The Physical Examination and Its Basis in Physiology
After reading this chapter, you will be able to:
• Describe the major components of a patient’s vital signs, including:
• Describe the systematic examination of the chest and lungs, including:
• Discuss in more depth the common clinical manifestations observed during inspection, including normal ventilatory pattern and the common pathophysiologic mechanisms that affect the ventilatory pattern.
• Describe the function of the following accessory muscles of inspiration:
• Describe the function of the following accessory muscles of expiration:
• Discuss the effects of pursed-lip breathing.
• Describe the pathophysiologic basis for substernal and intercostal retractions.
• Discuss splinting caused by chest pain or decreased chest expansion including pleuritic chest pain and nonpleuritic chest pain.
• List abnormal chest shape and configuration.
• List abnormal extremity findings, and include:
• Altered skin color (e.g., cyanotic, pale, with prominent venous distention)
• Presence or absence of digital clubbing
• Presence or absence of peripheral edema
• Presence or absence of distended neck veins
• Describe how the following correlates to normal and abnormal sputum production, including:
• Normal histology and mucous production of the tracheobronchial tree
• Define key terms and complete self-assessment questions at the end of the chapter and on Evolve.
Vital Signs
The four major vital signs—body temperature (T), pulse (P), respiratory rate (R), and blood pressure (BP)—are excellent bedside clinical indicators of the patient’s physiologic and psychologic health. In many patient care settings, the oxygen saturation as measured by pulse oximetry (Spo2) is considered to be the fifth vital sign. Table 2-1 shows the normal values that have been established for various age groups.
Table 2-1
Average Range for Vital Signs According to Age Group
Age Group | Temperature (F°) | Pulse (bpm) | Respirations (breaths/min) | Blood Pressure (mm Hg) | |
Systolic | Diastolic | ||||
Newborn | 96-99.5 | 100-180 | 30-60 | 60-90 | 20-60 |
Infant (1 mo.-1 yr) | 99.4-99.7 | 80-160 | 30-60 | 75-100 | 50-70 |
Toddler (1-3 yrs) | 99.4-99.7 | 80-130 | 25-40 | 80-110 | 55-80 |
Preschooler (3-6 yrs) | 98.6-99 | 80-120 | 20-35 | 80-110 | 50-80 |
Child (6-12 yrs) | 98.6 | 65-100 | 20-30 | 100-110 | 60-70 |
Adolescent (12-18 yrs) | 97-99 | 60-90 | 12-20 | 110-120 | 60-65 |
Adult | 97-99 | 60-100 | 12-20 | 110-140 | 60-90 |
Older adult (>70 yrs) | 95-99 | 60-100 | 12-20 | 120-140 | 70-90 |
Body Temperature
As shown in Figure 2-1, the normal body temperature is positioned within a relatively narrow range. A patient who has a temperature within the normal range is said to be afebrile. A body temperature above the normal range is called pyrexia or hyperthermia. When the body temperature rises above the normal range, the patient is said to have a fever or to be febrile. An exceptionally high temperature, such as 41° C (105.8° F), is called hyperpyrexia.
Hypothermia is a core temperature below normal range. Hypothermia may occur as a result of (1) excessive heat loss, (2) inadequate heat production to counteract heat loss, and (3) impaired hypothalamic thermoregulation. Box 2-1 lists the clinical signs of hypothermia.
Hypothermia may be caused accidentally or may be induced. Accidental hypothermia is commonly seen in the patient who (1) has had an excessive exposure to a cold environment; (2) has been immersed in a cold liquid environment for a prolonged time; or (3) has inadequate clothing, shelter, or heat. A reduced metabolic rate may compound hypothermia in older patients. In addition, older patients often take sedatives, which further depress the metabolic rate. Box 2-2 lists common therapeutic interventions for patients with hypothermia.
Factors Affecting Body Temperature
Table 2-2 lists several factors that affect body temperature. Knowing these factors can help the practitioner to better assess the significance of expected or normal variations in a patient’s body temperature.
Table 2-2
Factors Affecting Body Temperature
Age | Temperature varies with age. For example, the temperature of the newborn infant is unstable because of immature thermoregulatory mechanisms. However, it is not uncommon for the elderly person to have a body temperature below 36.4° C (97.6° F). The normal temperature decreases with age. |
Environment | Normally, variations in environmental temperature do not affect the core temperature. However, exposure to extreme hot or cold temperatures can alter body temperature. If an individual’s core temperature falls to 25° C (77° F), death may occur. |
Time of day | Body temperature normally varies throughout the day. Typically, an individual’s temperature is lowest around 3:00 am and highest between 5:00 pm and 7:00 pm. Approximately 95% of patients have their highest temperature around 6:00 pm. Body temperature often fluctuates by as much as 2° C (1.8° F) between early morning and late afternoon. |
Exercise | Body temperature increases with exercise because exercise increases heat production as the body breaks down carbohydrates and fats to provide energy. During strenuous exercise, the body temperature can increase to as high as 40° C (104° F). |
Stress | Physical or emotional stress may increase body temperature because stress can stimulate the sympathetic nervous system, causing the epinephrine and norepinephrine levels to increase. When this occurs, the metabolic rate increases, causing an increased heat production. Stress and anxiety may cause a patient’s temperature to increase without underlying disease. |
Hormones | Women normally have greater fluctuations in temperature than do men. The female hormone progesterone, which is secreted during ovulation, causes the temperature to increase 0.3° to 0.6° C (0.5° to 1° F). After menopause, women have the same mean temperature norms as men. |
Body Temperature Measurement
Because body temperature is usually measured orally, the practitioner must be aware of certain external factors that can lead to false oral temperature measurements. For example, drinking hot or cold liquids can cause small changes in oral temperature measurements. The most significant temperature changes have been reported after a patient drinks ice water. Drinking ice water may lower the patient’s actual temperature by 0.2° to 1.6° F. Before taking an oral temperature, the practitioner should wait 15 minutes after a patient has ingested ice water. Oral temperature may increase in the patient receiving heated oxygen aerosol therapy and decrease in the patient receiving a cool mist aerosol. Table 2-3 lists the body temperature sites, their advantages and disadvantages, and the equipment used.
Table 2-3
Site and Temperature | Advantages and Disadvantages | Equipment |
Oral (most common) Average 37° C or 98.6° F |
Advantages: Convenient. Easy access and patient comfort. Disadvantages: Affected by hot or cold liquids. Contraindicated in patients who cannot follow directions to keep mouth closed, who are mouth breathing, or who might bite down and break thermometer. Smoking, drinking, and eating can slightly alter the oral temperature. About 1° F lower than rectal temperature. |
Advantages: Convenient, readily accessible, fast, safe, and noninvasive. Does not require contact with any mucous membrane. Infection control is less of a concern. With the advent of the tympanic membrane thermometer, the ear is now a site where a temperature can be easily and safely measured. Reflects the core body temperature because it measures the tympanic membrane blood supply—the same vascular system that supplies the hypothalamus. Smoking, drinking, and eating do not affect tympanic temperature measurements. Allows rapid temperature measurements in the very young, confused, or unconscious patient.
Disadvantages: No remarkable disadvantages, assuming site is available.
Advantages: Safe and noninvasive. Recommended for infants and children, this is the route of choice in patients whose temperature cannot be measured at other sites.
Disadvantages: Considered the least accurate and least reliable site because a number of factors can adversely affect the measurement. For example, if the patient has recently been given a bath, the temperature may reflect the temperature of the bath water. Similarly, friction applied to dry the patient’s skin may influence the temperature.
