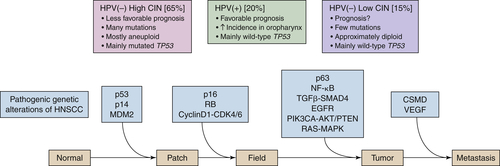
Table 33-1
Common Genetic Alterations in HNSCC
Gene ∗ | Description | Mutated (Activating/Missense/Inactivating) |
TP53 a,b | Transcription factor | 53% (NA/28%/25%) |
CCND1 b | Cell cycle activator (Cyclin D1) | 25% (25%/NA/NA) |
CDKN2A a,b | Cell cycle inhibitor/p53 activator | 21% (NA/3%/18%) |
NOTCH1 a,b, ∗ | Receptor/transcription factor | 15% (NA/8%/8%) |
CSMD3 a,b | Putative adhesion factor | 13% (NA/12%/1%) |
USH2A a,b | Basement membrane protein | 12% (NA/11%/1%) |
PIK3CA a,b, ∗ | PI3 kinase catalytic subunit | 10% (7%/7%/NA) |
PRDM9 b,∗ | Histone methyltransferase | 8% (NA/8%/1%) |
COL22A1 b, ∗ | Pro-apoptotic effector | 8% (NA/7%/2%) |
RIMS2 b, ∗ | Putative synaptic vesicle regulator | 8% (NA/8%/NA) |
ZFHX4 b | Zinc finger homeodomain | 8% (NA/8%/NA) |
MLL2 b, ∗ | Histone methyltransferase | 8% (NA/4%/4%) |
NAV3 b | Axonal/cytoskeleton guide | 8% (NA/7%/1%) |
CASP8 a.b | Pro-apoptotic proteolyase | 7% (NA/2%/5%) |
TP63 a,b | Transcription factor | 7% (NA/5%/1%) |
NSD1 b | Histone methyltransferase | 7% (NA/3%/4%) |
EGFR b | Growth factor Rtk | 7% (7%/NA/NA) |
PTEN b | Lipid phosphatase—PI3K inhibitor | 6% (NA/3%/3%) |
FBXW7 a, ∗ | Ubiquitin ligase | 5% (NA/3%/2%) |
HRAS a,b,∗ | RTK signaling protein | 4% (4%/4%/NA) |
IRF6 b | Interferon regulatory factor | 4% (NA/3%/1%) |
NOTCH2 b | Receptor/transcription factor | 4% (NA/3%/1%) |
NOTCH3 b, ∗ | Receptor/transcription factor | 4% (NA/2%/2%) |
Displays the percentage of samples in the aAgrawal et al. 15 and bStranksy et al. 16 exome sequencing studies with at least one mutant allele or copy number loss/amplification of a given gene. It also identifies the percentage of each respective mutation that is activating (known activating mutation or copy number amplification), missense, and inactivating (nonsense, splice site, frame shift, insertion/deletion, or copy number loss).
∗ Mutations present in at least one HPV(+) tumor.
Molecular Pathogenesis of HNSCC: Interfacing Genomic Pathways
Cell Cycle and Proliferation: TP53/CDKN2A/RB/CCND1/TERT
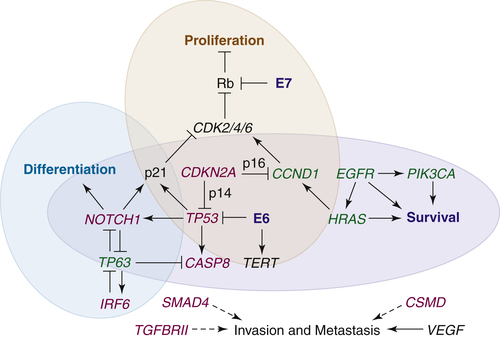
Apoptosis and Survival: EGFR/RAS-MAPK/PIK3CA-AKT/CASP8
Differentiation and Mesenchymal Transition: NOTCH/TP63
Invasion and Metastasis: MMP/TGFβ-SMAD/NFκB/CSMD/VEGF
Future Directions
1. Cancer of the head and neck is the sixth most common cancer worldwide . Curr Probl Surg . 2009 ; 46 : 114 – 117 .
2. Estimates of worldwide burden of cancer in 2008: GLOBOCAN 2008 . Int J Cancer . 2010 ; 127 : 2893 – 2917 .
3. . Cancer Facts and Figures 2012 . Surveillance Research ; 2012 : Retrieved from: http://www.cancer.org/research/cancerfactsfigures/cancerfactsfigures/cancer-facts-figures-2012 .
4. The molecular biology of head and neck cancer . Nat Rev Cancer . 2011 ; 11 : 9 – 22 .
5. Human papillomavirus-associated cancers—United States, 2004-2008 . MMWR Morb Mortal Wkly Rep . 2012 ; 61 : 258 – 261 .
6. Human papillomavirus and survival of patients with oropharyngeal cancer . N Engl J Med . 2010 ; 363 : 24 – 35 .
7. Carcinoma of the head and neck in the HPV era . Acta Dermatovenerol Alp Panonica Adriat . 2011 ; 20 : 161 – 173 .
8. Deficiencies in the Fanconi anemia DNA damage response pathway increase sensitivity to HPV-associated head and neck cancer . Cancer Res . 2010 ; 70 : 9959 – 9968 .
