The prenatal period and placental physiology
Overview of pregnancy
The duration of pregnancy averages 266 days (38 weeks) after ovulation, or 280 days (40 weeks) after the first day of the last menstrual period (Figure 3-1). This equals 10 lunar months, or just over 9 calendar months. During these months, the almost solid uterus, with a cavity of 10 mL or less, develops into a large, thin-walled organ. The total volume of the contents of the uterus is 5 L or more at term, 500 to 1000 times the original capacity.33
Most of the changes encountered during pregnancy are progressive and can be attributed to either hormonal responses or physical alterations secondary to fetal size. The preimplantation endocrine system controls the reproductive cycle. In the woman, this involves the cyclic release of pituitary gonadotropins and secretion of estrogen and progesterone by the ovary (see Chapter 2).
First trimester
Physical signs associated with pregnancy include Goodell’s sign (softening of the cervix and vagina with increased leukorrheal discharge), Hegar’s sign (softening and increased compressibility of the lower uterine segment), and Chadwick’s sign (bluish purple discoloration of the vaginal mucosa, cervix, and vulva) by 8 weeks. Although a presumptive sign of pregnancy, Chadwick’s sign is only useful in primiparous women. By 8 to 10 weeks, fetal heart tones can be auscultated by Doppler ultrasonography. Real-time ultrasound can pick up fetal heart movements earlier. Maternal cardiovascular changes are also occurring, with stroke volume and cardiac output increasing and systemic vascular resistance decreasing. These changes contribute to increased renal plasma flow and glomerular filtration.33 Weight gain during the first trimester is usually small.
Second trimester
The increasing vascularity of the vagina and pelvic viscera may result in increased sensitivity and heightened arousal and sexual interest. Mucorrhea is not uncommon as a result of the hyperactivity of the vaginal glandular tissues. This change may increase the pleasure experienced during sexual intercourse. Spontaneous orgasm and multiple orgasms may occur as a result of the increased congestion. Leukorrhea often occurs, with thick, white, acidotic (pH of 3.5 to 6.0) discharge that may contribute to inhibition of pathogenic colonization of the vagina.33 Perineal structures also enlarge as a result of the vasocongestion, increased vascularity, hypertrophy of the perineal body, and fat deposition that began during the first trimester.
Third trimester
The heart is displaced slightly to the left as a result of the increased pressure from the enlarged uterus. Blood pressure rises slightly, and cardiac output remains unchanged. Blood volume peaks at 28 to 34 weeks’ gestation. Dependent edema frequently occurs as blood return from the lower extremities is reduced. Increasing pelvic congestion, relaxation of the smooth muscle in the veins, and the increased pressure of the growing fetus may result in varicosities of the perineum and rectum. Constipation and obesity may lead to development of engorged blood vessels.33
The increased elasticity of connective and collagen tissue leads to relaxation and hypermobility of the pelvic joints. Separation of the symphysis pubis results in instability of the sacroiliac joint. The center of gravity shifts lower with development of a progressive lordosis to compensate for the anterior shift of the uterus. Balance is maintained by an enhanced cervicodorsal curvature, leading to difficulty in walking and the characteristic waddling gait. Stress on the ligaments and muscles of the middle and lower back and spine may lead to discomfort and back pain.33
Conception
For conception to occur, a precise set of sequential events must take place. The probability of a viable conception per menstrual cycle is less than 40% to 50%.25,103 The process of conception and fetal survival is selective, as evidenced by implantation failures and the approximately 50% anomaly rate encountered in spontaneously aborted fetuses.103,130 Gametogenesis is described in Chapter 1. The ovarian and endometrial cycles necessary for conception and early support of the fertilized ovum, as well as follicle maturation, are described in Chapter 2. This section will examine ovulation, sperm transport, fertilization, cleavage, and zygote transport.
Ovulation
The ovary is responsible for two important functions: gametogenesis and steroid hormone synthesis. Integration of ovarian steroid synthesis, follicle maturation, ovulation, and corpus luteum function is essential for fertilization and implantation. Estrogen and progesterone have significant effects on tubal and uterine motility, endometrial proliferation, and the properties of the cervical mucus.33 In order for fertilization to take place, the oocyte must become “fertilization competent” (see Chapter 1). The close proximity of the oocytes and follicular cells in the ovary allows the follicle and oocyte to communicate bidirectionally via gap junctions (intracellular membrane channels) in order to work together to control meiotic arrest and resumption, follicle maturation; ovulation; and corpus luteum formation, function, and regression.26 The hypothalamus and anterior pituitary regulate these latter morphologic changes through secretion of gonadotropin-releasing hormone (GnRH) and gonadotropins. Follicle-stimulating hormone (FSH) and luteinizing hormone (LH) act synergistically (see Chapter 2). Endocrine interactions in follicle maturation are illustrated in Figure 3-2.
Usually only one follicle matures and is ovulated, although the exact mechanism for this is unknown. At the beginning of the menstrual cycle, up to 15 to 20 primary (pre-antral) follicles are stimulated by FSH, but only 6 to 12 enlarge. Of these growing follicles, several develop into antral follicles. Eventually one follicle becomes dominant and begins to function independent of FSH. This follicle secretes inhibin, which inhibits pituitary FSH. Because the other maturing follicles are still dependent on FSH, which is now decreased, they begin to regress and degenerate.25 The process of follicular development and maturation is described in the section on the Ovarian Cycle in Chapter 2. The ovary during reproduction is illustrated in Figure 2-21.
In the fully developed follicle (Graafian or tertiary follicle), multiple layers of granulosa cells line the antral side of the basement membrane (membrane granulosa), and a cumulus of granulosa cells surrounds the oocyte. Proliferation of these cells is stimulated by growth differentiation factor 9 (GDF9), which is a member of the transforming growth factor β family.130 GDF9 is also important for oocyte maturation and differentiation. Oxygen and nutrients diffuse across the granulosa cells to the oocyte. Antral fluid contains proteins, enzymes, proteoglycans, and hormones such as FSH and steroids.25 The oocyte is surrounded by the zona pellucida, which contains sperm receptors. The external theca layers around the follicle consist of the outer theca externa (capsular like covering) and vascularized, glandular inner theca interna. Under the stimulation of FSH, the theca and granulosa cells produce large amounts of estrogen (primarily estradiol), which peak about 24 hours before ovulation. Production of estrogen stimulates proliferation of the endometrium, thinning of cervical mucus, and LH secretion.130
LH levels rise, which increases production of progesterone and inhibin A by the dominant follicle through interaction of LH with LH receptors on granulosa cells. The rise in progesterone occurs 12 to 24 hours before ovulation and elicits a rapid and marked surge in LH secretion, paralleling the mid-cycle FSH peak (see Chapter 2). The LH peak is essential for ovulation, which occurs 28 to 36 hours later.25,33 The mid-cycle surge of LH initiates ovulation by stimulating prostaglandin (PGE and PGF) synthesis, leading to formation of collagenase and other proteolytic enzymes with disruption of the gap junctions between the oocyte and follicular cells.25 The LH surge also increases concentration of maturation promoting factors, which disrupts meiotic inhibition and initiates completion of the first meiotic division.130 The oocyte completes its first meiotic division 10 to 12 hours before ovulation, forming the secondary oocyte (23 chromosomes plus most of the cell cytoplasm) and first polar body (23 chromosomes and minimal cytoplasm). The small polar body is nonfunctional and degenerates (see Chapter 1). The LH surge also causes a decrease in estradiol production.
Ovulation begins with a protrusion or bulge on the ovarian wall. A small avascular spot (stigma) develops, forms a vesicle, and ruptures, extruding the secondary oocyte, follicular fluid, and surrounding cells. Rupture is thought to be caused by enzymatic digestion of the follicular wall via the action of proteases (e.g., collagenase, plasmin, and hyaluronic acid), which dissolve connective tissues.58,103 The oocyte is surrounded by the zona pellucida and corona radiata (radially arranged granulosa cells). The second meiotic division begins with ovulation, then arrests in metaphase.130 The second meiotic division is not completed until fertilization. The oocyte is swept by the fimbriae into the fallopian tube. Muscular contraction of the tube and, primarily, beating of the cilia move the ovum along the tube to the ampulla (the usual site of fertilization). If unfertilized, the ovum usually dies within 24 hours.103
Corpus luteum
After ovulation, the follicular walls and theca collapse inward and become vascularized (Figure 3-3). The granulosa cells undergo a luteinizing process to form the corpus luteum. The corpus luteum secretes progesterone, beginning within 30 to 40 hours of the LH surge. A small amount of estrogen is secreted by the theca cells.25 If fertilization has taken place, implantation occurs during the latter part of this week. Around the time of implantation, the trophoblast tissue secretes human chorionic gonadotropin (hCG), a luteotropin that stimulates the corpus luteum to continue to function. hCG may alter the metabolism of the uterus to prevent the release of substances that result in luteal regression. The corpus luteum can only produce progesterone for about 10 days without hCG stimulation.25 If implantation does not occur, hCG is not produced, the corpus luteum begins to regress, undergoing apoptosis (mediated by uterine leutolytic factors such as prostaglandins), and involution begins.25 The decline in steroid hormones results in menstruation.
The corpus luteum is essential for continuation of the pregnancy until the placenta has developed the capacity to secrete estrogens and progesterone. Removal of the corpus luteum prior to this time usually leads to a miscarriage.130 From 6 to 10 weeks, there is a transition period in which both the placenta and corpus luteum are producing hormones; by 7 weeks the placenta is capable of producing sufficient progesterone to maintain pregnancy if needed. At 6 to 8 weeks, there is a dip in progesterone levels, indicating a decline in corpus luteum functioning. This is followed by a secondary rise in progesterone (presumably as a result of placental takeover) without a rise in the metabolite 17α-hydroxyprogesterone (secreted by the corpus luteum). Around 32 weeks there is a more gradual rise in this metabolite, indicating increased placental utilization of fetal precursors.
Sperm transport
Spermatozoa have not completely differentiated when they are released into the lumen of the seminiferous tubules (see Chapter 1). They are nonmotile and incapable of fertilization. Mature sperm have a condensed and genetically inactive nucleus. Reactivation of the nucleus occurs once the sperm enters the cytoplasm of the ovum.161 Sperm are moved down the seminiferous tubules and through the epididymis and vas deferens by (1) the pressure of additional sperm forming behind them, (2) seminal fluid, and (3) peristaltic action. Biochemical and morphologic maturation of the sperm occurs during their 14 to 21 day passage through the epididymis. Further modifications occur after ejaculation so that the sperm can bind to the zona pellucida of the ovum. Sperm are stored in the vas deferens and epididymis before ejaculation. Ejaculation occurs through the urethra with contraction of the ampulla and the ejaculatory duct upon orgasm.
The volume of ejaculate ranges from 2 to 6 (mean 3.5) mL and usually contains 100 million sperm per mL.103 Men with less than 10 million sperm per mL are likely to be sterile.103 Some spermatozoa are immature, senescent, or abnormal, and generally only the normal and strongest sperm are able to complete the journey within the female reproductive tract to the upper end of the fallopian tube. As sperm move along the epididymis, they begin to gain motility. Sperm become fully motile in the semen after entering the female reproductive tract.161 Semen provides fructose for energy and an alkaline pH for protection against the acid environment of the vagina; it also dilutes the sperm to improve motility. Sperm move at 2 to 3 mm per minute. Motility is slower in the acidic vaginal environment and faster in the alkaline uterine environment.103 Failure of sperm to achieve motility is a cause of male infertility; for potential fertility, at least 40% should be motile by 2 hours after ejaculation.103
The neck and midpiece of the spermatozoa contain a pair of centrioles, the base of the tail apparatus, and the mitochondrial sheath. The mitochondria are arranged in a tight helical spiral around the anterior portion of the flagellum (tail). Mitochondria supply the adenosine triphosphate (ATP) required for independent motility. Sperm must reach the ovum within an allotted time or they exhaust their energy supply and die. Sperm survival in the uterus is relatively short because phagocytosis by leukocytes begins within a few hours. Sperm retain their ability to fertilize the ovum for 1 to 3 days.132 However, most sperm do not survive for more than 48 hours.25,103
Once deposited at the external cervical os, some ejaculated sperm cross the cervical mucus facilitated by a decrease in mucus viscosity at mid-cycle (9 to 16 days), allowing for more rapid migration. Within minutes, these sperm enter the uterine cavity, although some get caught in cervical crypts and endometrial glands. The cervical crypts provide a short-term reservoir or storage site from which sperm are gradually released; this may increase the chance of fertilization.103 Uterine motility, stimulated by prostaglandins in seminal fluid that cause smooth muscle contraction, facilitates initial sperm transport.68 Other sperm move more slowly (2 to 3 mm/hr) or are stored in cervical crypts and slowly released.25
Sperm chemotaxis (organized movement of the sperm toward the ovum) is stimulated by chemoattractants in follicular fluid, and possibly the cumulus oorphus and ovum. Other components of follicular fluid that may also act as chemoattractants include heparin, progesterone, atrial natriuretic peptide, epinephrine, oxytocin, calcitonin, and acetylcholine.44 Capacitated sperm appear to be responsive to a sperm chemotrophic factor and other chemicals released by the follicle ovum and use these substances to “find” the ovum.132
Fertilization
The process of fertilization has been defined in three different ways: (1) the instant of sperm and ovum fusion, (2) time from sperm-ovum fusion to development of the male and female pronuclei, and (3) time from sperm-ovum fusion to the first mitotic division (about 24 hours). Fertilization begins with contact between the sperm and secondary oocyte, arrested in the metaphase of the second meiotic division (see Chapter 1). Fertilization usually occurs in the upper third of the fallopian tube, usually in the ampulla. Before fertilization, the sperm must undergo two final maturational changes: capacitation and the acrosome reaction.
Capacitation involves removal of the glycoprotein coat and seminal plasma proteins from the plasma membrane over the acrosome (head of the sperm), which allows the acrosome reaction to occur. Capacitation takes about 7 hours and usually occurs in the fallopian tubes while the sperm are attached to the tubal epithelial lining, but may begin while the sperm is still in the uterus.25 This process is stimulated by substances in the female genital tract and follicular fluid.58,103 For example, albumin in genital tract secretions stimulates loss of fatty acids and cholesterol from the sperm plasma membrane. This increases permeability of the sperm plasma membrane and initiates capacitation and the acrosome reaction.
Capacitated sperm are chemotaxically active.44 Approximately 2% to 14% of sperm are capacitated at any time, with continued replacement of sperm that lose their capacitation with newly capacitated sperm. Each sperm can only become capacitated once in its lifespan.44 This constant replacement of capacitated sperm extends the time when fertilization is possible by continuous production of “ripe” sperm.44 Thus after ejaculation precapacitated, capacitated, and postcapacitated sperm, as well as sperm that have undergone the acrosomal reaction, can be found within the female genital tract.