Pulse
A pulse is generated through the vascular system with each ventricular contraction of the heart (systole). Thus a pulse is a rhythmic arterial blood pressure throb created by the pumping action of the ventricular muscle. Between contractions, the ventricle rests (diastole) and the pulsation disappears. The pulse can be assessed at any location where an artery lies close to the skin surface and can be palpated against a firm underlying structure, such as muscle or bone. Nine common pulse sites are the temporal, carotid, apical, brachial, radial, femoral, popliteal, pedal (dorsalis pedis), and posterior tibial area (Figure 2-2).
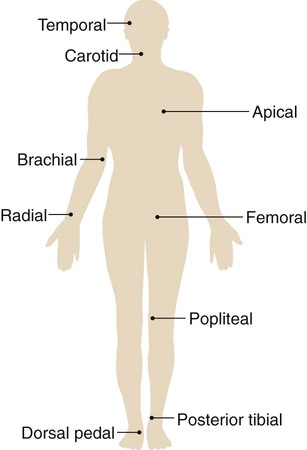
Rate
The normal pulse rate (or heart rate) varies with age. For example, in the newborn the normal pulse rate range is 100 to 180 beats per minute (bpm). In the toddler the normal range is 80 to 130 bpm. The normal range for the child is 65 to 100 bpm, and the normal adult range is 60 to 100 bpm (see Table 2-1).
Strength
Finally, the stimulation of the sympathetic nervous system increases the force of ventricular contraction, increasing the volume of blood ejected from the heart and creating a stronger pulse. Stimulation of the parasympathetic nervous system decreases the force of the ventricular contraction, thus leading to a decreased volume of blood ejected from the heart and a weaker pulse. Clinically the strength of the pulse may be recorded on a scale of 0 to 4+ (Box 2-3).
Respiration
The normal respiratory rate varies with age. For example, in the newborn the normal respiratory rate varies between 30 and 60 breaths per minute. In the toddler, the normal range is 25 to 40 breaths per minute. The normal range for the preschool child is 20 to 25 breaths per minute, and the normal adult range is 12 to 20 breaths per minute (see Table 2-1).
Ideally the respiratory rate should be counted when the patient is not aware. One good method is to count the respiratory rate immediately after taking the pulse, while leaving the fingers over the patient’s artery. As respirations are being counted, the practitioner should observe for variations in the pattern of breathing. For example, an increased breathing rate is called tachypnea. Tachypnea is commonly seen in patients with fever, metabolic acidosis, hypoxemia, pain, or anxiety. A respiratory rate below the normal range is called bradypnea. Bradypnea may occur with hypothermia, head injuries, and drug overdose. Table 2-4 provides an overview of common normal and abnormal breathing patterns.
Table 2-4
Common Normal and Abnormal Breathing Patterns
Pattern | Graphic Overview | Description |
Eupnea | ![]() |
Normal rate and rhythm; between 12 and 20 breaths per minute in regular rhythm and of moderate depth for an adult |
Bradypnea | ![]() |
Regular rhythm of less than 12 breaths per minute |
Tachypnea | ![]() |
Regular rhythm of more than 20 breaths per minute for an adult |
Apnea | ![]() |
Absence of breathing that leads to respiratory arrest and death |
Hypoventilation | ![]() |
Decreased rate and depth, decreasing alveolar ventilation and leading to an increased Paco2 |
Hyperventilation | ![]() |
Increased rate and depth, which increases alveolar ventilation and leads to a decreased Paco2 |
Cheyne-Stokes | ![]() |
Respirations that progressively become faster and deeper, followed by respirations that progressively become slower and shallower and ending with a period of apnea |
Kussmaul | ![]() |
Increased rate and depth of breathing. Usually associated with diabetic ketoacidosis as a compensatory mechanism to eliminate carbon dioxide, by buffering the metabolic acidosis |
Biot’s | ![]() |
Fast, deep respirations with abrupt pauses |
Blood Pressure
The normal blood pressure in the aorta and large arteries varies with age. For example, in the newborn the normal systolic blood pressure range is 60 to 180 mm Hg. In the toddler the normal range is 80 to 110 mm Hg. The normal range for the child is 100 to 110 mm Hg, and the normal adult range is 110 to 140 mm Hg (see Table 2-1). The numeric difference between the systolic and diastolic blood pressure is the pulse pressure. For example, a systolic pressure of 120 mm Hg and a diastolic pressure of 80 mm Hg equal a pulse pressure of 40 mm Hg.
Resistance
Table 2-5 presents factors that affect the blood pressure.
Table 2-5
Factors Affecting Blood Pressure
Age | Blood pressure gradually increases throughout childhood, and correlates with height, weight, and age. In the adult, the blood pressure tends to gradually increase with age. |
Exercise | Vigorous exercise increases cardiac output and thus blood pressure. |
Autonomic nervous system | Increased sympathetic nervous system activity causes an increased heart rate, an increased cardiac contractility, changes in vascular smooth muscle tone to enhance blood flow to vital organs and skeletal muscles, and an increased blood volume. Collectively, these actions cause an increased blood pressure. |
Stress | Stress stimulates the sympathetic nervous system and thus can increase blood pressure. |
Circulating blood volume | A decreased circulating blood volume, either from blood or fluid loss, causes blood pressure to decrease. Common causes of fluid loss include abnormal, unreplaced fluid losses such as in diarrhea or diaphoresis, and overenthusiastic use of diuretics. Inadequate oral fluid intake can also result in a fluid volume deficit. Excess fluid, such as in congestive heart failure, can cause the blood pressure to increase. |
Medications | Any medication that affects one or more of the previous conditions may cause blood pressure changes. For example, diuretics reduce blood volume; cardiac pharmaceuticals may increase or decrease heart rate and contractility; pain medications may reduce sympathetic nervous system stimulation; and specific antihypertension agents may exert their effects as well. |
Normal fluctuations | Under normal circumstances, blood pressure varies from moment to moment in response to a variety of stimuli. For example, an increased environmental temperature causes blood vessels near the skin surface to dilate, causing blood pressure to decrease. In addition, normal respirations alter blood pressure: Blood pressure increases during expiration and decreases during inspiration. Blood pressure fluctuations caused by inspiration and expiration may be significant during a severe asthmatic episode. |
Race | Black males over 35 years of age often have elevated blood pressures. |
Obesity | Blood pressure is often higher in overweight and obese individuals. |
Daily variations | Blood pressure is usually lowest early in the morning, when the metabolic rate is lowest. |
Oxygen Saturation
Oxygen saturation, often considered the fifth vital sign, is used to establish an immediate baseline Spo2 value. It is an excellent monitor by which to assess the patient’s response to respiratory care interventions. In the adult, normal Spo2 values range from 95% to 99%. Spo2 values of 91% to 94% indicate mild hypoxemia. Mild hypoxemia warrants additional evaluation by the respiratory practitioner but does not usually require supplemental oxygen. Spo2 readings of 86% to 90% indicate moderate hypoxemia. These patients often require supplemental oxygen. Spo2 values of 85% or lower indicate severe hypoxemia and warrant immediate medical intervention, including the administration of oxygen, ventilatory support, or both. Table 2-6 presents the relationship of Spo2 to Pao2 for the adult and newborn. Table 2-7 provides an overview of the signs and symptoms of inadequate oxygenation.
