is defined as the last intact transverse septum along the base of each physeal cartilage cell column. In contrast, the demarcation between the metaphysis and the diaphysis is less discrete, corresponding roughly to where the funneling of the metaphysis ceases. The cortex of the metaphysis is thin relative to that of the diaphysis.
In the diaphysis, the cortical thickness is maintained or increases by appositional new bone formation. During growth and development, the diameter of the diaphysis continues to enlarge and in specific sites becomes asymmetric. This process is dynamic and responsive to mechanical forces, and not only determines the eventual diameter of the bone, but controls the thickness and contour of the cortex, and the arrangement of the trabeculae. The expanding medullary cavity becomes largely free of spicules of cancellous bone in much of the diaphysis and becomes filled with adipose tissue and hematopoietic marrow. Conditions altering the balance of bone formation and resorption may cause abnormally thickened or significantly thinned (osteoporotic) cortices.
A variety of hormones regulate bone growth, and their concentrations cause the growth spurt that occurs during puberty. As this process waxes and wanes chondrocyte proliferation decreases, however, maturation and bone formation proceed. This eventually leads to complete enchondral ossification and, at this time, the growth plate is considered closed and all additional bone growth is appositional (34). Cessation of growth of the secondary centers of ossification occurs in a similar fashion. However, a remnant of mineralized growth cartilage, which is the tidemark cartilage, persists at the base of the articular surface (Fig. 1.24). It is demarcated from the true articular cartilage by a thin undulating layer of more densely mineralized matrix, known as the tidemark (Fig. 1.24). The biologic potential of the tidemark cartilage persists as increases in hormones, such as growth hormone in the setting of acromegaly, can reactivate the process of enchondral ossification and produce additional growth in the adult. In normal circumstances, however, the vestige of the growth cartilage remains dormant, and functions as an anchor of the true articular cartilage to the subchondral bone plate (Fig. 1.24).
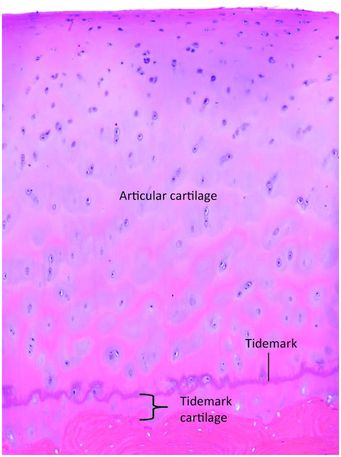
Figure 1.24 End of a large long bone containing true articular cartilage and at its base is the tidemark cartilage. The undulating purple line is the tidemark and it delineates the true articular cartilage from the tidemark cartilage, which is the remnant of growth plate-like cartilage. The tidemark cartilage is anchored to the underlying bone.
Intramembranous ossification
The fibrous membrane rich in osteoprogenitor cells that is the basis of intramembranous ossification develops from the mesenchymal condensations in the embryo, and the periosteum in the fetus, child, and adult (48). The osteoprogenitor cells produce offspring that differentiate into mature osteoblasts that deposit bone matrix. Large portions of the flat bones of the skull, including the frontal, parietal, occipital, and temporal bones, form by this process. Also, since the cortices of all bones are largely created by osteoblasts derived from the periosteum, all bones, in at least some part, are formed by intramembranous ossification. Growth of membranous bone occurs only by apposition of new bone, and the medullary cavities are created and maintained by endosteal osteoclastic activity. In the case of fracture callus, bone formation may occur both via intramembranous and enchondral ossification.
Modeling and remodeling
The processes of bone formation and resorption are tightly coupled, and their balance determines skeletal mass at any point in time (49, 50). As the skeleton grows and enlarges (undergoes modeling) during childhood and young adulthood, bone formation predominates, whereas after the third or fourth decades of life bone resorption prevails. The breakdown and renewal of bone fundamental to the formation and maintenance of the skeleton is called remodeling. Remodeling is a dynamic process involving the removal and replenishment of both cortical and trabecular bone; it continues throughout life to maintain bone mass, skeletal integrity, and skeletal function (49, 50). This process is complex and at least partially controlled by the interplay of different organ systems, including the peripheral sympathetic nervous system (51), and by mechanically induced microdamage. It depends on the integrated actions of osteoblasts, osteocytes, reversal cells, and osteoclasts (52), and, in adults, is responsible for turning over approximately 10% of the skeleton on an annual basis (49, 50).
Sites vulnerable to trauma in developing long bones
Growing bones are composed of an admixture of tissues of differing consistencies and quantities; therefore, they are susceptible to trauma. Although any portion of long bones may fracture, the metaphyseal–epiphyseal region is a distinctive site of injury in children. A region of fracture susceptibility to shear strains in the young infant is the trabecular transition zone (29, 40). In this zone, the density of bone-covered mineralized cartilage of the primary spongiosa is greater than that of the adjacent secondary spongiosa, providing a potential plane of trabecular failure when exposed to excessive mechanical loads. After infancy, the location of fractures shifts to involve the physeal cartilage, because of the concurrent increase in thickness of the juxtaphyseal metaphyseal bony trabeculae, which enhances its physical strength relative to the adjacent growth plate (53).
A closely related site of vulnerability of the infant metaphysis lies near the SPBC (Figs 1.17, 1.18) (29, 32, 54, 55). Fractures through the trabecular transition zone are limited from extending directly to the periphery by the thick bony collar that surrounds this region. Thus, planar fractures involving the trabecular transition zone characteristically extend to the bone surface at the junction of the SPBC and the metaphyseal cortex (Figs. 1.17, 1.18), resulting in the varied radiographic appearances of the classic metaphyseal lesion (CML) of infant abuse (32, 54, 55), an injury that will be discussed in depth in subsequent chapters.
Summary
The skeleton is a complex structure that plays a variety of different roles crucial to life. It is composed of many individual bones that are constructed of proteins, minerals, and the cells specific to bone, namely, the families of osteoblasts and osteoclasts. Bones form through enchondral and intramembranous ossification, and these processes are complex and tightly regulated by molecular genetics and cell-signaling pathways. Understanding the structure of the skeleton and its growth and development provides the foundation to understand its susceptibility to trauma and the pathology induced by injury and tissue damage.