Of the millions of sperm in the ejaculate, only up to 300 to 500 sperm are found in the fallopian tubes at any given time.25,130 The ampulla of the ovulatory tube has more sperm than the ampulla of the nonovulatory tube. Although it takes only one sperm to penetrate the ovum, it appears that several hundred are necessary to effect passage of the spermatozoa through the corona radiata to the ovum. The number of spermatozoa that are ejaculated does not appear to influence the number of sperm that enter the fallopian tubes unless very low counts occur.
The acrosome reaction with release of enzymes through small holes in the acrosomal membrane must occur for successful penetration of the corona radiata and zona pellucida by the sperm. The acrosome is a saclike structure on the head of the sperm containing many enzymes, including acid glycohydrases, proteases, phosphatases, esterases, and hyaluronidase.103 Acrosin, a serine protease, may be most important.25 The capacitated sperm binds to the zona pellucid of the ovum, initiating the acrosome reaction (sperm activation).155 The sperm penetrates the zona pellucida and binds to the outer membrane of the oocyte (Figure 3-4).
The zona pellucida is an ovum-specific extracellular membrane composed of three glycoproteins (ZP1, ZP2, ZP3) that act as ligands (molecules that bind to receptors) for sperm receptors. ZP3 mediates sperm binding and the acrosomal reaction.130 Roles of the zona pellucida include sperm activation (acrosome reaction), preventing fertilization by more than one sperm, protecting the ovum before fertilization and protecting the fertilized ovum until shortly before implantation.155 Binding of the sperm to ZP3 is mediated by a sperm surface protein (SED1).132 Once a sperm has bound to ZP3, a zonal reaction occurs with release of lysosomal enzymes. This reaction causes physicochemical alterations in the zona pellucida that make it impenetrable to other sperm.
The sperm head traverses the perivillous space between the plasma membranes and zona pellucida and attaches to the surface of the oocyte, and their plasma membranes fuse. This process is mediated by integrins (adhesion molecules) on the ovum surface along with FERTILINβ (also known as ADAM2), IZUMO and other substances produced by the sperm.132 The head and tail of the sperm enter the oocyte, leaving the outer plasma membrane of the sperm attached to the outer membrane of the oocyte. The ovum has a layer of cortical secretory granules along the inside of its plasma membrane. After sperm entry, the sperm-ovum interaction releases a wave of calcium along the zona pellucida resulting in fusion of the cortical granules with the plasma membrane of the ovum and release of hydrolytic enzymes, proteases, and polysaccharides into the perivillous space.25 This modifies the zona pellucida glycoproteins, preventing activation and entry of other sperm.154
After entering the cytoplasm of the oocyte, the sperm undergoes rapid morphologic changes. The tail of the sperm degenerates and the head enlarges to form the male pronucleus. Each pronucleus has 23 chromosomes (22 autosomes and 1 sex chromosome). Sex of the offspring is determined by the male and depends on whether the sperm that enters the ovum contains an X or Y chromosome. The sperm nucleus becomes reactivated so that it can again synthesize DNA and RNA.161 This processing involves removal of the nuclear membrane with exposure of the sperm chromatin to the cytoplasm of the ovum. The nuclear protein is remodeled and the nucleus decondenses, becoming larger and more spherical. A new nuclear envelope develops, forming the male pronucleus and activating DNA transcription and replication. This is thought to be mediated by factors in the cytoplasm of the ovum. This process takes about 3 to 4 hours, during which the developing male pronucleus gradually approaches the female pronucleus.161
The ovum must be metabolically activated. Entry of the sperm into the ovum triggers two events: (1) the cortical and zonal reactions described earlier, which blocks entry of other sperm; and (2) a transient increase in intracellular calcium accompanied by an increase in oxidative metabolism.25 The increased calcium stimulates the oocyte to complete its second meiotic division with extrusion of the second polar body into the perivitelline space. The nucleus enlarges and is called the female pronucleus. The oocyte is now mature and metabolically active.154 Failure of calcium signaling can lead to complete failure (triploidy) or partial failure (abnormalities of chromosomal number of the second meiotic division, cleavage arrest, and alterations in development of the inner cell mass and trophectoderm [trophoblast]). These alterations can result in implantation failure and miscarriage.143
The female and male pronuclei approach each other, their membranes disintegrate, and the nuclei fuse (see Figure 3-4). Chromatin strands intermingle, and the diploid number (46) of chromosomes is restored. The zygote (from the Greek, meaning “yoked together”) is formed, and mitotic division (cleavage) begins. The zygote measures 0.2 mm in diameter and carries the genetic material necessary to create a unique human being. Fertilization results in species variation, with half of the chromosomes coming from the mother and half from the father, mixing the genes each parent originally received from their parents.103
Cleavage and zygote transport
Cleavage involves a series of rapid mitotic cell divisions that begins with the first mitotic division of the zygote and ends with formation of the blastocyst. Cleavage is under the control of mitosis-promoting or maturation-promoting factor (MPF).25 The zygote divides into two daughter cells (blastomeres) about 30 hours after fertilization; each of these cells divides into two smaller cells, which also divide, and so forth (see Figure 3-3). The dividing cells are contained by the zona pellucida and become progressively smaller with each subsequent division, with no change in the total mass of the zygote. The trophoblast secretes an immunosuppressant protein called early pregnancy factor (EPF) by 24 to 48 hours after fertilization. Pregnancy tests within the first 10 days after fertilization use EPF in maternal serum.103
Cell division occurs every 12 to 24 hours. By 3 to 4 days, the zygote has divided into 8 to 16 blastomeres. Around the eight- to nine-cell stage, the blastomeres realign and form a tight ball of cells mediated by cell surface adhesion glycoproteins. This process, called compaction, allows increased interaction between cells needed for formation of the inner cell mass. This occurs via gap and tight junctions.25
The zygote remains in the ampulla for the first 24 hours, then is propelled down the fallopian tube by ciliary action over the next few days. At the 12- to 16-cell stage (about 3 days after fertilization), the zygote becomes a solid cluster of cells called the morula (from the Latin word for “mulberry,” which it resembles).25 The zygote reaches the uterine cavity 3 to 4 days after fertilization (about 90 hours or 5 days after follicle rupture). Development is now under control of the embryonic genome. Fluid (which provides nutrients) from the uterine cavity enters the morula as the blastocyst is formed.
The blastocyst consists of four distinct components: (1) zona pellucida, a thick glycoprotein membrane that is beginning to stretch and thin; (2) trophectoderm (trophoblast), a one-cell-thick outer layer of flattened cells that will form the placenta and chorion; (3) inner cell mass (embryoblast), a one- or two-cell-thick, crescent-shaped cluster of cells that will form the embryo; and (4) fluid-filled blastocyst cavity.103 The zona pellucida protects the zygote from adhering to the mucosa of the fallopian tube and from rejection by the maternal immune system (see Chapter 13). Position of individual cells and gene transcription factors influence which cells become trophoblast and which become inner cell mass. For example, Oct4 and Nanog are transcription factors found in all blastomeres in the morula. In the cells that become the inner cell mass, these transcription factors continue to be expressed, but are turned off in the cells of the future trophoblast.132 If these gene transcription factors are deficient, all or most of the cells in the blastocyst become part of the trophectoderm, resulting in a molar pregnancy (see Gestational Trophoblast Disease). The blastocyst floats free in the uterine cavity from 90 to 150 hours after ovulation, then begins to implant 6 to 7 days after fertilization (Figure 3-5).
Genetic control of development
Embryonic development combines growth, differentiation, and organization of cellular components at all levels. As development progresses, differential synthesis is established, resulting in cellular differentiation. Growth is the process of creating more of a substance that is already present through increase in cell size and number. In contrast, differentiation is the creation of new types of substances, cells, tissues, and organs that were not previously present. Organization is the process by which these elements are coordinated into functional integrated units. Morphogenesis is the production of a special form, shape, or structure of a cell or group of cells and occurs by the precise organization of cell populations into distinct organs.71
The mechanisms controlling morphogenesis are complex and incompletely understood.25 Development is controlled by developmental gene families within the embryo.21,25,31,52,85 Much of the knowledge of developmental genes to date comes from animal models. Often the names of these genes or their products reflect characteristics of the animals or situations in which they were first identified. Developmental genes control the definition of body axes (ventral/dorsal, anterior/posterior, left/right, medial/dorsal)—and the arrangement of different cells to form tissues and organogenesis.151 These processes involve the coordination of signaling molecules and other proteins, DNA transcription factors, extracellular matrix components, enzymes, and transport systems.40,73 Development genes are involved in cell differentiation and proliferation into adulthood and if later altered can lead to malignancies.56 Imprinted genes (see box on p. 9) are also important in prenatal and placental development as well as in development and function of metabolic processes.121
Although the first cell divisions after fertilization are under maternal genetic control, by the two- to four-cell stage, the embryonic genome is activated and is producing many intercellular signaling proteins and transcription factors.40,61 Transcription factors are proteins that turn other genes on and off, thus controlling expression of these genes. Positive feedback induces further production of regulatory proteins and gene transcription factors that influence that cell or other target cells. Negative feedback results in the production of inhibitors. This process is controlled by interactions of developmental genes with environmental factors that turn the gene on and off at precise intervals. Each gene can produce multiple isotypes, each isotype producing a different product. The different isotypes are produced by the splicing and reorganization of exons within a given gene (see Figure 1-3).85 As a result, a single gene can guide the production of many different forms of mRNA and formation of proteins with unique biologic functions. Thus individual developmental genes may have different functions at different stages of development and with development of specific organs.21,25,31,52
Developmental genes produce signaling molecules and transcription factors. Transcription factors remain within the cell and bind to DNA at the promoter or enhancer regions of specific genes or regulate mRNA production.25,56 Often a cascade is set up, wherein the transcription factor turns on various genes which in turn can regulate other genes. Initially these regulatory genes send out signals that induce expression of other genes, which in turn induce expression of still other genes and so forth until genes that encode development of specific structures or functions of cells or tissues within the embryo are expressed.132 The proteins produced regulate cell activities, such as causing a cell to differentiate in a specific way, and are modulated by positive and negative feedback loops.52 Examples of developmental gene families include the homeobox (HOX) and PAX gene families. For example, the HOX genes are involved with craniocaudal organization; the PAX gene family is involved with development of the urogenital system, central nervous system (CNS), thyroid gland, and eye, among other sites.25
Intercellular signaling molecules (first messengers), many of which are growth factors, influence other cells by binding to receptor molecules (Figure 3-6). Signaling molecules act in a paracrine fashion, that is, they are secreted into the spaces surrounding the cell where the molecules are produced and diffuse between cells in that area.73,130 These molecules act to bind (as ligands) to receptor molecules on cell membranes. After a series of protein interactions, a transcription factor is activated and a signal is sent to the cell nucleus and a gene is expressed resulting in production of specific proteins needed to guide development.25,130 Receptor molecules can be intracellular or on the cell surface. Extracellular receptors are binding sites for ligands (hormones, growth factor, or cytokine). Binding to the receptor alters the receptor and stimulates an intercellular response (signal transduction) either directly via a protein kinase or indirectly via a second messenger such as cyclic adenosine monophosphate (cAMP). Signaling molecules may also act by inhibiting other signaling molecules.25 Justacine signaling also occurs via three mechanisms: (1) interaction of a protein on one cell membrane with a receptor on the surface of another cell; (2) via gap junctions (see Chapter 4 for a discussion of gap junctions), or (3) interaction of extracellular matrix ligands (collagen, proteoglycans, fibronectin, and laminin) with receptors on neighboring cells.130Major signaling pathways are Wnt, Hedgehog, transforming growth factor β (TGF-β) family, tyrosine kinase, Notch, Integrin, and retinoic acid signaling.73,130,132 The Wnt family is involved in the dorsal/ventral axis and formation of the midbrain, muscles, gonads, and kidneys. Alterations are associated with tumors and possibly congenital anomalies.73 The hedgehog family, such as the sonic hedgehog (Shh) gene, is involved in patterning of many tissues and organs, including axis formation, motor neuron induction, somite differentiation, neural tube induction and patterning, and limb patterning.25,56,130,132 Mutations are associated with central nervous system (e.g., holoprosencephaly), axial skeletal, and limb abnormalities as well as with some basal cell carcinomas.25,73 The TGF-β family includes TGF-β, which is important in mesoderm induction and myoblast proliferation, activin (granulose cell proliferation, mesoderm induction), inhibin (inhibition of gonadotropin secretion by the pituitary), müllerian inhibitory substance (regression of the paramesonephric duct (see Chapter 1), bone morphogenetic proteins, and decapentaplegic (limb development).21,25,31,52,130,132 The TGF-β family is also involved in angiogenesis, axon growth, mesoderm differentiation and epithelial branching in the lung and kidneys.130 Defects in TGFβ signaling can lead to vascular and skeletal disorders as well as pulmonary hypertension and cancer.132
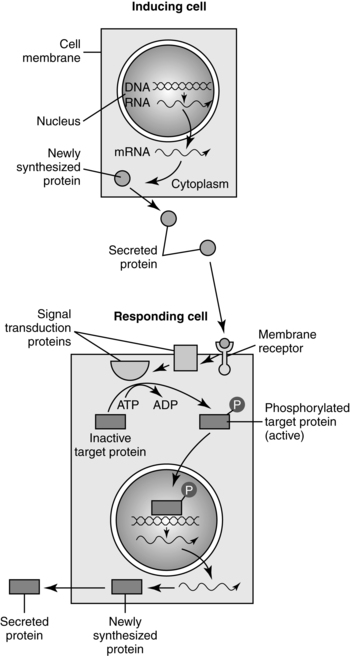
Tyrosine kinase signaling involves growth factors (GFs) such as fibroblast growth factor (FGF), epidermal GF (EGF), insulin-like GFs, platelet derived GFs, and vascular endothelial GF.130,132 FGFs are found in bone, so mutations in FGF receptor genes can lead to skeletal dysplasia and disorders such as achondroplasia, Crouzon syndrome, Apert syndrome, and some forms of craniosyntosis.73 Defects in Notch signaling can lead to skeletal disorders such as Alagille syndrome and spondylocostal dysostosis as well as cancers such as T-cell acute lymphoblastic leukemia.132 Integrins are receptors that are involved in linking the extracellular matrix and cell cytoskeleton and in signal transduction that can lead to changes in cell size, shape, and position.132 Defective integrin signaling can lead to alterations in skin and connective tissue such as epidermolysis bullosa and cancers of the breast, intestine, and reproductive organs.132 Retinoic acid (derived from vitamin A) acts as a morphogen, which is “a diffusible substance that determines cell fate during development in a concentration-dependent manner.”132, p. 161