Table 2-6
Spo2 and Pao2 Relationship for the Adult and Newborn
Oxygen Status | Adult | Newborn | ||
Spo2 | Pao2 | Spo2 | Pao2 | |
Normal | 95-99% | 75-100 | 91-96% | 60-80 |
Mild hypoxemia | 90-95% | 60-75 | 88-90% | 55-60 |
Moderate hypoxemia | 85-90% | 50-60 | 85-89% | 50-58 |
Severe hypoxemia | <85% | <50 | <85% | <50 |
Table 2-7
Signs and Symptoms of Inadequate Oxygenation
Central Nervous System | |
Apprehension | Early |
Restlessness or irritability | Early |
Confusion or lethargy | Early or late |
Combativeness | Late |
Coma | Late |
Respiratory | |
Tachypnea | Early |
Dyspnea on exertion | Early |
Dyspnea at rest | Late |
Use of accessory muscles | Late |
Intercostal retractions | Late |
Takes a breath between each word or sentence | Late |
Cardiovascular | |
Tachycardia | Early |
Mild hypertension | Early |
Arrhythmias | Early or late |
Hypotension | Late |
Cyanosis | Late |
Skin is cool or clammy | Late |
Other | |
Diaphoresis | Early or late |
Decreased urinary output | Early or late |
General fatigue | Early or late |
Systematic Examination of the Chest and Lungs
Lung and Chest Topography
Thoracic Cage Landmarks
Anteriorly, the first rib is attached to the manubrium just beneath the clavicle. After the first rib is identified, the rest of the ribs can easily be located and numbered. The sixth rib and its cartilage are attached to the sternum just above the xiphoid process (Figure 2-3).
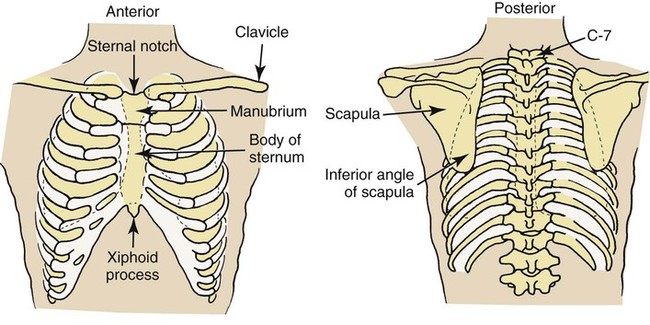
Posteriorly, the spinous processes of the vertebrae are useful landmarks. For example, when the patient’s head is extended forward and down, two prominent spinous processes can usually be seen at the base of the neck. The top one is the spinous process of the seventh cervical vertebra (C-7); the bottom one is the spinous process of the thoracic vertebra (T-1). When only one spinous process can be seen, it is usually C-7 (see Figure 2-3).
Imaginary Lines
Various imaginary vertical lines are used to locate abnormalities on chest examination (Figure 2-4). The midsternal line, which is located in the middle of the sternum, equally divides the anterior chest into left and right hemithoraces. The midclavicular lines, which start at the middle of either the right or left clavicle, run parallel to the sternum.
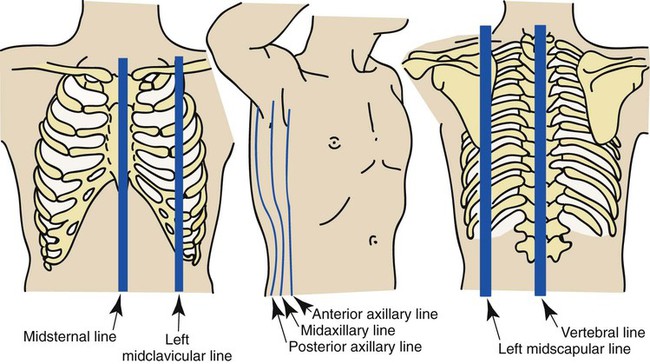
Lung Borders and Fissures
Anteriorly, the apex of the lung extends about 2 to 4 cm above the medial third of the clavicle. Under normal conditions the lungs extend down to about the level of the sixth rib. Posteriorly, the superior portion of the lung extends to about the level of T-1 and down to about the level of T-10 (Figure 2-5).
Inspection
Common Clinical Manifestations Observed during Inspection
Box 2-4 lists common clinical manifestations observed during the inspection of the patient with a pathologic respiratory condition. For example, during a systematic visual inspection, the respiratory practitioner might note the patient’s ventilatory pattern. Is the patient using accessory muscles of inspiration? Is the patient engaging in pursed-lip breathing? Are substernal or intercostal retractions occurring during inspiration? Does the patient appear to be splinting or to have decreased chest expansion because of chest pain? Are the shape and configuration of the chest normal? Do the patient’s skin, lips, fingers, or toenails appear cyanotic? Does the patient have digital clubbing, pedal edema, or distended neck veins? Is the patient coughing? How strong is the patient’s cough? What are the characteristics of the patient’s sputum? A more in-depth discussion of common clinical manifestations observed during inspection can be found later in this chapter (see page 28).
Palpation
Palpation is the process of touching the patient’s chest to evaluate the symmetry of chest expansion, the position of the trachea, skin temperature, muscle tone, areas of tenderness, lumps, depressions, and tactile and vocal fremitus. When palpating the chest, the clinician may use the heel or ulnar side of the hand, the palms, or the fingertips. As shown in Figure 2-6, both the anterior and posterior chest should be palpated from side to side in an orderly fashion, from the apices of the chest down.
Chest Excursion
The symmetry of chest expansion is evaluated by lightly placing each hand over the patient’s posterolateral chest so that the thumbs meet at the midline at about the T-8 to T-10 level. The patient is instructed to exhale slowly and completely and then to inhale deeply. As the patient is inhaling, the examiner evaluates the distance that each thumb moves from the midline. Normally, each thumb tip moves equally about 3 to 5 cm from the midline (Figure 2-7).
Percussion
Percussion over the chest wall is performed to determine the size, borders, and consistency of air, liquid, or solid material in the underlying lung. When percussing the chest, the examiner firmly places the distal portion of the middle finger of the nondominant hand between the ribs over the surface of the chest area to be examined. No other portion of the hand should touch the patient’s chest. With the end of the middle finger of the dominant hand, the examiner quickly strikes the distal joint of the finger positioned on the chest wall and then quickly withdraws the tapping finger (Figure 2-8). The examiner should perform the chest percussion in an orderly fashion from top to bottom, comparing the sounds generated on both sides of the chest, both anteriorly and posteriorly (Figure 2-9).
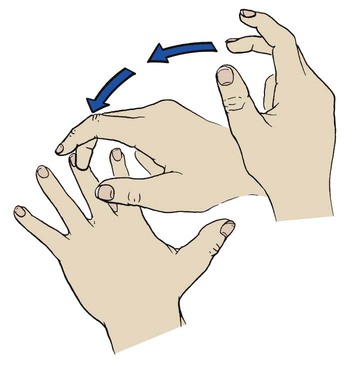
In the normal lung the sound created by percussion is transmitted throughout the air-filled lung and is typically described as loud, low in pitch, and long in duration. The sounds elicited by the examiner vibrate freely throughout the large surface area of the lungs and create a sound similar to that elicited by knocking on a watermelon (Figure 2-10).
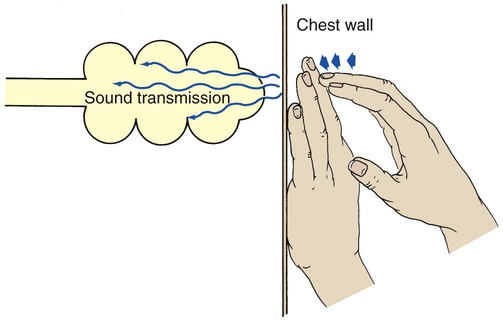
Abnormal Percussion Notes
A dull percussion note is heard when the chest is percussed over areas of pleural thickening, pleural effusion, atelectasis, and consolidation. When these conditions exist, the sounds produced by the examiner do not freely vibrate throughout the lungs. A dull percussion note is described as flat or soft, high in pitch, and short in duration, similar to the sound produced by knocking on a full barrel (Figure 2-11).
