13 The brainstem and reticular formation
Case 13.2
Questions
• 13.2.1 Discuss the difference between upper and lower motor neuron lesions in general.
• 13.2.2 Discuss the different clinical signs when differentiating between an upper and a lower motor neuron lesion involving the facial nerve.
• 13.2.3 Discuss six differential diagnostic considerations for this gentleman.
Anatomy of the brainstem
The brainstem is composed of the following anatomical areas (Figs 13.1, 13.2, and 13.3):
1. Midbrain or mesencephalon is contained between the cerebrum and the pons in an area which measures approximately 2.5 cm long;
2. Pons is contained between the midbrain and the medulla;
3. Medulla oblongata extends from the base of the pons to the first pair of cervical nerves. Caudally, the medulla is continuous with the medulla spinalis or the spinal cord.
We will approach the description of the brainstem by outlining the structures observed at various levels in cross-sectional dissections.
Input from primary afferents and their collaterals results in the following
1. Monosynaptic reflex involving the alpha motor neurons
4. Inhibition of contralateral homologues
5. Excitation of antagonist of contralateral homologues
7. Excitation of granular layer of the cerebellum, which leads to increased contralateral cortical integration via excitation of contralateral thalamus.
Mesencephalon
The structures of the mesencephalon can be observed in a cross-section of midbrain at the superior colliculus and the inferior colliculus (Figs 13.4 and 13.5).
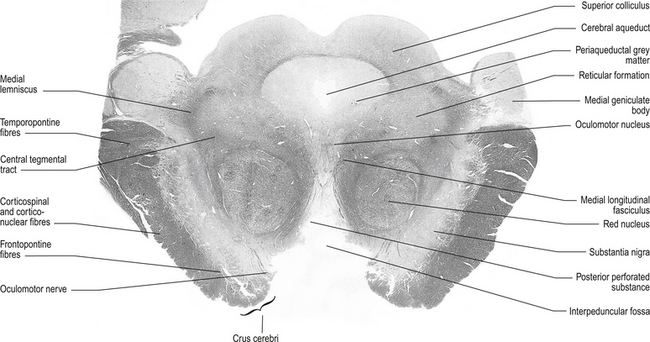
Figure 13.4 A cross-sectional view of the mesencephalon at the superior colliculus.
(from Standring 2008 Gray’s Anatomy 40th edn. Edinburgh, Elsevier, with permission)
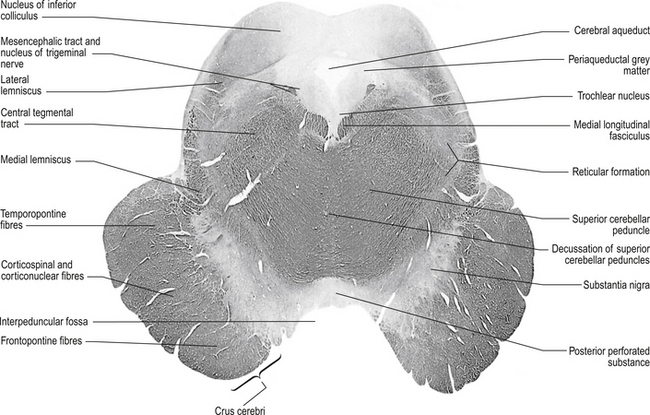
Figure 13.5 A cross-sectional view of the mesencephalon at the inferior colliculus.
(from Standring 2008 Gray’s Anatomy 40th edn. Edinburgh, Elsevier, with permission)
Tectum
The tectum comprises the ‘roof’ or dorsal portion of the midbrain and contains the corpora quadrigemina, which includes the superior and inferior colliculi and all the substances that lie dorsal to the cerebral aqueduct in the midbrain area of the neuraxis. The superior colliculi are involved with visual reflexes, and project to the lateral geniculate bodies of the thalamus. The inferior colliculi are involved with reflexes associated with sound, and project to the medial geniculate bodies of the thalamus.