Alterations in developmental genes and their products can result in congenital anomalies through various mechanisms.41 Mechanical failures involve defects in structural genes such as collagen resulting in qualitative or quantitative differences. For example, collagen mutations are seen in osteogenesis imperfecta, Apert syndrome, and epidermolysis bullosa; a fibrillin defect is seen in Marfan syndrome. Alterations in cell numbers due to regulatory failures can lead to overgrowth, such as occurs in Beckwith-Wiedemann syndrome, or undergrowth, such as occurs with some forms of microcephaly. Failure of cell migration during development leads to anomalies such as lissencephaly (failure of neuronal migration) or Hirschsprung’s disease (failure of neural crest cells to migrate and form ganglia). Failure of the developmental switch, turning genes on and off, can upset the development timetable and also lead to defects.41
Mechanisms of morphogenesis
Cell differentiation
Initially all cells are similar and unspecialized, but each must eventually become 1 of 350 different cell types found in the human body.139 Cells pass through two phases in order to become specialized. In the first phase (determination), the cell becomes restricted in its developmental capabilities and loses the ability to develop in alternative ways. Cell determination occurs for the first time in the blastocyst, with formation of the inner cell mass (which forms the embryo) and trophoblast (which becomes the placenta). In the second phase (differentiation), cells develop distinctive morphologic and functional characteristics. Initially, cell position determines the fate of the cell. Specific differentiation is regulated by interactions between cell populations and is controlled by HOX and other gene families that are switched on to produce specific signaling molecules in a sequential manner.139 Cell differentiation often involves induction (see below) in which one tissue signals (induces) a second tissue (responder) to differentiate into a specific structure. Signals are sent between cells in both directions (cross-talk) to complete the differentiation.130
Induction
Induction is the process by which cells in one part of the embryo influence cells around them to develop in a specific way. Induction requires inductors, or cells that stimulate reactions in surrounding cells via signal transduction and induced tissue, which is made up of cells that have the capacity or competence to respond to these protein signals via cell membrane receptor molecules (see Figure 3-6). At some point, inductors and inducers lose their ability to perform these actions.31
Secondary induction is a cascade of developmental events and is a common way many parts of the embryo are formed. For example, in the nervous system, the notochord is a primary inductor or organizer for brain development. The forebrain reacts to secondary inductors in the mesoderm to form the optic cup, which then induces adjacent ectoderm to form the eye lens. The eye lens then induces epidermis around it to form the corneal epithelium. Other examples of induction are in the formation of the gastrointestinal system, where the gut endoderm induces the surrounding mesenchyme to differentiate into organs such as the liver or pancreas (see Chapter 12) or in the renal system where the utereic bud causes the surrounding mesenchyme to become nephrons (see Chapter 11).130
If any of these steps is interfered with, the next stage in development may not occur, or it may occur abnormally. If these alterations occur early in the developmental sequence, complete organ agenesis may result.103 An example of chemical pathway activity during secondary induction is the interaction of activin and TGF-β, which influences branching of epithelial tubes in the kidney, pancreas, and salivary glands.85,130
Programmed cell death
Programmed cell death or apoptosis is a precisely timed event—under genetic control and cell feedback mechanisms—that occurs in many of the embryonic tissues as part of normal development. The process involves the release of lysosomal hydrolytic enzymes that dissolve cells, thereby altering the tissues. This mechanism is responsible for lumen formation in solid tubes (trachea and parts of the gut) and the disappearance of the webbing between the fingers and toes. If enzyme release is inhibited, syndactyly, some forms of bowel atresia, or imperforate anus may result. If enzyme activity is increased, micromelia (shortened limbs) may result.139
Cell migration
During development some cells move around in a fashion similar to that of an amoeba. This process is dependent on microtubular and microfilament elongation and contraction. Migration involves the elongation of the leading edge of the cell, followed by adhesion of the cell to a new contact point. Contraction of the cell toward the new adhesion site results in movement of the cell. Alterations or interference may limit cell migration and result in a defect. Hirschsprung’s disease (absence of intestinal ganglion cells) results from failure of neural crest cells to migrate. From 3 to 6 months’ gestation, millions of neurons and glial cells within the central nervous system migrate from their point of origin in the periventricular area to their eventual loci in the cerebrum and cerebellum. Alterations in this migration can result in alterations in CNS organization and function (see Chapter 15).103,139
Overview of embryonic development
Preembryonic development occurs from the time of conception and zygote formation until 2 weeks’ gestation. By the time of implantation, the inner cell mass consists of 12 to 15 cells. At about 7 days, the first of three germ cell layers that give rise to the embryo—the hypoblast, or primitive endoderm—appears.25 During the second week the bilaminar embryo develops as the inner cell mass differentiates to form the epiblast along the inner part of the amniotic cavity.
The developing organism appears as a flat disk with a connecting stalk that will become part of the umbilical cord. Cytotrophoblast cells around the inner wall of the blastocyst cavity form the primitive yolk sac (see Figure 3-5, C) and extraembryonic coelom, which serves as transfer interface and nutrient reservoir.69 Connective tissue (extraembryonic mesoderm) fills in the space between the cytotrophoblast cells and the extraembryonic coelomic membrane. Near the end of the second week, cavities appear in the extraembryonic mesoderm and fuse to form the extraembryonic coelom. A secondary yolk sac (see Figure 3-5, D) develops from the primary sac (which gradually disintegrates) and provides for early nutrition of the embryo. Part of it is eventually incorporated into the primitive gut. The fluid in this cavity is an ultrafiltrate of maternal serum with placental and secondary yolk sac products.69 An endodermal cell thickening (prochordal plate) appears at one end of the disk and is the future site of the mouth and cranial region.
The embryonic period lasts from 2 weeks after fertilization until the end of the eighth week. This period is the time of organogenesis. Figure 3-7 summarizes the major stages in development of specific organ systems. Development of specific organ systems is described in detail in Chapters 8 to 20. This section provides an overview of major events during embryogenesis.
The third week of development coincides with the first missed menstrual period (see Figure 3-1). During the third week, growth becomes more rapid, with development of the mesoderm and establishment of the trilaminar embryo; formation of the neural tube (CNS), somites (bones and other supporting structures), and coelom (body cavities); and development of a primitive cardiovascular system.
The primitive streak (thick band of epiblast cells) appears at 15 days in the midline of the dorsal aspect of the embryonic disk.103 Cells in the primitive streak migrate between the endoderm and ectoderm to form the mesoderm; the epiblast layer becomes the ectoderm, establishing the trilaminar embryo. Cells from the mesoderm later migrate out into the embryonic body to become mesenchyme and form supporting tissues.
The ectoderm layer eventually forms the central and peripheral nervous systems, epidermis, hair, nails, inner ear, epithelium of the sensory organs, nasal cavity and mouth, salivary glands, and mucous membranes. The middle mesoderm layer develops into the dermis; muscle; connective tissue; skeleton (tendon, bones, cartilage); circulatory and lymphatic systems; kidneys; gonads; and the lining of the pericardial, pleural, and peritoneal cavities. The endoderm forms the epithelium of the digestive, respiratory, and urinary tracts as well as the thyroid and parathyroid glands.25,103
Development is in a cranial-to-caudal direction, with the embryo initially being a pear-shaped disk with a broad cephalic end and a narrow caudal end. The primitive streak elongates cranially to form a midline rod of cells, or notochord. The notochord extends to the prochordal plate, where the endoderm and ectoderm fuse into the oropharyngeal membrane. Other cells from the primitive streak migrate around the notochord and prochordal plate to form a cardiogenic area where the heart will develop. At the caudal end the endoderm and ectoderm fuse into the cloacal membrane.25,103
The ectoderm over the notochord thickens to form the neural plate, which will eventually form the neural tube, which gives rise to the brain and spinal cord. The mesoderm on either side of the notochord thickens to form two long columns that divide into paired cuboidal bodies (somites). Somites give rise to the skeleton and its associated musculature and much of the dermis. Somite development can be used to distinguish the stage of embryonic development. The foregut and body cavities begin to develop. Mesoderm cells aggregate in the cardiogenic area at 18 to 19 days to form two endocardial tubes, which fuse by 19 to 20 days.25,103 Primitive blood cells and vessels develop in the yolk sac, chorion, and embryo, and by 21 days’ gestation they link with heart tubes to form a primitive cardiovascular system.
The fourth week is a time of body building. The embryo becomes cylindrical and begins to assume a C-shape as a result of transverse and longitudinal folding. The neural tube fuses during days 21 to 28. The cranial area enlarges and develops cephalic and cervical flexure, with the head oriented in the characteristic flexed position. The heart is prominent and begins beating at 20 to 22 days. Small swellings become visible on the lateral body walls at 26 (arm buds) and 28 (leg buds) days. The branchial arches (from which the face, mandible, and pharynx will develop) become visible; however, facial structures are not distinct and human likeness is not clear yet. The embryo is 2 to 5 cm long.25,103
As the embryo enters the fifth week, the form develops a humanlike appearance.103 Head growth is rapid as a result of brain development. The embryo further flexes into the characteristic C-shape and the facial area comes into close approximation with the heart prominence. The forelimbs begin to develop, and paddle-shaped hand plates with digital ridges are visible. The heart chambers are forming, and five distinct areas in the brain are visible. The cranial nerves are present. Retinal pigment and the external ear begin to appear.
The last 2 weeks of the embryonic period are a time of facial, organ system, and neuromuscular development. The head is rounded and more erect although still disproportionately large. The eyes are open, and the eyelids are developing. The eyelids fuse by the end of the eighth week and do not open again until about the twenty-fifth week. The mouth, tongue, and palate are complete. The external ear is distinct, although it is still low on the head. The regions of the limbs are distinct, and elbow and wrist flexion are possible. Fingers are longer and the toes are differentiated. The feet have moved to the midline. The forearms gradually rise above the shoulder level, and the hands often cover the lower face. The abdomen is less protuberant, and the body is covered by thin skin.25,103
Neuromuscular development leads to movement, which can be seen on ultrasound although not felt. The gastrointestinal and genitourinary systems have separated, and the kidneys have achieved their basic structure, although nephron development will continue until 34 to 36 weeks’ gestation. Although the internal genitalia have differentiated, the external genitalia have not. The rectal passage is complete, and the anal membrane is perforated, resulting in an open digestive system.25,103
Overview of fetal development
The fetal period extends from the end of the eighth week of gestation until term. All major systems and external features are established or have begun to develop by the beginning of this period. During the early fetal period (9 to 20 weeks), there is further differentiation of body structures, with a gradual increase in functional ability. By 6 months, the fetus has achieved 60% of its eventual length and 20% of its weight.103 During the late fetal period (20 weeks to term), further maturation of organ and body systems occurs, along with a marked increase in weight. Organization is a prominent feature of this period.
During weeks 9 through 12, the embryo is 5 to 8 cm long and weighs 8 to 14 g. The head is half the body length. The body length doubles during these weeks. Head growth slows down, the neck lengthens, and the chin is lifted off the chest. The face is broad with widely set eyes, fused lids, and low-set ears. Teeth begin forming under the gums, and the palate fuses. Fingernails become apparent, and the arms reach their final relative length.25,103
From 13 to 16 weeks, rapid growth continues. The length of the embryo almost doubles during these weeks. The embryo weighs 20 g by the end of the sixteenth week.103 The eyes and ears have achieved more normal positions, giving the face a distinctively human look. Fetal skin is extremely thin, and lanugo is present. There is increased muscle and bone development, which along with establishment of initial neuromuscular connections results in increased fetal movements. Skeletal ossification continues during this period. Brown fat deposition and meconium formation begin.25,103
Growth slows slightly during the next month (17 to 20 weeks), and the legs reach their final relative positions. During this period, fetal movement is felt by the first-time mother (earlier with successive pregnancies). Fetal heart tones are now audible with a stethoscope. Myelinization of the spinal cord begins. Head hair, eyelashes, and eyebrows can be seen. The sebaceous glands are active, resulting in vernix caseosa deposition. Lung development continues as bronchial branching is completed and terminal air sacs begin to develop. The pulmonary capillary bed is forming in preparation for gas exchange. By 20 weeks’ gestation, the fetus weighs about 300 g and is 25 cm long.103
After 20 weeks, weight increases substantially. By 24 to 25 weeks, the fetus weighs 650 to 780 g and is 30 cm long.103 The body is better proportioned. The skin is translucent, and subcutaneous fat has yet to be laid down. Fingerprint and footprint ridges are formed, and the eye is structurally complete.
From 29 weeks to term, fat and muscle tissue are laid down and skin thickness increases. Although the bones are fully formed, ossification is not complete. Vernix caseosa and lanugo begin to disappear as term gestation is approached and growth slows. The testes descend into the scrotum. The infant fills the uterine cavity, and the extremities are flexed against the body. Myelinization and skeletal ossification progresses, and sleep-wake cycles are established. By 38 to 40 weeks, fetal size averages 3000 to 3800 g and 45 to 50 cm.103
Intrauterine environment
The maternal body provides the fetus with a darkened environment, which may become grayer as the uterus grows and stretches. Auditory stimuli are rich and include the sound of blood flow through the umbilical cord. Most of these sounds are patterned and rhythmic. Extrauterine sounds, such as voices and music, are transmitted in a muted form to the fetus. The maternal system maintains a warm thermal environment. Kinesthetic and vestibular stimulation are provided by maternal movement and changes in position. Other stimuli that influence the activity and responses of the fetus and neonate include maternal biorhythms and diurnal, circadian, and sleep-wake cycles. Exposure of the fetus to these stimuli and events provides appropriate experiences that enhance neurologic organization and establishment of synaptic connections (see Chapter 15). These activities are critical for successful transition to extrauterine life and for establishing physiologic and social relationships necessary to ensure survival.