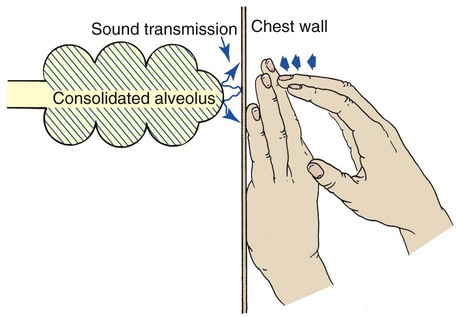
When the chest is percussed over areas of trapped gas, a hyperresonant note is heard. These sounds are described as very loud, low in pitch, and long in duration, similar to the sound produced by knocking on an empty barrel (Figure 2-12). A hyperresonant note is commonly elicited in the patient with chronic obstructive pulmonary disease or pneumothorax.
Auscultation
Auscultation of the chest provides information about the heart, blood vessels, and air flowing in and out of the tracheobronchial tree and alveoli. A stethoscope is used to evaluate the frequency, intensity, duration, and quality of the sounds. During auscultation the patient should ideally be in the upright position and instructed to breathe slowly and deeply through the mouth. The anterior and posterior chest should be auscultated in an orderly fashion from the apex to base while the right side of the chest is compared with the left (Figure 2-13). When examining the posterior chest, the examiner should ask the patient to rotate the shoulders forward so that a greater surface area of the lungs can be auscultated.
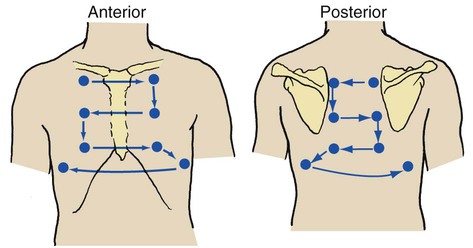
Normal Breath Sounds
Bronchovesicular breath sounds
Anteriorly, bronchovesicular breath sounds can be heard directly over the mainstem bronchi between the first and second ribs. Posteriorly, they are heard between the scapulae near the spinal column between the first and sixth ribs, especially on the right side (Figure 2-14, A).
Vesicular breath sounds
Vesicular breath sounds are the normal sounds of gas rustling or swishing through the small bronchioles and possibly the alveoli. Under normal conditions, vesicular breath sounds are auscultated over most of the lung field, both anteriorly and posteriorly (see Figure 2-14, B). Vesicular breath sounds are described as soft and low in pitch and are primarily heard during inspiration. As the gas molecules enter the alveoli, they are able to spread out over a large surface area and, as a result of this action, create less gas turbulence. As gas turbulence decreases, the breath sounds become softer and lower in pitch, similar to the sound of the wind in the trees. Vesicular breath sounds also are heard during the initial third of exhalation as gas leaves the alveoli and bronchioles and moves into the large airways (Figure 2-15).
Adventitious (Abnormal) Breath Sounds
Bronchial breath sounds
It is commonly believed that breath sounds in patients with alveolar consolidation should be diminished because the consolidation acts as a sound barrier. Although alveolar collapse or consolidation does act as a sound barrier and reduces bronchial breath sounds, the reduction is not as great as it would be if the gas molecules were allowed to dissipate throughout normal lung parenchyma. In addition, liquid and solid materials transmit sounds more readily than air-filled spaces and therefore may further contribute to the bronchial quality of the breath sound. Therefore when disease causes alveolar collapse or consolidation, harsher, bronchial-type sounds rather than the normal vesicular sounds are heard over the affected areas (Figure 2-16).
Diminished breath sounds
Breath sounds are diminished or distant in respiratory disorders that lead to alveolar hypoventilation, regardless of the cause. For example, patients with chronic obstructive pulmonary disease often have diminished breath sounds. These patients hypoventilate because of air trapping and increased functional residual capacity. In addition, when the functional residual capacity is increased, the gas that enters the enlarged alveoli during each breath spreads out over a greater-than-normal surface area, resulting in less gas turbulence and a softer sound (Figure 2-17). Heart sounds also may be diminished in patients with air trapping.
Wheezing
Wheezing is the characteristic sound produced by airway obstruction. Found in all bronchospastic disorders, it is one of the cardinal findings in bronchial asthma. The sounds are high-pitched and whistling and generally last throughout the expiratory phase. The mechanism of a wheeze is similar to the vibrating reed of a woodwind instrument. The reed, which partially occludes the mouthpiece of the instrument, vibrates and produces a sound when air is forced through it (Figure 2-18). The softest, higher-pitched wheezes occur in the tightest airway obstruction.
Whispering pectoriloquy
Whispering pectoriloquy is the term used to describe the unusually clear transmission of the whispered voice of a patient as heard through the stethoscope. When the patient whispers “one, two, three,” the sounds produced by the vocal cords are transmitted not only toward the mouth and nose but throughout the lungs as well. As the whispered sounds travel down the tracheobronchial tree, they remain relatively unchanged, but as the sound disperses throughout the large surface area of the alveoli, it diminishes sharply. Consequently, when the examiner listens with a stethoscope over a normal lung while a patient whispers “one, two, three,” the sounds are diminished, distant, muffled, and unintelligible (Figure 2-19).
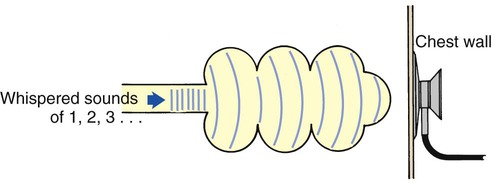
When a patient who has atelectasis or consolidated lung areas whispers “one, two, three,” the sounds produced are prevented from spreading out over a large alveolar surface area. Even though the consolidated area may act as a sound barrier and diminish the sounds somewhat, the reduction in sound is not as great as it would be if the sounds were allowed to dissipate throughout a normal lung. Consequently the whispered sounds are much louder and more intelligible over the affected lung areas (Figure 2-20).
Table 2-8 provides an overview of the common assessment abnormalities found during inspection, palpation, percussion, and auscultation.