Red nucleus
These bilateral structures are ovoid groups of nuclei composed of two different cell types, the magnocellular and parvocellular groups of neurons. The magnocellular neurons are large, multipolar cells located in the caudal area of the red nuclear mass. These neurons receive bilateral projections both from sensorimotor cortical areas via the corticorubral tracts and from collaterals via the corticospinal tracts. The cortical projections and their target neurons in the red nucleus are somatotopically organised. Axon projections from the magnocellular neurons form the rubrospinal tracts, which cross in the brainstem and project in a somatotopically organised fashion, mainly to the interneurons of the intermediate grey areas of the spinal cord. Some rubrospinal fibres terminate directly on ventral horn motor neurons as well. Some axons that form the rubrospinal tracts terminate on neurons in the pontomedullary reticular formation and the motor nuclei of various cranial nerves, forming the rubroreticular system and the rubrobulbar tracts, respectively (Brown 1974). Reciprocal, bilateral projections to the superior colliculi are also present and form the rubrotectal tracts (Fig. 13.6). The rubrospinal and corticospinal tracts form the lateral motor system of the spinal cord. The medial motor system is composed of the reticulospinal and vestibulospinal tracts.
The parvocellular neurons of the red nucleus are small pyramidal- and spherical-shaped neurons, mostly located in the rostral areas of the red nuclear mass. These neurons receive projections from the dentate nucleus of the contralateral cerebellum, and from the ipsilateral globus pallidus pars externa, substantia nigra, and subthalamic nuclei. These neurons project to the ipsilateral thalamus (Fig. 13.6).
Medial longitudinal fasciculus (MLF)
This structure is a highly myelinated axon tract that descends from the interstitial nucleus of Cajal in the lateral wall of the third ventricle through the midbrain, pons, and medulla to the spinal cord where it becomes continuous with the anterior intersegmental fasciculus. The MLF acts as a major communications conduit between all of the cranial nerve nuclei and all related structures including the reticular formations of the mesencephalon, pons, and medulla. The medial vestibulospinal tract axons project with the MLF to the spinal cord (Brodal et al. 1962). Other axons from the vestibular nuclei ascend in the MLF to more rostral structures including the extraocular cranial nuclei. The MLF is the first fibre tract in the developing embryo to become myelinated and probably acts as a major pathway providing stimulus to developing neurons in the early stages of embryonic development.
Medial lemnisci
The medial lemniscus is a bundle of axons that forms a triangular structure medial to the spinothalamic tract. The bundles are formed from axons of the contralateral dorsal column nuclei, the nucleus gracilis and cuneatus, which have decussated and formed the internal arcuate fibres which become continuous with the medial lemnisci. The fibres are joined by axons of the trigeminal sensory nucleus to project to the ipsilateral thalamus. Axons from the dorsal column nuclei terminate on neurons in the ventral posterior lateral nucleus of the thalamus, whereas axons from the trigeminal sensory nucleus terminate on neurons in the ventral posterior medial nucleus of the thalamus (Guyton & Hall 1996).
Pons
The structures of the pons can be observed through a cross-section at the level of the trigeminal nerves (Fig. 13.7), just superior to the cerebral peduncles.
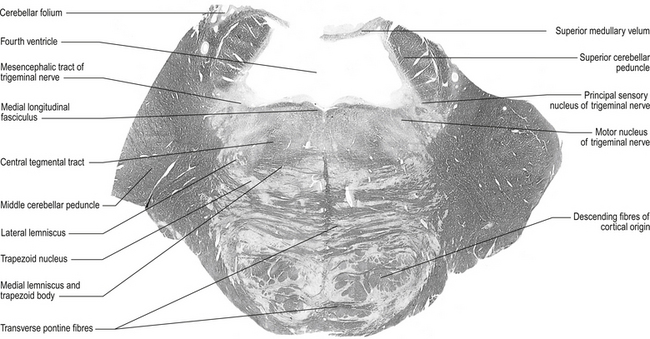
Figure 13.7 A cross-sectional view of the pons at the level of the trigeminal nerves.
(from Standring 2008 Gray’s Anatomy 40th edn. Edinburgh, Elsevier, with permission)
Superior cerebellar peduncle
1. Dentatorubral and dentatothalamic fibres, both of which terminate contralaterally in the red nucleus and the thalamus, respectively;
2. Fibres of the ventral or anterior spinocerebellar tract projecting to the cerebellum from the spinal cord; and
3. Fibres of the uncinate fasciculus that contains fibres from the fastigial nucleus that will terminate in the lateral vestibular nucleus (Chusid 1982).