The placenta and placental physiology
Placental development
Implantation
Implantation is mediated by a coordinated sequence of interactions between maternal and embryonic cells.42 Implantation involves three distinct processes: (1) loss of the zona pellucida (“hatching” of the blastocyst) 5 days after fertilization, followed by rapid proliferation of the trophectoderm to form the trophoblast cell mass; (2) adherence of the blastocyst to the endometrial surface, which leads to the decidual reaction; and (3) erosion of the epithelium of the endometrial surface, with burrowing of the blastocyst beneath the surface.46,84,132 With hatching, the blastocyst acquires the ability to attach to the uterus.67 This involves expression of Perlecon (a heparin sulfate proteoglycans), which binds specifically to extracellular matrix proteins.132 Both implantation and placentation require ongoing communication (cross-talk) between the developing blastocyst and maternal endometrium via hormones, cytokines, growth factors, and other immunoregulatory substances.42
The endometrium must undergo physiologic changes in order for implantation to occur, with a narrow period of maximal uterine receptivity (“window of implantation”) for implantation.42 The ideal window for synchronization between the uterus and blastocyst and thus normal implantation is thought to occur around 8 days after ovulation.87,112,130 Specific receptors (particularly αvβ3) appear opening the implantation window; this window is closed several days later.42,68,87,130 Implantation can also occur in other tissues, resulting in ectopic pregnancy, without this restricted time interval. The endometrium prepares for implantation by the cyclic secretion of 17β-estradiol and progesterone. These hormones regulate the expression of growth factors and cytokines in the uterus, which in turn alter the endometrial surface.42
Uterine receptivity is characterized by increased vascularity and edema of the endometrium, increased secretory activity of the endometrial glands, decrease in the polysaccharide matrix surface coating of the epithelial cells, and development of pinopods (microprotrusions) on the epithelial surface.111,132 The pinopods interact with microvilli on the trophoblast during initial attachment of the blastocyst. Markers of endometrial receptivity are the appearance of pinopods (that last only 1 to 2 days), cell adhesion molecules such as intregrins, cytokines (especially the interleukin family), homeobox genes and their transcription factors (see “Genetic Control of Development”), growth factors (especially the transforming growth factor-β family), proteases and their inhibitors, and endocrine mediators including estrogens, progesterone, calcitonin, human chorionic gonadotropin (hCG), prolactin, and corticotropin-releasing hormone (CRH).80,112 Adhesion molecules (integrins) form cell surface receptors that develop from days 20 to 24 of the menstrual cycle.
Uterine receptivity must be synchronized with preimplantation, implantation, and placentation signaling by the zygote and blastocyst. Substances involved in this signaling include (1) early pregnancy factor (EPF); (2) preimplantation factor (PIF); (3) growth factors such as epidermal growth factor, transforming growth factor–α, platelet-derived growth factor, insulin-like growth factors (IGFs), tumor necrosis factor–α (TNF-α), and colony stimulating factor–1; (4) immunoregulatory cytokines such as interleukin-1 (IL-1), interleukin-6 (IL-6), and TGF-β; (5) cyclooxygenase-2 and prostaglandins; (6) platelet activity factor (PAF); (7) vascular endothelial growth factor; and (8) hCG.42,67,81,112 Growth factors such as TNF-α are found by the two- to eight-cell stage; others appear several days later.42 The zygote develops receptors for cytokines and growth factors by the two-cell stage; by the blastocyst stage, these receptors are seen only on trophectoderm tissue.42 Before implantation the trophoblast is activated. This process occurs 5 to 6 days after fertilization and lasts about 24 hours.77 The initial signaling between the trophoblast and luminal epithelium is closely linked with immunologic mechanisms (see Chapter 13).55,67,81,112
By 5 to 6 days after fertilization (7 to 9 days after ovulation), the blastocyst rests on and adheres to the endometrium.67 Metabolism increases, with localized changes seen at the eventual site of the implantation beginning up to 24 hours before adherence of the blastocyst to the endometrium.84 The place of attachment is usually on the upper posterior wall of the uterus, near the side where the ovary with the corpus luteum is located, but it can occur at various other intrauterine and extrauterine sites.87
Ligands (molecules that bind to receptors) such as cytokines, growth factors, and hormones on the trophectoderm of the hatched blastocyst bind to cell surface adhesion molecules on the surface of the luminal endometrium.77 Initial attachment may be mediated by L-selectin (a carbohydrate binding protein) on the trophoblast and carbohydrate receptors on the uterine epithelium. Further attachment and invasion is mediated by integrins (transmembrane glycoproteins with α and β subunits that can be up-regulated via interaction with other substances), IL-10, metalloproteinases, vascular endothelial GF and L-selectin.68,123 Integrins serve as cell surface receptors for fibrinogen, fibronectin, collagen, and laminin.87 Laminin promotes attachment; fibronectin promotes invasion of the blastocyst into the uterine epithelium.130 In addition, near the time of implantation expression of heparin-binding epidermal growth factor-like GF is up regulated near implantation sites.132 Adhesion (apposition) is initially unstable, relying on interaction between uterine epithelial pinopods and trophoblast microvilli, then stabilizes and is followed by trophoblast invasion of the endometrium.112 The blastocyst orients itself so that the embryonic pole containing the embryo-forming inner cell mass contacts the endometrial surface first.
The trophectoderm (trophoblast) attaches to endometrial extracellular matrix proteins and secretes proteases to degrade these proteins and begins to invade the endometrium. Fingerlike projections of trophoblast cells protrude between the cells of the endometrial epithelium into the endometrial stroma.50 The trophoblast cells then migrate between the cells of the endometrial extracellular matrix until they reach maternal blood vessels. Regulatory substances found on both trophoblast and endometrial tissue enhance interaction, invasion, and trophoblast proliferation. These substances include (1) metalloproteinases (e.g., collagenases, gelatinases, stomelysins); (2) plasminogen activation; (3) plasmin-regulating factors; (4) cytokines such as IL-1β (stimulates trophoblast invasion); and (5) growth factors such as epidermal GF and TGFβ (limits invasion and induces syncytium formation).5,6,42,62,87 Other factors limit trophoblast invasion and develop as part of the decidual reaction. For example, the decidua (i.e., the altered endometrium during pregnancy) secretes protease inhibitors such as TGF-β and tissue inhibitor of metalloproteinase (TIMP). The trophoblast may also autoregulate its invasion via secretion of TGF-β, TIMP, and hCG.42 If trophoblast invasion is too extensive, placenta accreta can result; if invasion is too little, the risk of miscarriage or placental abruption is increased.
By the seventh day after fertilization, the trophoblast begins to differentiate into two layers: the inner cytotrophoblast and the outer syncytiotrophoblast layer.50 The mononuclear cytotrophoblast is a mitotically active layer that forms new syncytial cells, the chorionic villi, and the amnion. The cytotrophoblast serves as a stem cell population to generate new trophoblast cells.55 The syncytiotrophoblast is a thick multinuclear mass, without distinct cell boundaries, that puts out fingerlike projections that invade the endometrial epithelium, engulfing uterine cells (see Figure 3-5). Slight bleeding may occur during this process, which may be mistaken for a scanty, short menstrual period. The trophoblast, primarily the syncytiotrophoblast, produces hCG (which maintains the corpus luteum during early pregnancy) as well as estrogens, progesterone, hPL, and other substances (see “Placental Endocrinology”). The functions of the trophoblast are summarized in Table 3-1.
Table 3-1
FUNCTION | EFFECTORS |
Erosion of maternal tissue to make space for implantation and growth | Proteases (e.g., plasminogen system, matrix metalloproteinases) |
Hormone secretion | hCG, hPL, estrogen, progesterone and others |
Transport nutrients and waste products | Substrate specific transporters, trophoblast, endothelial plasma membranes |
Placental attachment | Adhesion molecules in the extracellular matrix and at the cell surface |
Migration and arterial transformation | Adhesion molecules, proteases, extracellular matrix components |
Adapted from Aplin, J. (2000). Maternal influences on placental development. Semin Cell Dev Biol, 17, 116. hCG, Human chorionic gonadotropin; hPL, human placental lactogen.
Several forms of extravillous trophoblast are derived from the cytotrophoblast. Interstitial trophoblast migrates into the uterine tissue and attaches the anchoring villi of the placenta to the decidua. HLA-G is expressed on the anchoring trophoblast and helps protect fetal tissue from the maternal immune system (see Chapter 13).55 Other extravillous trophoblast migrates out from the placenta into the endometrium and spiral arteries. This type of trophoblast has two roles: (1) conversion of the maternal spiral arteries into low-resistance, high-capacity vessels and (2) formation of plugs at the top of the spiral arteries to limit maternal blood flow into the placenta during the first trimester (see Placental Circulation).55,68,79,87 Implantation is complete by 10 days after fertilization.50 At this point, the blastocyst lies beneath the endometrial surface and is covered by a blood clot and cellular debris. By 10 to 12 days, the endometrial epithelium has regenerated in this area.112
Many ova that are fertilized never implant. One third to one half of all zygotes never become blastocysts; 70% to 75% of blastocysts implant and 51% of these survive to the second week.103 Implantation can be selectively inhibited by administration of low-dose estrogen for several days following sexual intercourse (“morning-after” pill). Estrogen preparations such as diethylstilbestrol act by altering the normal balance of estrogen and progesterone during the secretory phase of the endometrial cycle, making the endometrial lining unsuitable for implantation. Estrogen may also accelerate passage of the zygote along the fallopian tube so that it arrives in the uterus before the secretory phase of the endometrial cycle is established.103
Ectopic pregnancy.
Extrauterine implantation results in an ectopic pregnancy in 1 in every 80 to 250 pregnancies.103 The incidence of ectopic pregnancy has increased fourfold since 1972 and accounts for 10% to 11% of maternal mortality in the United States.33 Ectopic pregnancy is the most common cause of maternal death in the first 20 weeks of pregnancy. Much of the increased incidence in recent years is thought to be a result of the prevalence of sexually transmitted diseases (STDs) and pelvic inflammatory disease (PID). The most common site for an ectopic pregnancy is the isthmus and ampulla of the fallopian tubes.103 This probably results from delay in transport of the zygote from the site of fertilization to the uterine cavity. If transport is delayed, the blastocyst emerges from the zona pellucida while in the fallopian tube and adheres to and implants in tubal mucosa. The delay may be due to tubal adhesions or mucosal damage from PID.103 PID and salpingitis disrupt and damage the tubal mucosa, decreasing the number of cilia, which are essential for timely movement of the zygote along the tube. Alterations in the concentrations of progesterone, estrogen, and prostaglandins may also delay ovum transport.
Endometrium and decidua
The uterine endometrial lining consists of an epithelial layer that contains ciliated and mucus-secreting cells. These cells penetrate into the endometrial stroma and may enter the underlying myometrium. The endometrium is divided into two functional zones (see Figure 2-28). The deepest basalis layer lies adjacent to the myometrium. This layer responds to progesterone stimulus with secretory activity and provides the base for endometrial regeneration after menstrual sloughing.98 The superficial (functionalis) layer of endometrium includes the outer compacta and the middle spongiosa, which contains glands and blood vessels. During the secretory phase of the menstrual cycle, the endometrium undergoes physical changes in preparation for implantation (see Chapter 2).
With conception these changes become more extensive. Under stimulation of progesterone and estrogen, the epithelium and stromal cells become progressively hypertrophic and develop subnuclear vacuoles rich in glycogen and lipids.84,122,146 Early nutrition of the blastocyst is from digestion of substances in endometrial tissue and surrounding capillaries. The endometrial changes during pregnancy are known as the decidual reaction, and the altered endometrial lining is known as the decidua. The decidual reaction involves remodeling of the extracellular matrix with changes in collagen, proteoglycans, and glycoproteins. Estrogen and progesterone pathways that control epithelial and stromal function during implantation and decidualization include (1) CCATT/enhancer binding protein-β (transcription factor); (2) homeobox-10 (transcription factor); (3) bone morphogenetic protein-2 (morphogen); (4) Wnt4 (morphogen); (5) Indian hedgehog (morphogen); and (6) gap junctions.122 A poor decidual reaction is associated with placenta accreta and ectopic pregnancy.42 In addition these pathways are altered in endometriosis leading to impaired implantation.122 Decidualization also involves alterations in local immune cells and processes and changes in maternal spiral arteries (see “Placental Circulation”).19 As decidualization increases, the window of receptivity for implantation is closed.79
In addition to its role in early nutrition of the embryo, the decidua may protect the endometrium and myometrium from uncontrolled invasion by the trophoblast cell mass.84 The decidua also acts as a physical barrier and—via production of cytokines that promote trophoblast attachment, not invasion—to protect the endometrium during the period when trophoblast cells migrate out of the placenta to the maternal spiral arteries (see “Maternal Uteroplacental Circulation”).79 A somewhat hypoxic environment appears to be needed in early pregnancy for trophoblast invasion and differentiation; high oxygen levels may alter morphogenesis.68,79,157
The decidua is divided into three sections (Figure 3-8). The decidua capsularis, just above the area of trophoblast proliferation, initially covers the growing embryo. With development of the chorion, the decidua capsularis gradually regresses. The portion of the decidua on which the blastocyst rests forms a soft, spongy vascular bed known as the decidua basalis, site of the future placenta.4 Large numbers of maternal macrophages, especially maternal natural killer cells, migrate into the decidua basalis (see Chapter 13).108,146 Decidual macrophages are important in maternal tolerance of the implanting blastocyst and cooperate with fetal trophoblast cells in remodeling maternal spiral arteries during pregnancy (see “Placental Circulation”).108 At the interface between the trophoblast and decidua basalis is a specialized extracellular matrix rich in fibrin and fibronectin that supports trophoblast adhesion and migration.4 The remaining portion is known as the decidua parietalis (or decidua vera).
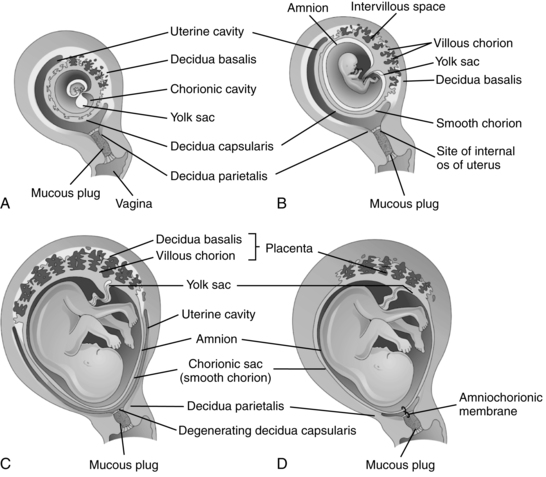
The decidua basalis forms the maternal portion of the placenta and the stratum in which separation of the placenta will occur at delivery.33,103 With embryonic growth, the decidua basalis is progressively compressed. The glands and blood vessels become distorted and assume oblique and horizontal courses. As the embryo fills the lumen of the uterus, the decidua capsularis disappears. By 18 to 20 weeks after conception, the chorion laeve and decidua parietalis meet and fuse, obliterating the uterine cavity (see Figure 3-8).51,84,124
Development of the amniotic cavity
The amniotic cavity appears during the second week following fertilization as the blastocyst is burrowing into the endometrium. Small spaces appear between the inner cell mass and cytotrophoblast. These spaces coalesce to form a narrow amniotic cavity that gradually enlarges to completely surround the fetus (Figure 3-9).