9. Acute or chronic life-threatening diseases associated with Epstein-Barr virus infection . Am J Med Sci . 2012 ; 343 : 483 – 489 .
10. AJCC Cancer Staging Manual . 7th ed. New York, NY : Springer Verlag ; 2009 .
11. Radiotherapy plus cetuximab for squamous-cell carcinoma of the head and neck . N Engl J Med . 2006 ; 354 : 567 – 578 .
12. Platinum-based chemotherapy plus cetuximab in head and neck cancer . N Engl J Med . 2008 ; 359 : 1116 – 1127 .
13. Proteomic analysis reveals successive aberrations in protein expression from healthy mucosa to invasive head and neck cancer . Oncogene . 2007 ; 26 : 54 – 64 .
14. TP53 mutations in human cancers: origins, consequences, and clinical use . Cold Spring Harb Perspect Biol . 2010 ; 2 a001008 .
15. Exome sequencing of head and neck squamous cell carcinoma reveals inactivating mutations in NOTCH1 . Science . 2011 ; 333 : 1154 – 1157 .
16. The mutational landscape of head and neck squamous cell carcinoma . Science . 2011 ; 333 : 1157 – 1160 .
17. Hallmarks of cancer: the next generation . Cell . 2011 ; 144 : 646 – 674 .
18. TP53 mutations and survival in squamous-cell carcinoma of the head and neck . N Engl J Med . 2007 ; 357 : 2552 – 2561 .
19. Dysregulated molecular networks in head and neck carcinogenesis . Oral Oncol . 2009 ; 45 : 324 – 334 .
20. p53 in health and disease . Nat Rev Mol Cell Biol . 2007 ; 8 : 275 – 283 .
21. Differential roles of p16INK4A and p14ARF genes in prognosis of oral carcinoma . Cancer Epidemiol Biomarkers Prev . 2008 ; 17 : 414 – 420 .
22. Evaluation of a combined triple method to detect causative HPV in oral and oropharyngeal squamous cell carcinomas: p16 immunohistochemistry, consensus PCR HPV-DNA, and in situ hybridization . Infect Agent Cancer . 2012 ; 7 : 4 .
23. Prognostic significance of cyclin D1 and p16 in patients with intermediate-risk head and neck squamous cell carcinoma treated with docetaxel and concurrent radiotherapy . Head Neck . 2007 ; 29 : 940 – 947 .
24. Cyclin D as a therapeutic target in cancer . Nat Rev Cancer . 2011 ; 11 : 558 – 572 .
25. Targeting PI3K signalling in cancer: opportunities, challenges and limitations . Nat Rev Cancer . 2009 ; 9 : 550 – 562 .
26. Loss of TGF-βsignaling and PTEN promotes head and neck squamous cell carcinoma through cellular senescence evasion and cancer-related inflammation . Oncogene . 2012 ; 31 3322-3232 .
27. A continuum model for tumour suppression . Nature . 2011 ; 476 : 163 – 169 .
28. Oncogenic mutations of the PIK3CA gene in head and neck squamous cell carcinomas . Int J Oncol . 2008 ; 32 : 101 – 111 .
29. PIK3CA-mediated PI3-kinase signalling is essential for HPV-induced transformation in vitro . Mol Cancer . 2011 ; 10 : 71 .
30. Targeted therapy in head and neck cancer . Tumour Biol . 2012 ; 33 : 707 – 721 .
31. RAS interaction with PI3K: more than just another effector pathway . Genes Cancer . 2011 ; 2 : 261 – 274 .
32. Mouse models for human head and neck squamous cell carcinomas . Head Neck . 2006 ; 28 : 945 – 954 .
33. A critical role of MYC for transformation of human cells by HPV16 E6E7 and oncogenic HRAS . Carcinogenesis . 2012 ; 33 : 910 – 917 .
34. Inhibition of Ras for cancer treatment: the search continues . Future Med Chem . 2011 ; 3 : 1787 – 1808 .
35. Epidermal growth factor receptor targeted therapy of squamous cell carcinoma of the head and neck . Head Neck . 2010 ; 32 : 1412 – 1421 .
36. Prognostic significance of epidermal growth factor receptor phosphorylation and mutation in head and neck squamous cell carcinoma . Oncologist . 2009 ; 14 : 900 – 908 .
37. Mutant epidermal growth factor receptor (EGFRvIII) contributes to head and neck cancer growth and resistance to EGFR targeting . Clin Cancer Res . 2006 ; 12 : 5064 – 5073 .
38. Clinical significance of genetic alterations and expression of epidermal growth factor receptor (EGFR) in head and neck squamous cell carcinomas . Oral Oncol . 2011 ; 47 : 487 – 496 .
39. Lyn kinase mediates cell motility and tumor growth in EGFRvIII-expressing head and neck cancer . Clin Cancer Res . 2012 ; 18 : 2850 – 2860 .