Lateral lemnisci
This tract carries fibres from the contralateral dorsal cochlear nucleus to the inferior colliculus.
Fourth ventricle
This cavity is bounded by the pons and medulla ventrally and by the cerebellum dorsally. It is continuous with the cerebral aqueduct above and the central canal of the medulla below and has a capacity for cerebrospinal fluid (CSF) of about 20 ml. The floor of the fourth ventricle, which is also referred to as the rhomboid fossa, is formed by the dorsal surfaces of the pons and medulla. The fourth ventricle acts as a component of the CSF system (Chusid 1982).
Medulla
Structures of the medulla can be viewed by cross-sections at:
Structures found at a cross-section at the inferior olive (fig. 13.8)
Inferior cerebellar peduncle
1. Olivocerebellar tract, which arises from neurons of the contralateral superior olivary nucleus and projects to the cerebellar hemispheres and vermis;
2. Dorsal spinocerebellar tract, which arises from neurons in the area of Clark’s column of the spinal cord to project to the interpositus nuclear region and the palaeocerebellar cortex;
3. Dorsal external arcuate fibres from the nuclei gracilis and cuneatus;
4. Ventral external arcuate fibres from the lateral reticular nuclei of the medulla; and
5. Vestibulocerebellar tract, which arises from the vestibular nuclei and projects to the flocculonodular lobe of the cerebellum.
Lateral spinothalamic tract
These tracts are located medial and anterior to the ventral spinocerebellar tracts in the ventral lateral fasciculus of the spinal cord. They are formed by axons projecting from neurons located in the contralateral dorsal horn area. These neurons project their axons via the anterior white commissure to the opposite lateral spinothalamic tract where they terminate on the ipsilateral thalamus to the tract in which they ascend.
Inferior olivary nucleus
These bilateral groups of nuclei are located within the olive of the medulla. They receive projections from the cortex, from other brainstem nuclei, from the ipsilateral parvocellular red nucleus, and from the ipsilateral spinal cord. These neurons project axons, referred to as climbing fibres, via the inferior cerebellar peduncles to all areas of the cerebellar cortex. These structures are part of a group of projections and nuclei referred to as the inferior olivary nuclear complex, which forms a complicated loop from the cerebellar cortex to the dentate nucleus to the contralateral red nucleus to the inferior olivary nucleus and back to the contralateral cerebellar cortex (Fig. 13.9)
Solitary tract nucleus
These nuclei are also referred to as the nuclei of the tractus solitarius (NTS). These nuclei are located ventrolaterally to the motor nuclei of the vagus nerve and run the full length of the medulla. All cranial visceral afferent nerves project to the NTS. The rostral portion of the NTS, which is referred to as the gustatory nucleus, receives projections from the special visceral afferent nerves (CN VII, CN IX, CN X) for taste. The caudal portion of the NTS, which is referred to as the cardiorespiratory nucleus, receives projections from the general visceral afferent fibres of CN IX and CN X.
Structures found in a cross-section at the lemniscal decussation (fig. 13.10)
Fasciculus gracilis
These bilateral tracts run dorsally in the medulla medial to the cuneate fasciculi. They are formed by the axons of the dorsal root ganglion cells that detect proprioception and touch in the lower limbs and trunk, usually below the T6 level. The axons enter the spinal cord via the dorsal root and ascend ipsilaterally in the fasciculus gracilis to the gracile nucleus.
Structures found at a cross-section through pyramidal decussation (fig. 13.11)
Anterior corticospinal tracts
These bilateral tracts are contained in the pyramids of the medulla until the decussation of the pyramids where they do not decussate but continue ipsilaterally in the ventral medial portion of the ventral fasciculus of the spinal cord. These tracts relay motor information to the muscles of the trunk, spine, and proximal limbs.
The reticular formation (RF)
The reticular formation receives little attention in traditional neurology textbooks. It is an area that spans all levels of the brainstem, from the thalamus to the spinal cord, and is responsible for integrating information from the brain and periphery and linking sensory, motor, and autonomic nuclei of the brainstem. The reticular formation therefore mediates complex reflexes and functions such as eye movements, posture, feeding, breathing, homeostasis, arousal, sleep, control of vasomotor tone and cardiac output, and pain. The reticular formation is composed of continuous groups of neurons interconnected via polysynaptic pathways that can be both crossed and uncrossed in nature. The RF receives projections from virtually all sensory modalities and projects to all areas of the neuraxis including direct projections to the cortex (Webster 1978).