The amniotic cavity develops a thin epithelial roof or lining. This lining is the amnion, which arises from amnioblasts (amniogenic cells) from the cytotrophoblast. The floor of the amniotic cavity is formed from the embryonic epiblast germ layer. Initially a small amount of fluid may be secreted by the amniotic epithelial cells, but the major early source of amniotic fluid is probably maternal serum. With advancing gestation, the epithelial cells of the amnion become more cuboidal or columnar and are covered with microvilli.103
Placentation
As the syncytiotrophoblast proliferates and invades the endometrial stroma, the blastocyst slowly sinks into the endometrium. By 7 to 8 days after fertilization, intersyncytial spaces or lacunae are seen in the syncytiotrophoblast (see Figure 3-5).51 Capillaries in the endometrium grow and surround the syncytiotrophoblast forming a capillary plexus connected to the early lacunae.164 The lacunae fill with a nutritive substance or filtrate containing primarily glandular fluid derived from maternal blood that diffuses through the trophoblast to the embryo. Individual lacunae fuse into lacunar networks that will later develop into the intervillous spaces (IVS) and become filled with maternal blood. Endometrial capillaries around the implanted embryo become congested and dilated, forming sinusoids.
Blood flow into the IVS is limited in the first trimester and the IVS is probably filled primarily with this filtrate of maternal serum and secretions from the endometrial glands.22,23,68,70,116 The highly oxygenated maternal blood does not fill the IVS until fetal vessels are established in the villi and mechanisms to protect the fetus against oxidative stress are established.22,68,70,79 This begins at 8 to 9 weeks and increases after 10 to 12 weeks.22 Before that time, trophoblast plugs fill the tops of the spiral arteries, controlling arterial pressure and limiting blood flow into the IVS.76,124 Oxygen concentration in the IVS at 8 weeks is ≤20 mmHg (3% to 5% O2) versus 60 mmHg (8% to 10% O2) in the surrounding decidua.116 Thus initial placental and embryonic development occurs in a relatively hypoxic environment. This environment may stimulate production of vascular endothelial growth factor and stimulate chorionic vascularization.22,68,70 Low O2 levels may be essential for control of early cardiovascular development by hypoxia inducible factors (HIF).157
HIFs act as mediators to allow cells to adjust to low O2 conditions and facilitate placental vascularization and signaling for trophoblast differentiation.116 HIFs are activated by both hypoxia and nonhypoxic factors such as the renin-angiotensin system, GFs, and cytokines.116,119 For example, HIF-1 regulation via angiotensin II increases extravillous trophoblast growth, cellular proliferation, and soluble fms-like receptor-1. Alterations are seen in the pathophysiology of preeclampsia (see Chapter 9). HIF-1 and TGFβ inhibit trophoblast invasion, while HIF-1 and insulin-like growth factor-2 increase trophoblast growth.116,119
Early flow of maternal blood into the IVS has been reported to be primarily in the periphery of the developing placenta.70 Jauniaux and colleagues postulate that higher oxygen flow in the periphery may induce regression of villi and formation of the chorion laeve (chorion).22,70 Increased flow in the central area of the developing placenta may increase the risk of early pregnancy loss due to oxidative damage to the trophoblast.70 By 11 to 12 weeks the spiral arteries become patent and maternal blood flow into the IVS and fetal oxygen levels increase. Vascular changes in the uteroplacental vessels are described further in “Placental Circulation.”
Development of the villi.
The placenta consists of the outer epithelial layer, derived from trophoblast cells, and an inner vascular network and connective tissue stroma, derived from embryonic mesoderm. Initially the lacunae are separated by trabecular columns of syncytiotrophoblast (primary villous stems), which provide the framework for development of the placental chorionic villi. The cytotrophoblast differentiates into vascular cytotrophoblast, which fuses to form chorionic villi and the extravascular invasive trophoblast, which is involved in remodeling of the spiral arteries (see “Placental Circulation”).78 Chorionic villi begin to appear toward the end of the second week of gestation as proliferation of the cytotrophoblast layer produces columns of cells or fingerlike processes known as primary chorionic villi.78 A mesenchymal core grows within these primary villi, forming secondary villi. Blood vessels within the villi arise from this mesenchymal core within a few days, forming tertiary villi.78,84
As the columns of cytotrophoblast cells proliferate, they extend through the syncytiotrophoblast, expanding laterally to meet and fuse with adjoining cytotrophoblast columns. This forms the cytotrophoblastic shell and divides the syncytiotrophoblast into an inner layer and a peripheral layer. The peripheral layer degenerates and is replaced by fibrinoid material.50,84 Villous development is stimulated by growth factors such as vascular endothelium growth factor (VEGF) and placental-like growth factor and by the relatively hypoxic environment. VEGF is found in maternal plasma by 6 weeks’ gestation and peaks at the end of the first trimester, similar to the pattern seen with hCG. During the third trimester, placental growth factors enhances formation of terminal villi.78 The low-oxygen environment stimulates angiogenesis, trophoblast formation, and increased numbers of highly vascularized terminal villi.78
The cytotrophoblastic shell is the point of contact between the fetal tissue and maternal tissue; it attaches the chorionic sac to the basal plate. The basal plate is formed by the compact and spongy zones of the maternal decidua basalis, remnants of the trophoblast, and fibrinoid material. By the end of the fourth month, the shell has regressed, with replacement of the cytotrophoblast cell columns by fibrinoid material (Rohr layer) and formation of clumps (islands) of cytotrophoblast cells.50 A layer of fibrinoid material (Nitabuch layer) also develops within the spongy zone of the decidua basalis. This is the level of placental separation at delivery (Figure 3-10).
Villi containing blood vessels (tertiary villi) arise around 20 days postconception.51,164 By 21 to 22 days, a primitive fetoplacental circulation is established between blood in the vessels of the villi, vessels forming in the embryo, primitive heart, and blood islands in the yolk sac (see Chapters 8 and 9). The villi that arise from the chorionic plate and attach to the maternal decidua basalis are known as anchoring (or stem) villi. Initially embryonic and fetal blood vessels develop within stem villi by branching angiogenesis.76,78 Stem villi, which contain arteries and veins as well as some arterioles and venules, make up about one third of the villi in the mature placenta.51 Mature intermediate villi grow from the sides of stem villi and project into the IVS (see Figure 3-10). These intermediate villi, which constitute approximately 25% of the villi in the mature placenta, contain primarily fetal capillaries with a few small arterioles and venules.50,51 Intermediate villi and their branches (terminal villi), constitute the major area of exchange between maternal and fetal circulations. After fetal viability, terminal villi are formed by nonbranching angiogenesis in mature intermediate villi.76 Terminal villi contain multiple dilated capillaries or sinusoids and account for 30% to 40% of the mature villous tree.51 Terminal villi bulge into the villous cytotrophoblast so that maternal and fetal blood are separated only by a thin syncytiotrophoblast layer.78 Alterations in development of the villous system may lead to miscarriage or fetal growth restriction.164
Anchoring villi grow more slowly than other portions of the placenta. As a result, during the third month, folds of the basal plate are pulled up into the IVS (see Figure 3-10). These folds (known as the placental septa) do not extend to the chorionic plate and have no known morphologic or physiologic function.50,84
By 40 to 50 days after ovulation, the trophoblast has invaded far enough into the endometrium to reach and begin to erode maternal spiral arterioles. Trophoblast plugs fill the tops of the spiral arteries until around 10 to 12 weeks’ gestation, when the arteries open up and begin to supply blood to the IVS.4 This is the time when the mature placenta is established. The chorion laeve fuses onto the decidua vera, forming the chorion or outer fetal membrane. The inner membrane, the amnion, is derived from the amniogenic cells (amnioblasts) of the cytotrophoblast (see “Development of the Amniotic Cavity”).
Placental growth
By 4 months, the placenta has achieved its full thickness, with no new lobules or stem villi added after 10 to 12 weeks. Circumferential placental growth continues with further ramification of stem villi (via growth and extension of new trophoblast sprouts, followed by growth of the mesenchymal core and development of blood vessels), lengthening of existing villi, and increases in the size and number of placental capillaries.33,103 Much of the expansion of villi after 20 weeks is in the terminal villi. Mature intermediate villi elongate in the third trimester, which assists in generation of new terminal villi branches.99 As a result of the proliferation of terminal villi, the surface area for placental exchange continues to increase until late in gestation. In addition, the thickness of the tissue layers separating maternal and fetal blood thins, thus enhancing diffusion. Trophoblast sprouts not used to form new villi break off, enter the maternal circulation, lodge in the mother’s lung capillaries, and are cleared by proteolysis.
After 30 weeks’ gestation, syncytial knots develop, pulling the syncytium and its nuclei into “piles” several layers thick and leaving a thin, attenuated, anuclear membrane in the intervening areas.103 These areas (known as the vasculosyncytial membrane) appear to, but do not actually, fuse with dilated fetal capillaries and are thought to be specialized regions that facilitate placental gas exchange.50,84
Increases in villous surface area and thinning of placental tissue layers increase the functional efficiency of the placenta during pregnancy. During the first 2 months, the relatively few villi are greater than 170 μm in diameter, are vascularized by a small central fetal vessel, and are covered by two layers of trophoblast (syncytiotrophoblast outer layer and inner layer of cytotrophoblast) of uniform thickness. At this stage, the villous stroma consists of a loose network of primitive mesenchymal tissue and many Hofbauer cells (tissue macrophages).50 From 8 to 30 weeks, the number of villi increase and the average diameter decreases to about 70 μm. The stroma becomes thinner and more compact and contains fibroblasts and collagen fibers with fewer Hofbauer cells.50 By term the placental villi are approximately 35 to 40 μm in diameter. The trophoblast layer and stroma have thinned considerably, and few cytotrophoblast cells are seen.51
The area-to-volume ratio of the placenta progressively increases. Surface area increases from 3.4 m2 (28 weeks) to 12.6 m2 (term), and the syncytium decreases in thickness from 10 mm2 to 1.7 mm2 late in gestation. The actual surface area is closer to 90 m2 because of the presence of extensive microvilli on the surface of the syncytiotrophoblast covering the villi.33,46 Transfer efficiency of placental tissue increases sixfold. The trophoblast layer and connective tissue thin so that the capillaries lie closer to the syncytial trophoblast, decreasing the distance that nutrients and waste products have to travel.
By term the placenta weighs approximately 480 g (±135 g) with a diameter of 18 to 22 cm and thickness of 2 to 2.5 cm, although considerable variation is seen.134 Although villous growth continues to near term, the placenta begins to undergo degenerative changes. These changes include increased syncytial knots, intervillous thrombi, fibrin deposits, infarcts, and calcifications.
Abnormal growth of the placenta may also occur. Hypoplasia of the syncytiotrophoblast can alter implantation and result in abortion, abruptio placentae, or poor development of terminal villi and increase the risk of stillbirth or preterm delivery. Hyperplasia and reactivation of the syncytial growth layer, possibly due to suboptimal oxygenation, have been observed in women with hypertension and prolonged pregnancy. Although the amount of cytotrophoblast decreases with gestation, the remaining tissue retains its proliferative capacity so the cytotrophoblast can be reactivated to replace damaged or destroyed syncytiotrophoblast.50 However, this hyperplasia may be followed by degenerative changes.33
Placental structure
Although the mature placenta contains maternal and fetal components, it is primarily a fetal structure composed of extensively branching, closely packed fetal villi containing fetal blood vessels (see Figure 3-10). Three main types of chorionic villi can be identified: (1) stem (or anchoring) villi, which consist of multiple branches and function to stabilize the villous tree; (2) mature intermediate villi, located between the stem and terminal villi; and (3) terminal villi, which are the major areas of maternal-fetal exchange.84 On the fetal side, the placenta is covered by the chorionic plate, a thin membranous structure continuous with the fetal membranes. On the maternal side, the outer layer of trophoblast cells fuse to the decidua basalis.
The fetal portion of the placenta is divided into 50 to 60 lobes, each arising as a primary stem villus supplied by primary branches of the umbilical vessels from the chorionic plate. Each lobe is divided into one to five subunits or lobules. Lobules are globular structures with a central cylindrical space that is relatively empty.50 This space may arise because of preferential growth of villi in relation to entry of maternal arteries into the IVS. Beneath the chorionic plate the primary stem villi divide into secondary stem villi. These villi run parallel to the chorionic plate, dividing into tertiary stem villi, which project downward through the parenchyma of the placenta around the central space and anchor onto the basal plate (see Figure 3-10).50 Each primary stem villus may give rise to varying numbers of secondary stem villi and lobules.50 The terminal villi branch off of the tertiary stem villi.
The placental septa divide the maternal surface of the placenta into 10 to 40 (average 15 to 20) lobes, each containing at least one and usually more main stem villi and their branches.33,51 The term cotyledon is sometimes used to describe these lobes. Fox suggests that, because the maternal lobes are just areas between placental septa with no physiologic or morphologic significance, cotyledon should really be used to describe portions of the villus tree that arise from a single primary stem villus.50
Maternal and fetal circulations are separated by several layers of tissue. These tissues are called the placental membrane or placental barrier. A substance, such as glucose, moving from the maternal to the fetal circulation must initially pass from maternal blood through five layers to reach the fetal blood: (1) the microvillous membrane of the syncytiotrophoblast, (2) the syncytiotrophoblast cells, (3) the basal membrane of the syncytiotrophoblast, (4) the connective tissue mesenchyme of the villus, and (5) the epithelium of the fetal blood vessel (Figure 3-11). The cytotrophoblast ceases to form a continuous layer after about 12 weeks and is replaced by a fibrinoid layer.103 As gestation continues, the connective tissue thins, and the fetal capillaries increase in number and size.