Table 2-8
Common Assessment Abnormalities
Finding | Description | Possible Etiology and Significance |
Inspection | ||
Pursed-lip breathing | Exhalation through mouth with lips pursed together to slow exhalation. | COPD, asthma. Suggests ↑ breathlessness. Strategy taught to slow expiration, ↓ dyspnea. |
Tripod position; inability to lie flat | Leaning forward with arms and elbows supported on overbed table. | COPD, asthma in exacerbation, pulmonary edema. Indicates moderate to severe respiratory distress. |
Accessory muscle use; intercostal retractions | Neck and shoulder muscles used to assist breathing. Muscles between ribs pull in during inspiration. | COPD, asthma in excerbation, secretion retention. Indicates severe respiratory distress, hypoxemia. |
Splinting | Voluntary ↓ in tidal volume to ↓ pain on chest expansion. | Thoracic or abdominal incision. Chest trauma, pleurisy. |
↑ AP diameter | AP chest diameter equal to lateral. Slope of ribs more horizontal (90 degrees) to spine. | COPD, asthma, cystic fibrosis. Lung hyperinflation. Advanced age. |
Tachypnea | Rate >20 breaths/min; >25 breaths/min in elderly. | Fever, anxiety, hypoxemia, restrictive lung disease. Magnitude of ↑ above normal rate reflects increased work of breathing. |
Kussmaul’s respirations | Regular, rapid, and deep respirations. | Metabolic acidosis; ↑ in rate aids body in ↑ CO2 excretion. |
Cyanosis | Bluish color of skin best seen in earlobes, under the eyelids, or in nail beds. | ↓ Oxygen transfer in lungs, ↓ cardiac output. Nonspecific, unreliable indicator. |
Clubbing of fingers | ↑ Depth, bulk, sponginess of distal digit of finger. | Chronic hypoxemia. Cystic fibrosis, lung cancer, bronchiectasis. |
Peripheral edema | Pitting edema. | Congestive heart failure, cor pulmonale. |
Distended neck veins | Jugular venous distention. | Cor pulmonale, flail chest, pneumothorax. |
Cough | Productive or non-productive. | Bronchial airway and alveolar disease. |
Sputum | See Table 2-11 | COPD, asthma, cystic fibrosis. |
Abdominal paradox | Inward (rather than normal outward) movement of abdomen during inspiration. | Inefficient and ineffective breathing pattern. Nonspecific indicator of severe respiratory distress. |
Palpation | ||
Tracheal deviation | Leftward or rightward movement of trachea from normal midline position. | Nonspecific indicator of change in position of mediastinal structures. Medical emergency if caused by tension pneumothorax. |
Altered tactile fremitus | Increase or decrease in vibrations. | ↑ In pneumonia, atelectasis; pulmonary edema; ↓ in pleural effusion, lung hyperinflation; absent in pneumothorax. |
Altered chest movement | Unequal or equal but diminished movement of two sides of chest with inspiration. | Unequal movement caused by atelectasis, pneumothorax, pleural effusion, splinting; equal but diminished movement caused by barrel chest, restrictive disease, neuromuscular disease. |
Percussion | ||
Hyperresonance | Loud, lower-pitched sound over areas that normally produce a resonant sound. | Lung hyperinflation (COPD), lung collapse (pneumothorax), air trapping (asthma). |
Dullness/Flatness | Medium-pitched sound over areas that normally produce a resonant sound. | ↑ Density (pneumonia, large atelectasis), ↑ fluid pleural space (pleural effusion). |
Auscultation | ||
Fine crackles | Series of short, explosive, high-pitched sounds heard just before the end of inspiration; result of rapid equalization of gas pressure when collapsed alveoli or terminal bronchioles suddenly snap open; similar sound to that made by rolling hair between fingers just behind ear. | Interstitial fibrosis (asbestosis), interstitial edema (early pulmonary edema), alveolar filling (pneumonia), loss of lung volume (atelectasis), early phase of congestive heart failure. |
Coarse crackles | Series of short, low-pitched sounds caused by air passing through airway intermittently occluded by mucus, unstable bronchial wall, or fold of mucosa; evident on inspiration and, at times, expiration; similar sound to blowing through straw under water; increase in bubbling quality with more fluid. | Congestive heart failure, pulmonary edema, pneumonia with severe congestion, COPD. |
Rhonchi | Continuous rumbling, snoring, or rattling sounds from obstruction of large airways with secretions; most prominent on expiration; change often evident after coughing or suctioning. | COPD, cystic fibrosis, pneumonia, bronchiectasis. |
Wheezes | Continuous high-pitched squeaking sound caused by rapid vibration of bronchial walls; first evident on expiration but possibly evident on inspiration as obstruction of airway increases; possibly audible without stethoscope. | Bronchospasm (caused by asthma), airway obstruction (caused by foreign body, tumor), COPD. |
Stridor | Continuous musical sound of constant pitch; result of partial obstruction of larynx or trachea. | Croup, epiglottitis, vocal cord edema after extubation, foreign body. |
Absent breath sounds | No sound evident over entire lung or area of lung. | Pleural effusion, mainstem bronchi obstruction, large atelectasis, pneumonectomy, lobectomy. |
Pleural friction rub | Creaking or grating sound from roughened, inflamed surfaces of the pleura rubbing together; evident during inspiration, expiration, or both and no change with coughing; usually uncomfortable, especially on deep inspiration. | Pleurisy, pneumonia, pulmonary infarct. |
Bronchophony, whispered pectoriloquy | Spoken or whispered syllable more distinct than normal on auscultation. | Pneumonia. |
Egophony | Spoken “e” similar to “a” on auscultation because of altered transmission of voice sounds. | Pneumonia, pleural effusion. |
Modified from Lewis S, Heitkemper MM, Dirksen SR: Medical-surgical nursing: Assessment and management of clinical problems, ed 7, vol. 1, St. Louis, 2007, Mosby.
In-Depth Discussion of Common Clinical Manifestations Observed during Inspection
Normal Ventilatory Pattern
An individual’s normal breathing pattern is composed of a tidal volume (VT), a ventilatory rate and an inspiratory-to-expiratory ratio (I:E ratio). In normal adults the VT is about 500 mL (7 to 9 mL/kg), the ventilatory rate is about 15 (with a range of 12 to 18) breaths per minute, and the I:E ratio is about 1 : 2. In patients with respiratory disorders, however, an abnormal ventilatory pattern is often present (see Table 2-4 for common abnormal ventilatory patterns).
Common Pathophysiologic Mechanisms That Affect the Ventilatory Pattern
Lung Compliance and Its Effect on the Ventilatory Pattern
< ?xml:namespace prefix = "mml" />
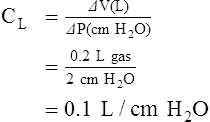
The normal compliance of the lungs is graphically illustrated by the volume-pressure curve (Figure 2-21). When CL increases, the lungs accept a greater volume of gas per unit pressure change. When CL decreases, the lungs accept a smaller volume of gas per unit pressure change (Figure 2-22).
Although the precise mechanism is not clear, the fact that certain ventilatory patterns occur when lung compliance is altered is well documented. For example, when CL decreases, the patient’s breathing rate generally increases while the tidal volume simultaneously decreases (Figure 2-23). This type of breathing pattern is commonly seen in restrictive lung disorders such as pneumonia, pulmonary edema, and adult respiratory distress syndrome. This breathing pattern also is commonly seen during the early stages of an acute asthmatic attack when the alveoli are overinflated; CL progressively decreases as the alveolar volume increases (see Figure 2-21) at high lung volumes.
Airway Resistance and Its Effect on the Ventilatory Pattern
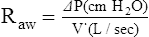
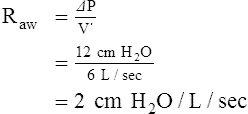
When airway resistance increases significantly, the patient’s ventilatory rate usually decreases while the tidal volume simultaneously increases (see Figure 2-23). This type of breathing pattern is commonly seen in large airway obstructive lung diseases (e.g., chronic bronchitis, bronchiectasis, asthma, cystic fibrosis) during the advanced stages.
Peripheral Chemoreceptors and Their Effect on the Ventilatory Pattern
The peripheral chemoreceptors (also called carotid and aortic bodies) are oxygen-sensitive cells that react to a reduction of oxygen in the arterial blood (Pao2). The peripheral chemoreceptors are located at the bifurcation of the internal and external carotid arteries (Figure 2-24) and on the aortic arch (Figure 2-25). Although the peripheral chemoreceptors are stimulated whenever the Pao2 is less than normal, they are generally most active when the Pao2 falls below 60 mm Hg (Sao2 of about 90%). Suppression of these chemoreceptors, however, is seen when the Pao2 falls below 30 mm Hg.
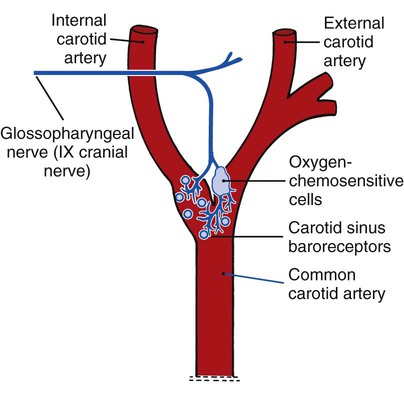
Causes of hypoxemia
In respiratory disease, a decreased arterial oxygen level (hypoxemia) is the result of a decreased ventilation-perfusion ratio, pulmonary shunting, and venous admixture (see Chapter 5 for a broader discussion of hypoxemia).
Decreased ventilation-perfusion ratios
Ideally, each alveolus should receive the same ratio of ventilation and pulmonary capillary blood flow. In reality, however, this is not the case. Alveolar ventilation is normally about 4 L/min, and the pulmonary capillary blood flow is about 5 L/min, which makes the overall ratio of ventilation to blood flow 4 : 5, or 0.8. This relationship is referred to as the ventilation-perfusion () ratio (Figure 2-26).