Afferent projections to the RF include:
Efferent projections from the RF include:
• Projections to autonomic nuclei and intermediolateral (IML) column;
• Direct projections to the thalamus and cortex; and
• Direct projections to other areas of the reticular system.
Anatomy of the reticular formation
The neurons of the reticular formation form multiple interconnecting patterns that resemble a fish net; hence the name reticular, which means net-like. The neurons are located centrally in the neuraxis. The neurons can be roughly grouped into three columns based on their size. The median column is located most centrally and is composed of intermediate-sized neurons. The medial column, which is just lateral to the median column, contains relatively large neurons. The lateral column is located most laterally and contains relatively small neurons (Fig. 13.12).
The neurons of the RF columns can be grouped into various nuclei which include the following (Fig. 13.12):
Reticular neurons projection systems utilise different neurotransmitters
Nuclear groups can also be identified based on the neurotransmitter that they release. Several projection systems have been discussed in detail in Chapter 9. Only those related to the reticular formation will be described here.
The mesocortical projection pathway arises from neurons in the ventral tegmental and substantia nigral areas of the midbrain and terminates in widespread areas of prefrontal cortex. The projections seem to favour motor cortex and association cortical areas over sensory and primary motor areas (Fallon & Loughlin 1987).
The noradrenergic projection system consists of neurons in two different locations in the rostral pons and the lateral tegmental area of the pons and medulla. The neurons in the rostral pons area are referred to as the locus ceruleus and together with the neurons in the lateral tegmental area of the pons and medulla project to all areas of the entire forebrain including the limbic areas as well as to the cerebellum, brainstem, and spinal cord.
The serotonergic projection system consists of a group of nuclei in the midbrain pons and medulla referred to as the raphe nuclei and additional groups of neurons in the area postrema and caudal locus ceruleus. These nuclei can be divided into rostral and caudal groups. The rostral raphe nuclei project ipsilaterally via the median forebrain bundle to the entire forebrain where serotonin can act as either excitatory or inhibitory in nature, depending on the situation (Fallon & Loughlin 1987). The caudal raphe nuclei project to the cerebellum, medulla, and spinal cord.
Functions of the reticular formation
Functions of the reticular formation include the following:
1. Modulation of motor control—The RF can modulate the activation levels of both alpha and gamma motor neurons, and thus alter the tone and reflex activity of muscle. The RF is particularly involved with reciprocal inhibition of antagonist muscles and in the maintenance of muscle tone in antigravity muscles. Motor activity of the facial muscles associated with an emotional response is mediated by the RF. These pathways are independent of the corticobulbar tracts to the cranial nerve nuclei, and thus a person with a corticobulbar stroke can still smile symmetrically when stimulated emotionally. The mesencephalic reticular formation (MRF) is responsible for increasing flexor tone on the contralateral side. The pontomedullary reticular formation (PMRF) is responsible for the inhibition of ipsilateral anterior (flexor) muscles above T6, and the inhibition of ipsilateral posterior (flexor) muscles below T6.
2. Modulation of somatic and visceral sensations—The RF has the capacity to modulate all somatic and visceral sensations including pain. The PMRF in particular modulates the inhibition of pain.
3. Modulation of the autonomic nervous system—The RF is also involved with modulation of the activity of both sympathetic and parasympathetic functions of the autonomic nervous system. Activation of the MRF results in excitation of the preganglionic sympathetic neurons of the IML bilaterally. Activation of the PMRF results in inhibition of the ipsilateral preganglionic neurons of the IML.
4. Modulation of pituitary hormones—The RF, through both direct and indirect pathways, modulates the output of releasing factors from the hypothalamus, thus modulating the release of pituitary hormones. The RF also influences the hypothalamic circadian and biological rhythm patterns.
5. Modulation of reticular activation system—The RF is also involved in the maintenance and level of consciousness through direct projections to wide areas of cortex. This projection system is referred to as the reticular activation system.