Umbilical cord
The umbilical cord can be seen on ultrasound by 42 days and is well established by 8 to 9 weeks.97 It normally contains two arteries and one vein surrounded by Wharton jelly, a substance containing collagen, muscle, and mucopolysaccharide. The cord epithelium is formed by amnion. No other blood vessels are found in the cord; neural tissue is also absent. Vasoresponsiveness of the cord to stimuli or drugs is thought to be myogenic.84
The umbilical vessels are longer than the cord and tend to twist and spiral within the cord. The umbilical arteries contain four muscle layers: an inner circular layer, a longitudinal layer, and two helical layers. The helical layers function independently to coil both the arteries and the umbilical cord. The twist or spiral of the cord is established by 9 weeks’ gestation. A left (counterclockwise) twist is more common than a right (clockwise) twist; however, bidirectional twisting is sometimes seen. The direction of the twist is not significant.134 Coiling is thought to protect the cord against tension, compression, and entanglement. Up to 30% of cords may be uncoiled at 20 weeks, but less than 5% by term.97 Lack of coiling has been associated with fetal growth restriction, oligohydramnios, fetal anomalies, and preterm delivery.97
The cord arises from fusion of the connecting stalk with the yolk sac stalk and allantois at the end of the fourth week of gestation.103 The umbilical vessels arise from the allantois and initially consist of four vessels. One of the two original umbilical veins invariably atrophies by 6 weeks after fertilization, followed by dilation of the remaining (left) vessel. Occasionally one umbilical artery, usually the left, also regresses sometimes or does not form, resulting in a two-vessel cord, which may sometimes be associated with other anomalies (see “Abnormalities of the Cord and Placenta”).97
The cord reaches its maximal length by 30 weeks and averages 55 to 60 cm (range, 30 to 90 cm) with a width of 1 to 2 cm (thicker in large for gestational age infants).47,97,134 Cord length is determined both genetically and by intrauterine space and fetal activity, which places tension on the cord.47,97 The minimum cord length for vaginal delivery is thought to be 32 cm.134 Occasionally the arteries may fuse near the placenta, so observation of the number of placental vessels should occur at least 3 cm from the placenta.134
The umbilical vessels constrict soon after delivery. Constriction of the umbilical arteries begins within 5 seconds of birth and is complete by 45 seconds; the umbilical vein begins to constrict within 15 seconds of delivery and is functionally closed by 3 to 4 minutes.84 Placental transfusion and issues regarding timing of cord clamping are discussed in Chapter 8.
Amnion and chorion
The amnion and chorion begin to develop soon after fertilization and continue to grow until about 28 weeks’ gestation. After this point, further mitotic activity is rare, and enlargement takes place by stretching of the existing membranes.84 The layers of the fetal membranes at term are: the innermost amniotic epithelium (involved in amniotic fluid exchange and pH regulation), lamina propria, chorionic connective tissue, cytotrophoblastic layer (which along with the chorionic connective tissue becomes the chorionic basal plate at the placental margin), and decidual layer.51 By 7 to 10 weeks, the amnion and chorion are in contact with each other, and their mesenchymal layers may fuse in places.51
The amnion and chorion are connected by an extracellular matrix (fibrous proteins embedded in polysaccharide gelatinous matter). The chorion adheres to the decidua. The chorion is relatively fixed. The amnion is passively pushed against and moves over the chorion aided by mucus from the spongy layer. As a result, the amnion can rupture, forming shreds of tissue (amniotic bands), while the chorion remains intact. The amniotic bands may wrap around, constrict, or amputate fetal parts. The reason for rupture of the amnion is unknown but may be related to trauma.84
The fetal membranes are metabolically active and are involved in amniotic fluid turnover (see “Amniotic Fluid Volume and Turnover”) and initiation of labor (see Chapter 4). The fetal membranes are a major site of prostaglandin (PG) synthesis and metabolism. During most of pregnancy many PGs are converted to inactive metabolites due to a balance between prostaglandin synthetase (need for PG synthesis) and 15-hydroxyprostaglandin dehydrogenase (PGDH; needed for PG inactivation). However near term, PG synthesis increases and PGDH decreases in preparation for labor onset.107 The amnion is a reservoir for storage of arachidonic acid, an essential prostaglandin precursor, and the chorion is a reservoir for progesterone.84 The membranes and decidua are rich sources of enzymes (e.g., phospholipase A2) needed for formation of prostaglandins. Cells of the amnion are involved in protein synthesis; protein and lipid secretion; and exchange of water, electrolytes, and other solutes. The amnion has a metabolic rate similar to that of the liver. The chorion synthesizes substances such as renin and prostaglandins. 11-β-hydroxysteroid dehydrogenase type 1 (11β-HSD1) is also found in fetal membranes. 11β-HSD1 converts inactive cortisone to active cortisol. Fetal membrane 11β-HSD1 contributes to the increase in glucocorticoid concentrations in the third trimester needed for fetal organ maturation and initiation of labor (see Chapter 4).107
The ability of the membranes to stretch and resist rupture from increasing fetal size and amniotic fluid volume until the end of pregnancy is thought to be related to the collagen-rich tissue of the amnion, which has great tensile strength that is maintained throughout gestation. With delivery, the decidua and chorion must separate to allow expulsion of the membranes. This process involves fetal fibronectin, an extracellular matrix protein found at the decidual-chorionic interface.107 Rupture of the membranes is discussed in Chapter 4. Premature rupture of the membranes (PROM), or rupture before onset of uterine contractions, may be due to factors such as mechanical stress (polyhydramnios, multiple gestation), alterations in membranous collagen, or chorioamnionitis. In chorioamnionitis a variety of vaginal and cervical microorganisms have been demonstrated to produce proteases that alter membrane integrity and reduce the pressure needed for rupture. PROM may be mediated by collagenetic enzymes from the placenta and amniotic fluid that increase in activity with increasing gestational age.
Placental circulation
Adequate blood flow to and through the placenta from both fetal and maternal circulations is essential for sufficient exchange of nutrients, gases, and waste products. However, nearly half of the combined maternal-fetal blood flow to the placenta is not involved in maternal-fetal transfer due to maternal and fetal shunts within the uteroplacental circulation.46 The fetal shunt is the portion of umbilical blood flow that does not supply the area of exchange between maternal and fetal blood. Approximately 17% to 25% of the total umbilical blood flow is used to supply the fetal membranes and placental tissue. Maternal shunt is the portion of uterine blood flow that does not supply the IVS. Approximately 20% to 27% of uterine arterial blood supplies the myometrium and cervix. At term the uterus extracts 30 to 35 mL of oxygen per minute from maternal blood.50
Fetal-placental circulation
The rate of fetal blood flow in the placenta is about 500 mL/min.50 Blood flow is dependent on fetal heart activity and regulated by the interaction of blood pressure, fetal right-to-left shunts, and systemic and pulmonary vascular resistance.46 Contraction of smooth muscle fibers in stem villi may help pump blood from the placenta back to the fetus. The placenta is a low-resistance circuit in the fetal circulatory system (see Chapter 9) as a result of minimal umbilical innervation.
Maternal uteroplacental circulation
Blood flows to the uterus via the uterine arteries, which are branches of the internal iliac and ovarian arteries from the abdominal aorta, and then into the uterine spiral arteries (see Figure 2-28). The proportion of the maternal cardiac output supplying the uterus and IVS increases during pregnancy, peaking at 10% to 20%.102 The increased blood flow is mediated by the low-resistance uteroplacental circuit, alterations in maternal cardiac output and systemic and peripheral vascular resistance, and hormonal and chemical influences (see Chapter 9). Blood reaches the placenta via the altered spiral arteries of the uterus. The spiral arteries are also altered during the menstrual cycle, elongating in the proliferative phase and becoming coiled (spiraled) during the secretory phase.76 However, more extensive changes occur during pregnancy along with destruction of muscular and elastic elements of the walls of these arteries.
Maternal blood enters the IVS via uteroplacental arteries (altered spiral arteries) in the endometrium. By term, blood in these spaces is supplied by 100 to 200 (decreasing to 50 to 100 by term) maternal uteroplacental arteries and removed by 50 to 200 veins.51 Blood flow enters the IVS through arterial inlets near the center of a lobule as a funnel-shaped stream flowing toward the chorionic plate or “roof” of the placenta (see Figure 3-10).103 The blood then flows radially around the villi, allowing exchange of materials between maternal and fetal circulation. Blood leaves the IVS via wide venous outlets at the periphery of villous trees and flows into the uteroplacental veins and subchorial, interlobular, and marginal venous lakes.51
Overall the pressures within this system are low due to anatomic changes in maternal uterine blood vessels. As a result, the maternal arterial blood pressure is not transmitted to the IVS, and pressure gradients from the arterial to venous sides are relatively small (i.e., pressure averages 25 mmHg [3.25 kPa] in the uteroplacental arteries, 15 to 20 mmHg [1.99 to 2.66 kPa] in the IVS, and 5 to 10 mmHg [0.66 to 1.33 kPa] in the uterine veins).50,84
The IVS in the mature placenta contains about 150 mL of blood, which is completely replenished three to four times a minute. The rate of blood flow within the maternal side of the placenta increases during pregnancy from 50 mL/min at 10 weeks to 500 to 600 mL/min by term.33,46 Uterine contractions limit the entry of blood into the IVS but do not squeeze out a significant amount of blood. Thus oxygen transfer to the fetus may be decreased during a normal contraction but does not cease, because transfer continues from blood remaining in the IVS.
The arteries of the endometrium (decidua) and myometrium undergo marked physiologic changes during pregnancy that convert the uterine spiral arteries into uteroplacental arteries. These changes are mediated by extravillous invasive trophoblast that invades the spiral arteries in the decidua and upper third of the myometrium. Interstitial extravillous trophoblast migrates into the decidua and surrounds the spiral arteries, destroying their muscular walls and elastic elements, causing them to swell. A subtype of extravillous trophoblast, endovascular trophoblast, migrates into the luminal walls replacing the spiral artery endothelium (Figure 3-12).23,42,50,63,146 Trophoblast invasion involves a combination of migratory and proteolytic activities mediated by matrix metalloproteinases, serine proteases, tissue plasminogen activator, and urokinase.51,63 Much of the arterial wall is replaced by a fibrinoid extracellular matrix that arises from maternal fibrin and from proteins secreted by the trophoblast so that the smooth muscle of the wall and elastic lamina are lost.19,23,76,113
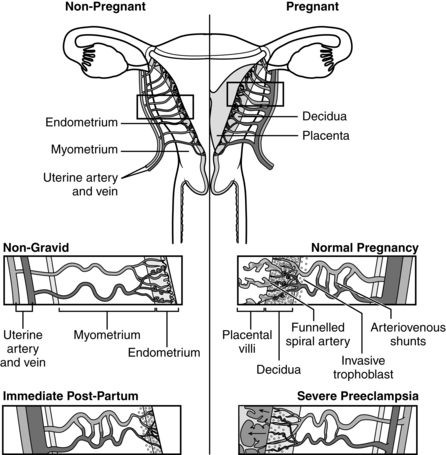
These alterations change the vessels from narrow to large caliber, widely dilated vessels, enhancing the capacity of the uteroplacental vessels to accommodate the increased blood volume needed to supply the placenta.76 The vessels become funnel shaped with a four-fold increase in diameter.23 The terminal coils of the arteries may reach 2 to 3 mm in diameter.23 These vessels are also functionally denervated due to decreased neurotransmitter sites. As a result, the spiral arteries (now called uteroplacental arteries) underlying the placenta are almost completely dilated and become distended, flaccid, saclike structures with low resistance, able to accommodate the blood needed to supply and provide a reservoir for the IVS.146 These arteries are no longer responsive to systemic circulatory pressor agents or influences of the autonomic nervous system.47 Control of the uteroplacental circulation is at the level of the radial arteries and is mediated primarily by local (uteroplacental) influences, including placental production of prostacyclin (PGI2). PGI2, the most potent vasodilator produced by the placenta, is thought to maintain vasodilation of these vessels, prevent platelet aggregation, and enhance cell disengagement (needed for disruption of elastic and muscular elements).
As the trophoblast migrates into the decidua, it acquires an endothelial cell adhesion molecule phenotype, switching from a proliferative function to an invasive function. This switch is controlled by down- and up-regulation of specific genes and gene products, including integrins, and mediated by trophoblast and decidual factors, such as growth factors, enzymes, and binding proteins.63,65 Proinvasive factors include trophoblast proteases and decidual activators and attractants; antinvasive factors include decidual barrier properties and local inhibitors. If the balance between proinvasive and inhibiting factors is altered, abnormally decreased or increased invasion occurs.79 The depth of the invasion is limited because extravillous trophoblast can only proliferate while in contact with anchoring villi; once these cells migrate out they can only invade for the lifespan of the migrating cell.51 In addition, the extravillous trophoblast is thought to be under the influence of a paracrine signal, probably IGF-1, secreted by the villous mesenchyme. As the extravillous trophoblast migrates farther away, the paracrine signal and thus trophoblast invasiveness diminishes.83 Altered invasion is associated with miscarriage, fetal or intrauterine growth restriction (IUGR), preeclampsia (see Figure 3-12), preterm labor with intact membranes, preterm rupture of membranes, spontaneous abortion, and placental abruption.18,23,63,127,155 Excessive blood flow to the IVS in the first trimester may result in an oxidative insult to the developing embryo and decreased angiogenesis in the placenta.70
The invasive trophoblast not only remodels the spiral arteries but also temporarily occludes the tips of the spiral arteries, limiting maternal blood from entering the IVS in significant amounts until 10 to 12 weeks’ gestation (see Placentation).22,23,68,70,79,124 The trophoblast plugs, seen particularly in the central areas, probably allow seepage of plasma through intracellular clefts.22 These changes reduce placental oxygen concentration until about 10 weeks, when concentrations increase rapidly. At the same time, as maternal blood begins to flow more readily into the IVS, fetal antioxidant systems increase.70 Thus first trimester placental circulation is very different from that in the second and third trimesters.68 The low oxygen environment in the first trimester may protect the developing embryo, who has not yet developed protective mechanisms against oxidative stress.68,70 Failure of conversion of the spiral arteries results in maternal blood entering the IVS at a greater velocity. This can lead to morphologic changes in the placenta including reduction in anchoring villi, impaired maternal-fetal oxygen exchange, and increased intermittent perfusion of the IVS leading to placental oxidative stress.23
The extravillous invasive trophoblast migrates into the maternal tissue in two phases. During the initial phase from about 6 to 10 or 12 weeks, spiral arteries in the decidua are altered; during the second phase, beginning at 14 to 16 weeks and lasting for 4 to 6 weeks (generally completed by 20 to 22 weeks), spiral arteries in the inner one third of the myometrium are altered.19,50,65,76,84,146 Only spiral arteries underlying the placental bed are modified. This process is controlled by the interaction of ovarian and placental hormones, local cytokines, immune cells, and vascular growth factors.76
The importance of these changes for fetal survival and growth can be appreciated by examining situations such as spontaneous abortion, preeclampsia, and IUGR in which invasion of the spiral arteries by invasive cytotrophoblast does not occur or is abnormal.18,23,63,65,68 Early pregnancy loss is often associated with defective placentation (thin, fragmented trophoblast shell and decreased cytotrophoblast invasion at the ends of the spiral arteries). Failure of plugging of these arteries exposes the placenta and embryo to intermittent oxidative stress that may increase the risk of pregnancy complications.22,23,106 Recurrent spontaneous abortion in some women may be due to absent or inadequate conversion of spiral arteries to uteroplacental arteries.22 Absence of changes in the decidual portion of the spiral arteries has been associated with late first trimester loss; absence of changes in the myometrial portion has been associated with second trimester loss.118 Failure of the normal cytotrophoblastic invasion of the decidua and myometrium and subsequent arterial changes occurs in some types of fetal growth restriction. Underperfusion of the IVS may lead to occlusion of small arterioles within the villi. These changes can markedly alter fetal growth and health status and are similar to changes reported with some growth-restricted fetuses.