In some disorders, such as pulmonary embolism, the lungs receive less blood flow in relation to ventilation. When this condition develops, the ratio increases. A larger portion of the alveolar ventilation therefore will not be physiologically effective and will be said to demonstrate “wasted” or dead-space ventilation (Figure 2-27).
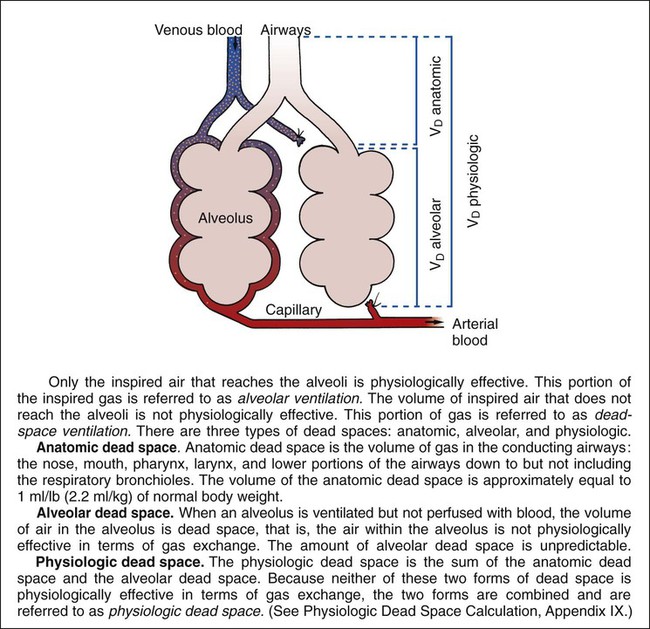
In most lung disorders (e.g., asthma, emphysema, pulmonary edema, or pneumonia), the lungs receive less ventilation in relation to blood flow. When this condition develops, the ratio decreases. A larger portion of the pulmonary blood flow is not physiologically effective in terms of molecular gas exchange and is said to be “shunted” blood (see the following section on pulmonary shunting). Generally, when the
ratio decreases, the Pao2 decreases and the Paco2 increases.
Pulmonary shunting
An anatomic shunt exists when blood flows from the right side of the heart to the left side without coming in contact with an alveolus for gas exchange (Figure 2-28, B). In the healthy individual, the normal anatomic shunt is about 3% of the cardiac output. This normal shunting is caused by nonoxygenated blood completely bypassing the alveoli and entering (1) the pulmonary vascular system by means of the bronchial venous drainage, and (2) the left atrium by way of the thebesian veins. Common causes of anatomic shunts include the following:
When pulmonary capillary perfusion is in excess of alveolar ventilation, a relative shunt, or shuntlike effect, is said to be present (see Figure 2-28, D). Common causes of a relative shunt include (1) hypoventilation, (2) decreased ratios (e.g., emphysema, chronic bronchitis, asthmatic episode, excessive airway secretions), and (3) increased alveolar-capillary membrane thickness disorders (e.g., pulmonary edema, acute respiratory distress syndrome, pneumoconiosis, chronic interstitial lung disease).
Table 2-9 illustrates the type of pulmonary shunting associated with common diseases.
Table 2-9
Type of Pulmonary Shunting Associated with Common Respiratory Diseases
Respiratory Diseases | Capillary Shunt | Relative or Shuntlike Effect |
Chronic bronchitis | X | |
Emphysema | X | |
Asthma | X | |
Croup/epiglottitis | X | |
Bronchiectasis* | X | X |
Cystic fibrosis* | X | X |
Pneumoconiosis* | X | X |
Pneumonia | X | |
Lung abscess | X | |
Pulmonary edema | X | |
Near-drowning | X | |
Adult respiratory distress syndrome | X | |
Chronic interstitial lung disease | X | |
Flail chest | X | |
Pneumothorax | X | |
Pleural diseases | X | |
Kyphoscoliosis | X | |
Tuberculosis | X | |
Fungal diseases | X | |
Idiopathic (infant) respiratory distress syndrome | X | |
Smoke inhalation | X |
Venous admixture
The result of pulmonary shunting is venous admixture, which is the mixing of shunted nonreoxygenated blood with reoxygenated blood distal to the alveoli (i.e., downstream in the pulmonary circulatory system; Figure 2-29). When venous admixture occurs, the shunted nonreoxygenated blood gains oxygen molecules while the reoxygenated blood loses oxygen molecules. The result is a blood mixture that has (1) higher Po2 and Cao2 values than the nonreoxygenated blood and (2) lower Po2 and Cao2 values than the reoxygenated blood—in other words, a blood mixture with Pao2 and Cao2 values somewhere between the original values of the reoxygenated and nonreoxygenated blood. Clinically, this mixed blood is sampled downstream (e.g., from the radial artery) to assess the patient’s arterial blood gases.
The peripheral chemoreceptors are frequently stimulated in respiratory disease because they respond to hypoxemia caused by decreased ratios, pulmonary shunting, and venous admixture. The decreased arterial oxygen tension then stimulates the peripheral chemoreceptors to send a signal to the medulla to increase ventilation (Figure 2-30).
Central Chemoreceptors and Their Effect on the Ventilatory Pattern
Although the mechanism is not fully understood, it is now believed that two special respiratory centers in the medulla, the dorsal respiratory group (DRG) and the ventral respiratory group (VRG), are responsible for coordinating respiration (Figure 2-31). Both the DRG and VRG are stimulated by an increased concentration of H+ in the CSF. The H+ concentration of the CSF is monitored by the central chemoreceptors, which are located bilaterally and ventrally in the substance of the medulla. A portion of the central chemoreceptor region is actually in direct contact with the CSF. The central chemoreceptors transmit signals to the respiratory neurons by the following mechanism:
1. When the CO2 level increases in the blood (e.g., during periods of hypoventilation), CO2 molecules readily diffuse across the blood-brain barrier and enter the CSF. The blood-brain barrier is a semipermeable membrane that separates circulating blood from the CSF. The blood-brain barrier is relatively impermeable to ions such as H+ and HCO3− but is very permeable to CO2.
2. After CO2 crosses the blood-brain barrier and enters the CSF, it forms carbonic acid:

3. Because the CSF has an inefficient buffering system, the H+ produced from the previous reaction rapidly increases and causes the pH of the CSF to decrease.
4. The central chemoreceptors react to the liberated H+ by sending signals to the respiratory components of the medulla, which in turn increases the ventilatory rate.
5. The increased ventilatory rate causes the Paco2 and subsequently the Pco2 in the CSF to decrease. Therefore the CO2 level in the blood regulates ventilation by its indirect effect on the pH of the CSF (Figure 2-32).
Use of the Accessory Muscles of Inspiration
Scalenes
The anterior, medial, and posterior scalene muscles are separate muscles that function as a unit. They originate on the transverse processes of the second to sixth cervical vertebrae and insert into the first and second ribs (Figure 2-33). These muscles normally elevate the first and second ribs and flex the neck. When they are used as accessory muscles of inspiration, their primary role is to elevate the first and second ribs.
Pectoralis Major Muscles
The pectoralis major muscles are powerful, fan-shaped muscles that originate from the clavicle and sternum and insert into the upper part of the humerus. The primary function of the pectoralis muscles is to pull the upper part of the arm to the body in a hugging motion (Figure 2-35).