Importantly, the relationship between the spine, vestibular system, midline cerebellum, cortex, limbic system, and the autonomic nervous system can be seen intimately in this region and may influence immune function and behavioural characteristics such as fear, anxiety, panic, mood, disinhibition, sleep, arousal, and risk taking.
Functional systems and clinical implications of the reticular system dysfunction
The cortex projects to excite the MRF bilaterally and the PMRF ipsilaterally. The MRF then projects as described above to excite the IML and the flexor muscle groups bilaterally. Loss of functional integrity of the MRF would result in a decreased activation of the sympathetic nervous system bilaterally and decreased flexor tone bilaterally (Fig. 13.13).
The PMRF projects to inhibit the IML and flexor groups ipsilaterally (Fig. 13.14). Decreased PMRF integrity may therefore result in the following clinically relevant signs:
Cranial nerves
The cranial nerves, with the exception of the olfactory (CN I) and optic (CN II), all arise from nuclei in the brainstem (Figs 13.15 and 13.16).
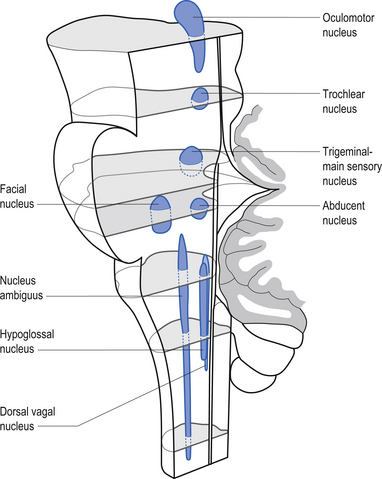
Figure 13.15 A lateral view of the anatomical position and relationships of the cranial nerve nuclei.
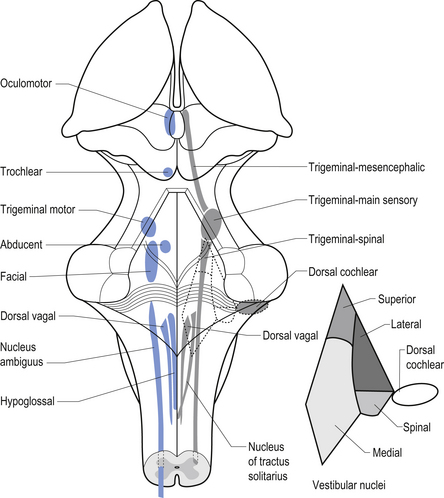
Figure 13.16 A posterior view of the anatomical position and relationships of the cranial nerve nuclei.
Olfactory nerve (CN I)
Olfactory epithelium is bathed in lipid substance produced by Bowman’s glands. Primary afferents synapse with mitral cells of the olfactory glomerulus after passing unmyelinated through the cribriform plate. Axons of mitral cells make up the olfactory nerve (Fig. 13.17).
The main tract has both centrifugal and centripetal fibres that help modulate receptor activity and enhance variety of perceived odours. The tract passes above the optic nerve and chiasm below the frontal lobes.
The tract terminates in three striae which synapse with neurons of the olfactory tubercle and gyrus, medial amygdaloid nucleus, and other limbic regions. The secondary olfactory cortex, along with the hypothalamus, mediates emotional and autonomic reflexes associated with smell (Moore 1980; Wilson-Pauwels et al. 1988) (Fig. 13.18).
Optic nerve (CN II)
The visual image inverts and reverses as it passes through the lens of the eye and forms an image on the retina. The image from the upper visual field is projected on the lower retina and the lower visual field on the upper retina. The left visual field is projected to the right hemiretina of each eye in such a fashion that the right nasal hemiretina of the left eye and the temporal hemiretina of the right eye receive the image. The central image or focal point of the visual field falls on the fovea of the retina, which is the portion of the retina with the highest density of retinal cells and as such produces the highest visual acuity. The fovea receives the corresponding image of the central 1°–2° of the total visual field but represents about 50% of the axons in the optic nerve and projects to about 50% of the neurons in the visual cortex. The macula comprises the space surrounding the fovea and also has a relatively high visual acuity (Fig. 13.19).