Preeclampsia is associated with alterations in the normal invasion of the spiral arteries by the invasive trophoblast (see Figure 3-12).76,79,105 This defect, which may have a genetic basis, is postulated to be an initial event in the development of preeclampsia. Generally the first invasive trophoblast phase proceeds normally, but there is failure of the second phase.50,84 Thus decidual spiral arteries undergo the usual physiologic changes, but the myometrial arteries do not. The uninvaded arterial segments may also develop atherosclerosis with necrosis and invasion of the damaged wall by fibrin and other substances. Alterations in the normal changes within the uterine arteries in women with preeclampsia lead to (1) decreased perfusion of the IVS due to the retained muscular coat and inability of the vessels to dilate sufficiently to accommodate the increased blood flow, and (2) hypertension in the uteroplacental arteries due to continued sensitivity of these vessels to circulatory pressor agents and the influences of the autonomic nervous system.84,118
The basis for this defect in preeclampsia is unclear. It may be a primary defect in invasive trophoblast, alterations in endometrial metabolism, altered endometrial immunologic reactions, or due to an altered environment.79 Preeclampsia has been associated with abnormal expression of integrin molecules limiting trophoblast invasion into the decidua, resulting in decreased uteroplacental blood flow.65 If the spiral arteries are not converted into low-resistance circuits, the placenta secretes vasoactive substances that increase maternal blood pressure.79 Alterations in production of placental PGI2 and thromboxane (TXA2) occur with preeclampsia. Prostacyclin (PGI2) is produced in many tissues and is a potent vasodilator and inhibitor of platelet aggregation and uterine contractility, which acts to promote increased uteroplacental blood flow during pregnancy. Conversely, TXA2 is a potent vasoconstrictor that opposes the action of prostacyclin. An imbalance in levels of these mediators produced by the placenta, with a relative elevated TXA2 and decreased prostacyclin, may alter the usual changes in the uterine blood vessels.
Placental function
The placenta has four major activities: metabolic, endocrine, immunologic, and transport. The placenta also serves as a “radiator,” with 85% of fetal heat production transmitted to the mother via the placenta.133
Placental metabolism
Placental metabolism contributes to the quality and quantity of the fetal nutrient supply. Placental metabolic functions are particularly important early in pregnancy in providing nutrition and energy for the developing fetus and for the placenta itself. The placenta has a high metabolic rate. Rates of oxygen consumption and glucose utilization by the placenta approximate those of the brain.78 Glucose use by the placenta equals 50% to 70% of the uterine glucose uptake.
The placenta is an active synthesizer of glycogen, fatty acids, cholesterol, and enzymes. The placenta produces protective and enhancement enzymes such as sulfatase, which enhances the excretion of fetal estrogen precursors, and insulinase, which increases the barrier to the transfer of insulin.46 Relatively large amounts of ammonia and lactate are produced by the placenta and may be important in stimulating metabolic activity of the fetal liver. The impact of alterations in these metabolic processes on placental function and transport during pathologic states is not clear, but they decrease the capacity of the fetus to tolerate labor and transition to extrauterine life. Placental metabolic functions are described further in Chapter 16.
Placental endocrinology
Placental endocrine activities are important in maintaining pregnancy and inducing metabolic adaptations in the mother and fetus. The placenta synthesizes polypeptide hormones (such as hCG and human placental lactogen [hPL]) and steroid hormones (estrogens and progesterone) as well as mediators such as pregnancy-associated plasma proteins (PAPP), neurohormones and neuropolypeptides, binding proteins, cytokines, and growth factors (Table 3-2). Growth factor receptors appear on the trophoblast early in gestation. Growth factors are short polypeptides that are produced in many different types of tissues. Growth factors act in paracrine and autocrine manner on specific localized target tissues by interacting with receptors on the target cell’s membrane. This stimulates, via second messengers, signal transduction within the cell, initiating specific changes within the cell such as glucose uptake, RNA and protein synthesis, amino acid transport, and DNA synthesis and cell replication.87
Table 3-2
Examples of Growth Factors, Neuropeptides, and Proteins Identified in Placental Tissues
PROTEIN/PEPTIDE HORMONE | NEUROHORMONE/ NEUROPEPTIDE | GROWTH FACTOR | BINDING PROTEIN | CYTOKINE |
From: Liu, J.H. (2009). Endocrinology of pregnancy. In R.K. Creasy, et al. (Eds.). Creasy & Resnik’s Maternal-fetal medicine: Principles and practice (6th ed.). Philadelphia: Saunders Elsevier, p.117.
Four major hormones synthesized by the placenta are hCG, hPL, progesterone, and estrogens. The placenta also produces pituitary-like and gonad-like peptide hormones (i.e., placental corticotropin, human chorionic thyrotropin, melanocyte stimulating hormone, β-endorphin, β-lipoprotein), hypothalamus-like releasing hormones (i.e., human chorionic somatostatin, CRH), and gut hormones (i.e., gastrin, vasoactive intestinal peptide). The placenta, membranes, and fetus also synthesize a variety of peptide growth factors including EGF, nerve growth factor (NGF), platelet-derived growth factor (PDGF), skeletal growth factor, and IGF-1 and IGF-2 (see Table 3-2).45,87
Placental growth factors regulate cell growth and differentiation, hormone release at the local level, and uterine contractility.87 For example, IGF-1 and IGF-2 regulate cell proliferation and differentiation to maintain normal fetal growth. IGF works by enhancing amino acid and glucose uptake and preventing protein breakdown. Transforming growth factor–α (TGF-α) and EGF have primary roles in regulating cell differentiation.87 TGF-β has both proliferative and antiproliferative actions to stimulate and inhibit cell differentiation. This factor is also involved in embryogenesis and neural migration and differentiation. Activin stimulates and inhibin decreases placental hCG and progesterone. Follistatin inhibits follicle-stimulating hormone (FSH) release.87 Human placental growth hormone (GH-V) regulates IGF-1.
Until 15 to 20 weeks, maternal pituitary GH (GH-N) is the major maternal growth hormone; after that time, levels of GH-V increase and maternal GH-N is supressed.45,87 GH-V may alter maternal metabolism by stimulating gluconeogenesis and lipolysis in the second half of pregnancy to increase nutrient availability for the fetus (see Chapter 16).45,87 Parathyroid hormone–related protein (PTHrP) mediates placental calcium transport (see Chapter 17). PTHrP also has a role in development of the bone and epithelial organs and in the interaction of epithelial and mesenchymal cells.141 Leptin is involved in regulating energy homeostasis, weight, and reproductive processes in pregnancy and may play a role in regulating fetal growth and development and possibly in placental development, angiogenesis, and hematopoiesis.45,96 In addition to the hypothalamus, corticotropin-releasing hormone (CRH) is synthesized by the placenta (the major source of CRH during pregnancy), amnion, chorion, and decidua and stimulates release of prostaglandins. CRH has a major role in initiation of myometrial contractility and labor onset (see Chapter 4).
The placenta also produces cytokines that help regulate placental function (see Table 3-2). Cytokines are regulatory peptides or glycoproteins produced by most nucleated cells that regulate cell function via paracrine (intercellular) or autocrine (intracellular) signals. For example, interleukins induce syncytiotrophoblast release of hCG that in turn stimulates release of progesterone from the corpus luteum. Other cytokines and peptides produced by the placenta are involved in immunoregulation (see Chapter 13).
Human chorionic gonadotropin.
Human chorionic gonadotropin (hCG) is a heterodimer glycoprotein with α and β subunits that is biologically similar to LH, FSH, and thyroid stimulating hormone. In early pregnancy there are more β subunits found, and then similar numbers of each type are seen; after 22 weeks there are more α than β subunits.30 Measurement of free β subunits is used in first and second trimester maternal serum screening (see “Assessment of the Embryo and Fetus”). The major function of hCG is to maintain the corpus luteum during early pregnancy in order to ensure secretion of progesterone and other substances until placental production is adequate.87 In addition, hCG may stimulate the fetal testes and adrenal gland to enhance testosterone and corticosteroid secretion, stimulate production of placental progesterone, promote angiogenesis, uterine growth and quiescence, enhance fetal growth and development, and suppress maternal lymphocyte responses to prevent rejection of the placenta by the mother.30,114 hCG may also have a role in preventing cervical ripening during pregnancy.75 Release of hCG is enhanced by gonadotropin-releasing hormone (GnRH), IL-1, IL-2, IGF, EGF, and activin and inhibited by inhibin, opioids, and TGF-β.117 hCG is produced primarily by syncytiotrophoblast cells in the placenta, although small amounts may be produced by other types of trophoblast tissue.50 Several variants of hCG are produced, including hyperglycosalated hCG (hCG-H). hCG-h is produced by the extravillous trophoblast and promotes implantation and cytotrophoblast growth.30
hCG can be detected in maternal serum and urine 7 to 8 days after ovulation, or around the time of implantation and has been found in the preimplantation blastocyst.75,87 hCG is used as the basis of pregnancy tests using maternal urine. Generally, enough hCG has been produced to give a positive indication of pregnancy by 3 weeks after conception (7 to 8 days before the expected menses).87 Concentrations of hCG in maternal serum double every 2 to 3 days until peak values (100,000 mU/mL) are reached 60 to 90 days after conception (Figure 3-13).75,87 Concentrations of hCG decrease after 10 to 11 weeks and reach a plateau at low levels at 18 to 20 weeks.43 By 2 weeks after delivery, hCG disappears. Persistently low levels may indicate an abnormal placenta or ectopic pregnancy; levels remain elevated in women with hydatidiform moles.33 Higher levels are also seen with multiple gestations.131
Human placental lactogen.
hPL, also called human chorionic somatomammotropin (hCS) or chorionic growth hormone, consists of a single polypeptide chain similar in structure to human growth hormone and prolactin. hPL promotes fetal growth by altering maternal protein, carbohydrate, and fat metabolism (see Chapter 16). The primary role of hPL is regulating glucose availability for the fetus. hPL is an insulin antagonist that increases maternal metabolism and use of fat as an energy substrate and reduces glucose uptake and use by maternal cells. As a result, more glucose is available for transport to the fetus.50 Thus hPL acts as a growth-promoting hormone, promoting fetal growth by altering maternal metabolism. The functions and regulation of hPL are not completely understood.87
Production of hPL by the syncytiotrophoblast begins 12 to 18 days after conception (5 to 10 days after implantation), rises during pregnancy, and peaks near term. hPL is the most abundant placental secretory product.87,131 Secretion of hPL is regulated by glucose; decreased serum glucose leads to increased hPL secretion and increased maternal lipolysis. As early as 4 weeks after conception, hPL can be detected in maternal serum; little is found in maternal urine. hPL has a short half-life, so maternal serum levels reflect the rate of production.
Steroidogenesis.
Progesterone and the estrogens are steroid hormones whose placental synthesis increases during pregnancy (see Figure 3-13). Early in gestation, the corpus luteum is the main synthesis site, but by 6 to 8 weeks the placental syncytiotrophoblast has taken over as the major producer.74 Steroidogenesis during pregnancy is based on a complex set of interactions by systems in the mother, fetus, and placenta. Each system lacks essential enzymes necessary for creation of the final hormone. Placental production of progesterone and estrogens is an example of a function that requires cooperative efforts of the mother, placenta, and fetus. The main source of cholesterol for steroidogenesis is maternal low-density lipoproteins.74 The placenta lacks certain enzymes needed for production of estriol; these enzymes are present in the fetal adrenal gland. The fetus is the major source of specific precursors for the estrogens, and the mother is the major source of precursors for the progesterone (Figure 3-14). Placental progesterone also serves as a precursor for fetal synthesis of corticosteroids, testosterone, and androgens.33,71,74
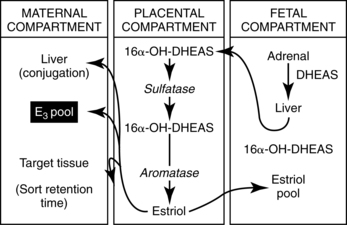
Progesterone.
Progesterone is produced by the corpus luteum (under the influence of hCG) during the first 6 to 10 weeks after fertilization.87 After that period, progesterone is synthesized primarily by the placenta using maternal cholesterol and low-density lipoproteins as precursors. A fetus is not essential for placental progesterone production, which continues even after fetal death. Progesterone production in late pregnancy is about 250 mg/day; 90% is secreted into maternal circulation.50,74 Active progesterone metabolites such as deoxycorticosterone (DOC) contribute to the altered response to the pressor action of angiotensin II during pregnancy (see Chapter 11).
During pregnancy, progesterone acts to decrease myometrial activity and irritability; constrict myometrial vessels; decrease sensitivity of the maternal respiratory center to carbon dioxide; inhibit prolactin secretion; help suppress maternal immunologic responses to fetal antigens, thereby preventing rejection of the fetus; relax smooth muscle in the gastrointestinal and urinary systems; increase basal body temperature; and increase sodium and chloride excretion.33
Estrogens.
The three major estrogens are estrone, estradiol, and estriol. During pregnancy, production of estrogens, particularly estriol, increases markedly. Estrone and estradiol production increases about 100 times; estriol production increases about 1000 times. At term, estriol production by the placenta is 35 to 45 mg/day.74 During pregnancy, estrogens act to enhance myometrial activity, promote myometrial vasodilation, increase sensitivity of the maternal respiratory center to carbon dioxide, soften fibers in the cervical collagen tissue, increase pituitary secretion of prolactin, increase serum binding proteins and fibrinogen, decrease plasma proteins, and increase the sensitivity of the uterus to progesterone in late pregnancy.33
Early in pregnancy, estriol is derived from estrone and estradiol. Production of estrogens, and particularly estriol, is dependent on interaction of the maternal-fetal-placental unit. Approximately 90% of the precursors for estriol are derived from the fetus; 40% of the precursors for estrone and estradiol come from the mother and 60% from the fetus.33,87 The primary source of estriol precursors (dehydroepiandrosterone sulfate [DHEAS]) is the fetal adrenal gland under stimulation by fetal adrenocorticotropic hormone (ACTH). DHEAS is hydroxylated in the fetal liver and further metabolized by the placenta to form estriol. By term the fetal adrenal is producing 100 to 200 mg/day of DHEAS.74 Estriol is secreted by the placenta into maternal circulation and eventually excreted in maternal urine. Maternal serum and urinary estriol levels rise rapidly during early pregnancy, more slowly between 24 and 32 weeks, and then increase rapidly again in the last 6 weeks. Although not currently used, estriol assays were one of the earlier methods of assessing fetal well-being and placental function for management of complicated pregnancies.33,93
Placental immunologic function
The immunologic functions of the placenta include protection of the fetus from pathogens and prevention from rejection by the mother. Many bacteria are too large to cross the placenta, although most viruses and some bacteria are able to cross. The placenta also allows passage of maternal antibodies of the immunoglobulin G (IgG) class primarily via pinocytosis, although some may cross by diffusion. This may also be a disadvantage, because both protective and potentially deleterious antibodies cross the placenta. The fetus differs in genetic makeup from the mother, yet it is not rejected. Possible explanations for this phenomenon and placental immunologic functions are discussed in Chapter 13.