40. The MET receptor tyrosine kinase is a potential novel therapeutic target for head and neck squamous cell carcinoma . Cancer Res . 2009 ; 69 : 3021 – 3031 .
41. Genotypic and histological evolution of lung cancers acquiring resistance to EGFR inhibitors . Sci Transl Med . 2011 ; 3 75ra26 .
42. Anaplastic lymphoma kinase inhibition in non-small-cell lung cancer . N Engl J Med . 2010 ; 363 : 1693 – 1703 .
43. Monovalency unleashes the full therapeutic potential of the DN-30 anti-Met antibody . J Biol Chem . 2010 ; 285 : 36149 – 36157 .
44. p63 mediates survival in squamous cell carcinoma by suppression of p73-dependent apoptosis . Cancer Cell. J . 2006 ; 9 : 45 – 56 .
45. p63 is a p53 homologue required for limb and epidermal morphogenesis . Nature . 1999 ; 398 : 708 – 713 .
46. p63 is essential for regenerative proliferation in limb, craniofacial and epithelial development . Nature . 1999 ; 398 : 714 – 718 .
47. DeltaNp63alpha repression of the Notch1 gene supports the proliferative capacity of normal human keratinocytes and cervical cancer cells . Cancer Res . 2010 ; 70 : 4034 – 4044 .
48. Notch, apoptosis and cancer . Adv Exp Med Biol . 2012 ; 727 : 199 – 209 .
49. Gain-of-function mutations and copy number increases of Notch2 in diffuse large B-cell lymphoma . Cancer Sci . 2009 ; 100 : 920 – 926 .
50. Whole-genome sequencing identifies recurrent mutations in chronic lymphocytic leukaemia . Nature . 2011 ; 475 : 101 – 105 .
51. Activating mutations of NOTCH1 in human T cell acute lymphoblastic leukemia . Science . 2004 ; 306 : 269 – 271 .
52. Notch tumor suppressor function . Oncogene . 2008 ; 27 : 5115 – 5123 .
53. Notch1 functions as a tumor suppressor in mouse skin . Nat Genet . 2003 ; 33 : 416 – 421 .
54. Exclusive development of T cell neoplasms in mice transplanted with bone marrow expressing activated Notch alleles . J Exp Med . 1996 ; 183 : 2283 – 2291 .
55. Matrix metalloproteases in head and neck cancer . Head Neck . 2006 ; 28 : 639 – 648 .
56. TGFbeta signalling: a complex web in cancer progression . Nat Rev Cancer . 2010 ; 10 : 415 – 424 .
57. Smad4 loss in mice causes spontaneous head and neck cancer with increased genomic instability and inflammation . J Clin Invest . 2009 ; 119 : 3408 – 3419 .
58. Disruption of transforming growth factor beta-Smad signaling pathway in head and neck squamous cell carcinoma as evidenced by mutations of SMAD2 and SMAD4 . Cancer Lett . 2007 ; 245 : 163 – 170 .
59. Mutation and downregulation of the transforming growth factor beta type II receptor gene in primary squamous cell carcinomas of the head and neck . Carcinogenesis . 1997 ; 18 : 2285 – 2290 .
60. Attenuated transforming growth factor beta signaling promotes nuclear factor-kappaB activation in head and neck cancer . Cancer Res . 2009 ; 69 : 3415 – 3424 .
61. TGF-β and NF-κB signal pathway cross-talk is mediated through TAK1 and SMAD7 in a subset of head and neck cancers . Oncogene . 2013 ; 32 : 1549 – 1559 .
62. Prognostic significance of vascular endothelial growth factor in squamous cell carcinomas of the tonsil in relation to human papillomavirus status and epidermal growth factor receptor . Ann Surg Oncol . 2009 ; 16 : 2908 – 2917 .
63. Loss of CSMD1 expression is associated with high tumour grade and poor survival in invasive ductal breast carcinoma . Breast Cancer Res Treat . 2010 ; 121 : 555 – 563 .
64. A miR-centric view of head and neck cancers . Biochim Biophys Acta . 2011 ; 1816 : 67 – 72 .
65. Down-regulation of the microRNA-99 family members in head and neck squamous cell carcinoma . Oral Oncol . 2012 ; 48 : 686 – 691 .
66. The role of miRNAs in human papilloma virus (HPV)-associated cancers: bridging between HPV-related head and neck cancer and cervical cancer . Br J Cancer . 2012 ; 106 : 1526 – 1534 .
67. Delineating an epigenetic continuum in head and neck cancer . Cancer Lett . 2012 [Epub ahead of print] .
68. Genome-wide methylation and expression differences in HPV(+) and HPV(-) squamous cell carcinoma cell lines are consistent with divergent mechanisms of carcinogenesis . Epigenetics . 2011 ; 6 : 777 – 787 .
69. Loss of heterozygosity at 9p and p53 immunopositivity in surgical margins predict local relapse in head and neck squamous cell carcinoma . Int J Cancer . 2011 ; 128 : 1852 – 1859 .
70. Differential proteomics identifies protein biomarkers that predict local relapse of head and neck squamous cell carcinomas . Clin Cancer Res . 2009 ; 15 : 7666 – 7675 .