The rods and cones are the primary receptors of light stimulation and are located at the deepest point on the retina adjacent to the pigment epithelial cells. They synapse with bipolar cells, which in turn synapse with the ganglion cells. The ganglion cells can be further classified as M cells, which have large receptive fields and respond best to movement, or P cells, which have small receptive fields and respond best to fine detail and colour (Fig. 13.20). Injury or dysfunction at any point along the optic nerves, tracts, or radiations can produce characteristic clinical visual field deficits (Fig. 13.21). Clinical testing of the optic nerve includes visual acuity and visual field testing (Moore 1980; Wilson-Pauwels et al. 1988).
Oculomotor nerve (CN III)
These nerves arise from the oculomotor nuclei of the midbrain and course through the red nucleus, exiting the skull through the superior orbit fissure to supply motor innervation of the superior rectus, inferior rectus, and medial rectus muscles of the eye. Parasympathetic fibres from the Edinger–Westphal nucleus also accompany the axons of these nerves to supply the parasympathetic component (Moore 1980, Wilson-Pauwels et al. 1988) (Fig. 13.22).
Trochlear nerve (CN IV)
These nerves arise from the trochlear nuclei located just caudal from the oculomotor nuclei at the level of the inferior colliculi. The axons exit posteriorly and cross in the anterior medullary velum and wind around the cerebral peduncles to exit the skull through the superior orbital fissure and innervate the superior oblique muscles of the eye (Moore 1980; Wilson-Pauwels et al. 1988).
Abducens nerve (CN VI)
These axons arise from nuclei in the floor of the fourth ventricle in the caudal portion of the pons. The axons course through the pons and exit anteriorly to run along the petrous portion of the temporal bone to the outer wall of the cavernous sinus, where the nerve exits the skull through the superior orbital fissure to supply motor innervation to the lateral rectus muscle (Moore 1980; Wilson-Pauwels et al. 1988).
Control of eye movement
In order to understand oculomotor control it is necessary to remember that all eye movements are designed to keep a desired object centred on the fovea, which allows for the greatest visual acuity. In order for the desired object to be clearly visualised, it must be held relatively steady on the fovea, and the two eyes must be simultaneously aligned to within a few minutes of arc (Leigh & Zee 1992). Understanding normal function allows us to have a better understanding of when and why abnormal eye movements occur. The normal tendency of the eyeball is to return to primary position. To hold the eyeball in any other position requires constant contraction of the extraocular muscles in exactly the right proportions.
When the eye moves to a new target it does so by a movement called a saccade, which is a fast, burst-like movement. Saccades can reach velocities of 700° per second and vision is transiently suppressed during saccadic movements. The saccade is programmed with two distinct components, a pulse phase and a step phase. The pulse phase is the burst of action potential activity to move the eye to the new target. The step phase is the new action potential firing rate to maintain the eye in the new position (Fig. 13.23).
Saccades can be horizontal or vertical in nature. The burst phase of activity for a horizontal saccade is generated by burst neurons in the pontine paramedian reticular formation. The duration of firing of a burst neuron begins just before the saccade and ends exactly when the saccade enters the step phase. In between burst outputs, the burst neurons are tonically inhibited by omnipause neurons in the nucleus raphe interpositus (Buttner-Ennever & Buttner 1988). The omnipause neurons continuously discharge, inhibiting the burst neurons until they enter a pause cycle in which the burst neurons become disinhibited and fire a burst of action potentials that results in a saccade motion of the eye until the pause neurons resume their firing and inhibit the burst neurons. The step phase of the horizontal saccade is thought to be created by a neural gaze maintenance network or neural integrator that calculates the saccadic velocity to produce the appropriate position and to produce the appropriate contraction in the extraocular muscles to maintain the gaze at a specific point. The medial vestibular nucleus, the flocculus of the cerebellum, and the nucleus prepositus hypoglossi are important components of the neural integration system of horizontal movements (Zee et al. 1981; Cannon & Robinson 1987) (Fig. 13.24).