Placental transport
Placental transfer or transport involves bidirectional movement of gases, nutrients, waste materials, drugs, and other substances across the placenta from maternal-to-fetal circulation or from fetal to maternal circulation (Figure 3-15). Transport across the placenta increases during the course of gestation due to changes in placental structure (decreasing distance between maternal and fetal blood), increased fetal and maternal blood flow, and greater fetal demands. Relative concentrations of substance in maternal versus fetal circulation may be higher in the fetus, higher in the mother or similar in mother and fetus. For example, amino acids, calcium, and phosphorus are higher in fetal than maternal plasma; total proteins, globulins, fibrinogen, phospholipids, glucose, and fatty acids are higher in maternal than fetal plasma, while concentrations of sodium, chloride, and urea are similar.72
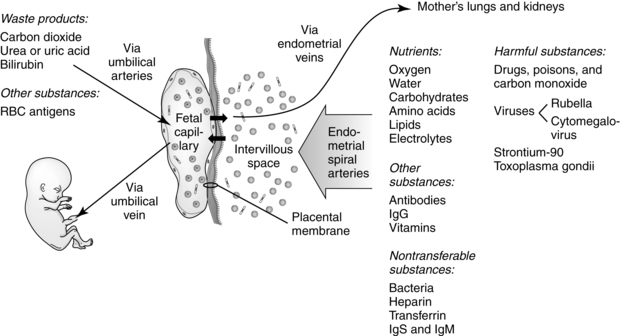
The syncytiotrophoblast is the site of transport with substances moving from the apical membrane (facing maternal blood in the IVS), across the cell wall and villous stoma to the basal membrane (facing the fetal capillary epithelium).92 The mechanisms by which substances are transferred across the placenta include simple (passive) diffusion, facilitated diffusion, active transport, pinocytosis, endocytosis, bulk flow, solvent drag, accidental capillary breaks, and independent movement. Facilitated diffusion and active transport are mediated by protein carriers and other transporters. Transporters are located on both the maternal-facing brush border syncytiotrophoblast (facing the IVS) and the fetal-facing basal membrane (facing the fetal villous stroma).54 Transporters include both nutrient (see Chapter 16) and drug (see Chapter 7) transporters.32,66,148,158 Transfer can be modified by maternal nutritional status; exercise; and disease, such as diabetes mellitus (glucose transport increases due to maternal hyperglycemia), hypertension (decreased nutrient transfer as a result of reduced uteroplacental blood flow), and alcoholism (ethanol impairs placental uptake of amino acids and glucose).
Simple (passive) diffusion.
Diffusion is movement of a substance from higher to lower concentration or electrochemical gradients (Figure 3-16, A). The quantity of a substance transferred is illustrated by the Fick diffusion equation:
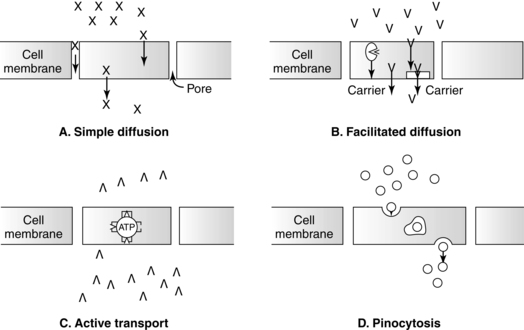
< ?xml:namespace prefix = "mml" />
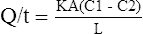
Diffusion is the major mechanism of placental transfer. Simple diffusion is generally limited to smaller molecules that can pass through pores in the cell wall. Because the cell walls have a high lipid content, some lipid-soluble substances may cross directly through these lipid regions.33 Water-soluble substances cross easily if less than 100 molecular weight (MW); lipid-soluble molecules less than 600 MW cross unimpeded. The cell walls can act as a barrier to some large non–lipid-soluble substances such as muscle relaxants used with anesthesia. Substances that cross the placenta via simple diffusion include water, electrolytes, oxygen, carbon dioxide, urea, simple amines, creatinine, fatty acids, steroids, fat-soluble vitamins, narcotics, antibiotics, barbiturates, and anesthetics.46 Fat-soluble vitamins and cholesterol diffuse as lipoprotein complexes. Compounds such as histamine, serotonin, angiotensin, and epinephrine diffuse readily, but significant concentrations may never reach the fetus due to enzymatic deamination within the placenta.33 Free water crosses at a rate of 180 mL/second, faster than any other known substance.46
Carbon dioxide is highly soluble in the placental membrane and diffuses readily. Oxygen diffuses with greater difficulty and therefore requires a considerable gradient of oxygen pressure on either side of the membrane. The average gradient is about 20 mmHg (2.66 kPa) for oxygen and 5 mmHg (0.66 kPa) for carbon dioxide. The oxygen gradient is higher because the placenta and myometrium also extract oxygen.46 The rate of gas exchange across the placenta is limited by maternal blood flow supplying oxygen to the placenta. The placenta consumes about 10% to 30% of the oxygen delivered to it.84
Although the placenta is similar to the lungs in relation to the efficiency of gas exchange, the PO2 levels of maternal and fetal blood leaving the placenta differ. These differences are due to the previously described vascular shunts in maternal and fetal circulations, nonuniform distribution of maternal and fetal blood flow, and differences in the positions of the maternal and fetal oxygen hemoglobin dissociation curves (see Chapters 6 and 10). When maternal blood flow exceeds fetal flow, the exchange of oxygen with fetal blood results in only minimal changes in maternal PO2. Blood from these compartments contributes heavily to the PO2 of mixed venous blood leaving the uterus. Conversely, oxygen exchange in areas in which fetal blood flow exceeds maternal flow depletes maternal reserves and equilibrates at a level similar to the fetal PO2.103 Placental gas exchange is discussed further in Chapter 6.
Facilitated diffusion.
Facilitated diffusion (or transplacental protein mediated diffusion) involves transport via protein transporters to move substances across the placental membrane (see Figure 3-16, B). Movement is from higher to lower concentration or electrochemical gradients. Glucose (fetal levels are 70% to 80% of maternal values) and possibly some oxygen are transported from maternal-to-fetal circulation via facilitated diffusion. Placental glucose metabolism may partially account for differences in maternal and fetal glucose concentrations. Glucose transport occurs via glucose transport proteins or GLUT (see Chapter 16).72,123 Waste products such as lactate are transported from fetus to mother via facilitated diffusion.
Active transport.
Active transport utilizes energy-dependent carrier systems and other protein transporters to move substances against concentration or electrochemical gradients (see Figure 3-16, C). Amino acids, potassium, water-soluble vitamins, calcium, phosphate, iron, and iodine cross the placenta via active transport.46,72,117 Active transport systems become saturated at high concentrations. Similar molecules may compete, reducing the movement of some substances across the placenta.
Amino acids such as alanine, glutamine, threonine, and serine that are transported by multiple carrier systems are found in high concentrations in placental tissue. Thirteen amino acid monomeric or heterodimeric transporter protein systems have been described.72 Umbilical vein concentrations of most amino acids are higher than maternal plasma concentrations from midgestation to term.72 The net movement of many amino acids is greater than that estimated for fetal growth needs, suggesting that the fetus also uses these substances for energy or the synthesis of other amino acids.72 The amount of amino acids transported across the placenta increases markedly late in pregnancy due to increased numbers of carriers. Most polypeptides and larger proteins that cross the placenta do so via endocytosis/pinocytosis or accidental capillary breaks.
Endocytosis and exocytosis.
Endocytosis is an invagination in the surface of a cell that forms intracellular membrane–bound vesicles; exocytosis occurs on the opposite side of the cell with fusion of the vesicle to the membrane and release of the vesicles’ contents.72 Endocytosis involves the engulfing of microdroplets of maternal plasma or small solutes (pinocytosis) (see Figure 3-16, D) or large particles (phagocytosis) by trophoblast cells. Substances in maternal plasma such as globulins (IgG), phospholipids, lipoproteins, and antibodies are transferred to the fetus or metabolized by the placenta. These mechanisms are necessary to transfer molecules too large for diffusion or for which no carrier transport exists and allow some molecules of up to 150,000 MW (i.e., IgG) to cross the placenta.
Maternal antibodies of the IgG class readily cross the placenta during the third trimester. This is a facilitated process that becomes more efficient in late gestation.29 Transfer may be mediated by fetal Fc receptors.29 IgG antibodies from the mother can be protective (e.g., antibodies against diphtheria, measles, mumps, herpes simplex virus) or potentially damaging (as occurs in Rh incompatibility or with maternal Graves’ disease or myasthenia gravis) to the fetus (see Chapter 13). Maternal immunoglobulin A (IgA) antibodies do not cross in significant amounts; immunoglobulin M (IgM) antibodies are not transferred.
Bulk flow, solvent drag, and water channels.
Water crosses the placenta rapidly. Bulk flow may occur with changes in hydrostatic or osmotic forces. Ions cross either by simple diffusion or by solvent drag as dissolved electrolytes are pulled across the placenta by the movement of water. This mechanism is useful in maintaining water and osmotic balances between maternal and fetal circulations.46 This mechanism may involve aqueous pores and is most effective for molecules with low MW. Water movement varies within the placenta depending on the concentrations of various osmotically active substances and the hydrostatic pressure.72 At least five different aquaporin (AQP) water channels are expressed on the placenta and fetal membranes.34 These channels play a role in the transfer of water across the fetal membranes into fetal circulation via osmotic gradients.34,135
Accidental capillary breaks.
Accidental capillary breaks and breaks in the villous covering permit passage of intact blood cells between maternal and fetal circulations. Small amounts (0.1 to 0.2 mL) of fetal cells can be found in maternal circulation intermittently during pregnancy. More extensive (≥1 to 2 mL) fetomaternal hemorrhage may occur with placental separation and increase the risk of isoimmunization (see Chapter 13).46
Independent movement.
Maternal leukocytes or organisms such as Treponema pallidum may cross the placenta under their own power. Although many viruses can infect the fetus, the specific mechanisms through which they cross the placenta are unclear. Some viruses may be carried across via pinocytosis.67
Transfer of substances across the placenta
Dancis noted that in thinking about placental transfer: “Ask not whether a maternal nutrient [or other substance] crosses the placenta. Ask rather, how, how much, and how fast. Ask also as to fetal need.”35 There are few compounds—endogenous or exogenous—that are unable to cross the placenta in detectable amounts given sufficient time and sensitivity of detection.35 Placental transfer is influenced by the area of the placenta, physicochemical characteristics of the diffusing substance, concentration gradients, electrical potential differences, diffusing distance, degree of binding of a substance to hemoglobin or other blood proteins, permeability of the placental barrier, and the rates of maternal and fetal blood flow through the intervillous space and villi.46
Diffusion of a substance across the placenta can be expressed as follows:

Physicochemical characteristics that influence movement across the placenta include lipid solubility, MW, degree of ionization, and protein binding. These characteristics can increase, decrease, or prohibit movement of potentially harmful drugs and other substances from maternal-to-fetal circulation (see Chapter 7).33,46 Placental transfer may be increased or enhanced if a substance is lipid soluble (e.g., lipoproteins) or nonionized (e.g., phenobarbital), has an MW less than 600 (e.g., propylthiouracil), or lacks significant binding to albumin (e.g., ampicillin). Increased maternal-to-fetal concentration or electrochemical gradients also increase transfer. A substance may be prevented from crossing the placenta because it has a certain charge or molecular configuration (e.g., heparin) or certain size (e.g., bacteria, IgM), is altered or bound by enzymes within the placenta (e.g., amines, insulin), or is firmly bound to the maternal red blood cell or plasma protein (e.g., carbon monoxide).46,50
The rate of maternal blood flow to and through the IVS and fetal blood flow to and through the villi influence placental transfer. Blood flow is the limiting factor in gas exchange across the placenta and also affects transfer of nutrients and waste products. During uterine contractions, the entry of blood into the IVS is limited. Transfer of oxygen and other nutrients to the fetus may decrease but does not cease during contractions.46,50 The decrease in afferent blood flow during a contraction may be due to: (1) compression and obliteration of the uteroplacental veins with increased IVS pressure, (2) occlusion of the uteroplacental arteries, or (3) increased intraluminal pressure within the uterus with alteration in the arteriovenous pressure gradients within the IVS.
In addition to uterine contractions, factors that may alter uteroplacental blood flow include maternal position; anesthesia; nicotine and other drugs; emotional or physical stress; and degenerative changes within the placenta that are seen with hypertension, prolonged pregnancy, diabetes, or renal disease. Maternal blood flow to and through the IVS can be altered by (1) changes in the systemic circulation (cardiac disease, small uterine artery); (2) changes in the number or size of the uteroplacental blood vessels (infection, degeneration); (3) compression of the uteroplacental blood vessels (tetanic or other abnormal contractions, polyhydramnios, multiple gestation); and (4) degenerative changes in the IVS associated with high-risk conditions such as maternal hypertension.46
Separation of the placenta
Placental separation and expulsion occur during the third stage of labor. The “afterbirth” consists of maternal and fetal tissues (see Figure 3-10). Maternal tissues include the decidua basalis (maternal portion of the placenta), decidua parietalis, and any remaining decidua capsularis. Fetal tissues include the chorion frondosum (fetal portion of the placenta), chorion laeve (chorion), amnion, and umbilical cord. Retained fragments of the placenta and membranes can lead to uterine atony, hemorrhage, or infection.
The sudden emptying of the uterus with delivery of the fetus rapidly reduces the surface area of the placental site to an area approximately 10 cm in diameter. This reduction in the base of support for the placenta leads to compression and shearing of the placenta from the uterine wall.84 The usual site of placental separation is within the spongy layer of the decidua basalis (see Figure 3-10). This layer has been described as “like the lines of perforation found between postal stamps.”84 The placenta may separate from the central area to the margins with inversion so that the fetal surface presents first (Schultze mechanism), or from the margins toward the center with initial presentation of the maternal surface (Duncan mechanism). Placentas implanted in the fundus of the uterus are more likely to separate via Schultze mechanism; those implanted lower on the uterine wall usually separate by Duncan mechanism (although these placentas may invert before expulsion).