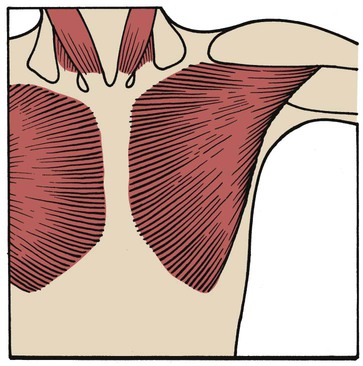
When operating as an accessory muscle of inspiration, the pectoralis pulls from the humeral insertion and elevates the chest, resulting in an increased anteroposterior diameter. Patients with chronic obstructive pulmonary disease usually secure their arms to something stationary and use the pectoralis major muscles to increase the anteroposterior diameter of the chest (Figure 2-36). This braced position is called the emphysematous habitus.
Trapezius
The trapezius is a large, flat, triangular muscle that is situated superficially in the upper part of the back and the back of the neck. The muscle originates from the occipital bone, the ligamentum nuchae, the spinous processes of the seventh cervical vertebra, and all the thoracic vertebrae. It inserts into the spine of the scapula, the acromion process, and the lateral third of the clavicle (Figure 2-37). The trapezius muscle rotates the scapula, raises the shoulders, and abducts and flexes the arm. Its action is typified in shrugging the shoulders (Figure 2-38). When used as an accessory muscle of inspiration, the trapezius helps elevate the thoracic cage.
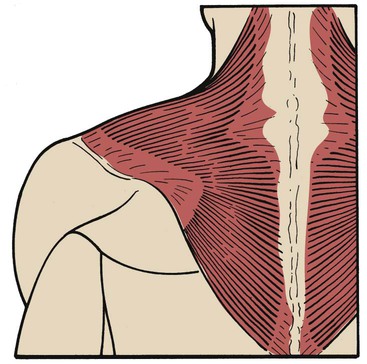
Use of the Accessory Muscles of Expiration
Rectus Abdominis
A pair of rectus abdominis muscles extends the entire length of the abdomen. Each muscle forms a vertical mass about 4 inches wide, separated by the linea alba. It arises from the iliac crest and pubic symphysis and inserts into the xiphoid process and the fifth, sixth, and seventh ribs. When activated, the muscle assists in compressing the abdominal contents, which in turn push the diaphragm into the thoracic cage (Figure 2-39).
External Obliques
The broad, thin, external oblique muscle is on the anterolateral side of the abdomen. The muscle is the longest and most superficial of all the anterolateral muscles of the abdomen. It arises by eight digitations from the lower eight ribs and the abdominal aponeurosis. It inserts in the iliac crest and into the linea alba. The muscle assists in compressing the abdominal contents. This action also pushes the diaphragm into the thoracic cage during exhalation (see Figure 2-39).
Internal Obliques
The internal oblique muscle is in the lateral and ventral part of the abdominal wall directly under the external oblique muscle. It is smaller and thinner than the external oblique. It arises from the inguinal ligament, the iliac crest, and the lower portion of the lumbar aponeurosis. It inserts into the last four ribs and the linea alba. The muscle assists in compressing the abdominal contents and pushing the diaphragm into the thoracic cage (see Figure 2-39).
Transversus Abdominis
The transversus abdominis muscle is found immediately under each internal oblique muscle. It arises from the inguinal ligament, the iliac crest, the thoracolumbar fascia, and the lower six ribs. It inserts into the linea alba. When activated, it constricts the abdominal contents (see Figure 2-39).
When all four pairs of accessory muscles of exhalation contract, the abdominal pressure increases and drives the diaphragm into the thoracic cage. As the diaphragm moves into the thoracic cage during exhalation, the intrapleural pressure increases and enhances expiratory gas flow (Figure 2-40).
Pursed-Lip Breathing
Pursed-lip breathing occurs in patients during the advanced stages of obstructive pulmonary disease. It is a relatively simple technique that many patients learn without formal instruction. During pursed-lip breathing the patient exhales through lips that are held in a position similar to that used for whistling, kissing, or blowing through a flute. The positive pressure created by retarding the airflow through pursed lips provides the airways with some stability and an increased ability to resist surrounding intrapleural pressures. This action offsets early airway collapse and air trapping during exhalation. In addition, pursed-lip breathing has been shown to slow the patient’s ventilatory rate and generate a ventilatory pattern that is more effective in gas mixing (Figure 2-41).
Substernal and Intercostal Retractions
Substernal and intercostal retractions may be seen in patients with severe restrictive lung disorders such as pneumonia or adult respiratory distress syndrome. In an effort to overcome the low lung compliance, the patient must generate a greater-than-normal negative intrapleural pressure during inspiration. This greater negative intrapleural pressure causes the tissues between the ribs and the substernal area to retract during inspiration (Figure 2-42). Because the thorax of the newborn is quite flexible (as a result of the large amount of cartilage found in the skeletal structure), substernal and intercostal retractions are seen in infants with idiopathic respiratory distress syndrome (IRDS).
Nasal Flaring
Nasal flaring is often seen during inspiration in infants experiencing respiratory distress. It is likely a facial reflex that enhances the movement of gas into the tracheobronchial tree. The dilator naris, which originates from the maxilla and inserts into the ala of the nose, is the muscle responsible for this clinical manifestation. When activated the dilator naris pulls the alae laterally and widens the nasal aperture, providing a larger orifice for gas to enter the lungs during inspiration (see Chapter 31).
Splinting Caused by Chest Pain or Decreased Chest Expansion
Pleuritic Chest Pain
Pleuritic chest pain is usually described as a sudden, sharp, or stabbing pain. The pain generally intensifies during deep inspiration and coughing and diminishes during breath holding or splinting. The origin of the pain may be the chest wall, muscles, ribs, parietal pleura, diaphragm, mediastinal structures, or intercostal nerves. Because the visceral pleura, which covers the lungs, does not have any sensory nerve supply, pain originating in the parietal region signifies extension of inflammation from the lungs to the contiguous parietal pleura lining the inner surface of the chest wall. This condition is known as pleurisy (Figure 2-43). When a patient with pleurisy inhales, the lung expands, irritating the inflamed parietal pleura and causing pain.
Abnormal Chest Shape and Configuration
During inspection the respiratory care practitioner systematically observes the patient’s chest for both normal and abnormal findings. Is the spine straight? Are any lesions or surgical scars evident? Are the scapulae symmetric? Common chest deformities are listed in Table 2-10.
Table 2-10
Common Abnormal Chest Shapes and Configurations
Condition | Description |
Kyphosis | A “hunchbacked” appearance caused by posterior curvature of the spine |
Scoliosis | A lateral curvature of the spine that results in the chest protruding posteriorly and the anterior ribs flattening out |
Kyphoscoliosis | The combination of kyphosis and scoliosis (see Figure 25-1) |
Pectus carinatum | The forward projection of the xiphoid process and lower sternum (also known as “pigeon breast” deformity) |
Pectus excavatum | A funnel-shaped depression over the lower sternum (also called “funnel chest”) |
Barrel chest | In the normal adult, the anteroposterior diameter of the chest is about half its lateral diameter, or 1 : 2. When the patient has a barrel chest, the ratio is nearer to 1 : 1 (Figure 2-44) |
Abnormal Extremity Findings
The inspection of the patient’s extremities should include the following:
• Altered skin color (e.g., cyanotic, pale, with prominent venous distention)
• Presence or absence of digital clubbing
Cyanosis
Cyanosis is common in severe respiratory disorders. Cyanosis is the term used to describe the blue-gray or purplish discoloration of the mucous membranes, fingertips, and toes whenever the blood in these areas contains at least 5 g/dL of reduced hemoglobin. When the normal 14 to 15 g/dL of hemoglobin is fully saturated, the Pao2 is about 97 to 100 mm Hg, and there is about 20 vol% of oxygen in the blood. In a cyanotic patient with one third (5 g/dL) of the hemoglobin reduced, the Pao2 is about 30 mm Hg and there is 13 vol% of oxygen in the blood (Figure 2-45).
Digital Clubbing
Digital clubbing is sometimes noticed in patients with chronic respiratory disorders. Clubbing is characterized by a bulbous swelling of the terminal phalanges of the fingers and toes. The contour of the nail becomes rounded both longitudinally and transversely, which results in an increase in the angle between the surface of the nail and the terminal phalanx (Figure 2-46).
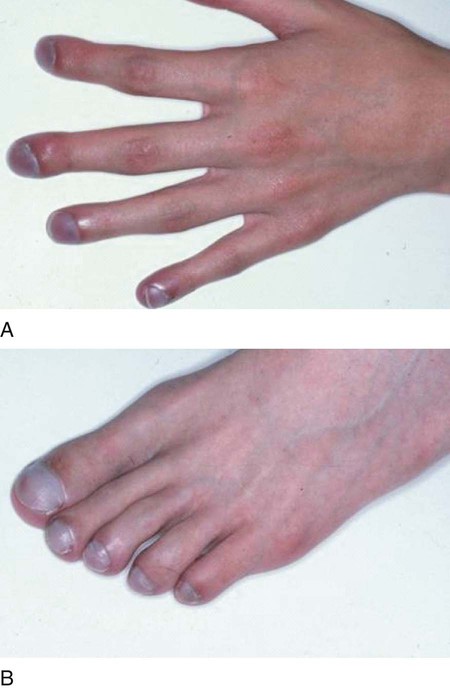
Peripheral Edema
Bilateral, dependent, pitting edema is commonly seen in patients with congestive heart failure, cor pulmonale, and hepatic cirrhosis. To assess the presence and severity of pitting edema, the health-care practitioner places a finger or fingers over the tibia or medial malleolus (2 to 4 inches above the foot), firmly depresses the skin for 5 seconds, and then releases. Normally, this procedure leaves no indentation, although a pit may be seen if the person has been standing all day or is pregnant. If pitting is present, it is graded on the following subjective scale: 1+ (mild, slight depression) to 4+ (severe, deep depression) (Figure 2-47).
Distended Neck Veins
In patients with cor pulmonale, severe flail chest, pneumothorax, or pleural effusion, the major veins of the chest that return blood to the right side of the heart may be compressed. When this happens, venous return decreases and central venous pressure (CVP) increases. This condition is manifested by distended neck veins (also called jugular venous distention; Figure 2-48). The reduced venous return also may cause the patient’s cardiac output and systemic blood pressure to decrease. In severe cases the veins over the entire upper anterior thorax may be dilated.
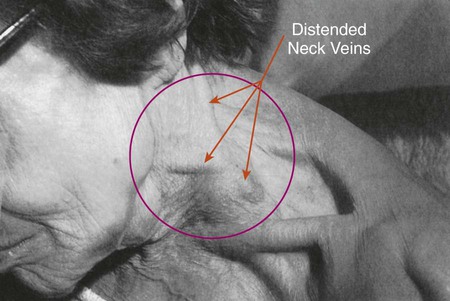
Normal and Abnormal Sputum Production
Normal Histology and Mucous Production of the Tracheobronchial Tree
The wall of the tracheobronchial tree is composed of three major layers: an epithelial lining, the lamina propria, and a cartilaginous layer (Figure 2-49).
The epithelial lining, which is separated from the lamina propria by a basement membrane, is predominantly composed of pseudostratified, ciliated, columnar epithelium interspersed with numerous mucus-secreting glands and serous cells. The ciliated cells extend from the beginning of the trachea to—and sometimes including—the respiratory bronchioles. As the tracheobronchial tree becomes progressively smaller, the columnar structure of the ciliated cells gradually decreases in height. In the terminal bronchioles the epithelium appears more cuboidal than columnar. These cells flatten even more in the respiratory bronchioles (see Figure 2-49).
A mucous layer, commonly referred to as the mucous blanket, covers the epithelial lining of the tracheobronchial tree (Figure 2-50). The viscosity of the mucous layer progressively increases from the epithelial lining to the inner luminal surface and has two distinct layers: (1) the sol layer, which is adjacent to the epithelial lining, and (2) the gel layer, which is the more viscous layer adjacent to the inner luminal surface. The mucous blanket is 95% water. The remaining 5% consists of glycoproteins, carbohydrates, lipids, DNA, some cellular debris, and foreign particles.
The cartilaginous structures that surround the tracheobronchial tree progressively diminish in size as the airways extend into the lungs. The cartilaginous layer is completely absent in bronchioles less than 1 mm in diameter (see Figure 2-49).
Abnormal Sputum Production
Excessive sputum production is commonly seen in respiratory diseases that cause an acute or chronic inflammation of the tracheobronchial tree (see Figure 11-1). Depending on the severity and nature of the respiratory disease, sputum production may take several forms. For example, during the early stages of tracheobronchial tree inflammation, the sputum is usually clear, thin, and odorless. As the disease intensifies, the sputum becomes yellow-green and opaque. The yellow-green appearance results from an enzyme (myeloperoxidase) that is released during the cellular breakdown of leukocytes. It may also be caused by retained or stagnant secretions or secretions caused by an acute infection.
Clinically, hemoptysis may be confused with hematemesis, which is blood that originates from the upper gastrointestinal tract and usually has a dark, coffee-ground appearance. Repeated expectoration of blood-streaked sputum is seen in chronic bronchitis, bronchiectasis, cystic fibrosis, pulmonary embolism, lung cancer, necrotizing infections, tuberculosis, and fungal diseases. A small amount of hemoptysis is common after bronchoscopy, particularly when biopsies are performed. Massive hemoptysis is defined as coughing up 400 to 600 mL of blood within a 24-hour period. Death from exsanguination resulting from hemoptysis is rare. Table 2-11 provides a general overview and analysis of the types of sputum commonly seen in the clinical setting.
Table 2-11
Color | Indications and Conditions |
Brown/dark | Old blood |
Bright red (hemoptysis) | Fresh blood (bleeding tumor, tuberculosis) |
Clear and translucent | Normal |
Copious | Large amount |
Frank hemoptysis | Massive amount of blood |
Green | Stagnant sputum or gram-negative bacteria |
Green and foul smelling | Pseudomonas or anaerobic infection |
Mucoid (white/gray) | Asthma, chronic bronchitis |
Pink, frothy | Pulmonary edema |
Tenacious | Secretions that are sticky or adhesive or otherwise tend to hold together |
Viscous | Thick, viscid, sticky, or glutinous |
Yellow or opaque | Presence of white blood cells, bacterial infection |
Cough
Although a cough may be voluntary, it is usually a reflex response that arises when an irritant stimulates the irritant receptors (also called subepithelial mechanoreceptors). The irritant receptors are located in the pharynx, larynx, trachea, and large bronchi. When stimulated, the irritant receptors send a signal by way of the glossopharyngeal nerve (cranial nerve IX) and vagus nerve (cranial nerve X) to the cough reflex center located in the medulla. The medulla then causes the glottis to close and the accessory muscles of expiration to contract. Box 2-5 lists common factors that stimulate the irritant receptors.
Productive Cough
When the cough is productive, the respiratory practitioner should assess the following:
• Is the cough strong or weak? In other words, does the patient have a good or poor ability to mobilize bronchial secretions? A good, strong cough may indicate only deep breath and cough therapy, whereas an inadequate cough may indicate chest physical therapy or postural drainage.
• A productive cough should be evaluated in terms of its frequency, pitch, and loudness. A brassy cough may indicate a tumor, whereas a barking or hoarse cough indicates croup.
• Finally, the sputum of a productive cough should be monitored and evaluated continuously in terms of amount (teaspoons, tablespoons, cups), consistency (thin, thick, tenacious), odor, and color (see Table 2-11).