The burst phase of activity for a vertical saccade is generated by burst neurons in the rostral interstitial nucleus of the medial longitudinal fasciculus (riMLF) (Buttner-Ennever & Buttner 1988). This nucleus is located ventral to the aqueduct of Sylvius in the prerubral fields at the junction of the mesencephalon and the thalamus. The activity in this nucleus is dependent on ascending projections from the pontine paramedian reticular formation, omnipause neurons in the nucleus raphe interpositus, and inputs from the contralateral vestibular nuclei. The riMLF projects to the ipsilateral oculomotor (CN III) and trochlear (CN IV) nuclei. Reciprocal connections occur between the right and left riMLF through the posterior and anterior commissures of the midbrain. The fibres of the elevator nuclei which innervate the superior rectus and the inferior oblique muscles pass through the posterior commissural pathways, and the projections to the depressor nuclei innervating the inferior rectus and superior oblique muscles pass through the anterior commissural pathways. The velocity commands of vertical saccadic movements are integrated by the interstitial nucleus of Cajal (Fukushima et al. 1990) (Fig. 13.25).
Cortical modulation of saccadic eye movements
Cortical modulation of saccadic eye movements is also a necessary component for normal vision. The frontal lobe contains three areas that contribute to saccadic control; these are the frontal eye fields (FEF), the supplementary eye fields, and the dorsal lateral prefrontal cortex. The FEF are composed of a group of neurons in the posterior areas of Brodmann area 8 that discharge prior to saccadic movement and are thought to play a part in the initiation of saccades to previously seen or remembered targets (Bruce & Goldberg 1985). Excitation of these neurons results in contralateral saccades. Neurons in the supplementary FEF which are located in the dorsomedial frontal lobes are involved in programming saccades as part of complex learned behaviours (Mann et al. 1988). The dorsal lateral prefrontal cortex is involved with programming saccades to remembered target locations (Boch & Goldberg 1989).
Control of smooth pursuit
Smooth pursuit movements allow viewing of moving objects. They are much slower than saccades and can reach a maximum velocity of only 100° per second. They are not under voluntary control. Smooth pursuit activities seem to be modulated in the middle and superior medial temporal visual areas. These areas of cortex project to the dorsal lateral median pontine nuclei (Suzuki et al. 1990). The dorsal lateral median pontine nuclei project to the flocculus, uvula, and dorsal of the cerebellum where the smoothness of the motion is calculated and adjusted.
Cerebellar influences on eye movements
The cerebellum is involved in two basic operations involving eye control. The first involves its role in real-time positional eye control with respect to visual acquisition, and the second involves long-term adaptive control mechanisms regulating the oculomotor system (Leigh & Zee 1991).
The cerebellum functions to ensure that the movements of the eyes are appropriate for the stimulation that they are receiving. The flocculus of the vestibulocerebellum contains Purkinje cells that discharge in relation to the velocity of eye movements during smooth pursuit tracking, with the head either stationary or moving. For example, you can keep your head still and fixate your gaze on a moving object, in which case your eyes should smoothly follow the object across your visual field. Or you could keep your eyes fixed on a stationary object and rotate your head, in which case your eyes should still smoothly track in the opposite direction and at the same speed as the rotation of your head to maintain the target in focus (Zee et al. 1981). Other neurons discharge during saccadic eye movement in relation to the position of the eye in the orbit. Individual control of eye movement is accomplished for the most part by the contralateral cerebellum although intimate bilateral integration is also important. For example, the smoothness of pursuit activity and the return to centre function of saccadic movement of the right eye are under left cerebellar modulatory control.
Disorders of smooth pursuit
1. Lesions or dysfunction of the peripheral vestibular apparatus usually produce horizontal nystagmus with the slow phase towards the side of the lesion.
2. Up-beating nystagmus usually represents a lesion or dysfunction in pontomedullary or pontomesencephalic junction or areas surrounding the forth ventricle.
3. Down-beating nystagmus usually reflects disease or dysfunction of areas around the craniocervical junction such as Arnold–Chiari malformations or degenerative lesions of the cerebellum.
Trigeminal nerve (CN V)
The trigeminal nerve has three projection divisions that supply different areas of the head and face. The ophthalmic division supplies sensation from the midpoint of the eyes to the apex of the frontal skull at the level of the ears. The maxillary division supplies the nasal mucosa and the skin from the upper lip to the inferior halve of the eye. The mandibular division supplies the internal mouth, tongue, teeth, skin of lower jaw, and part of the external ear and auditory meatus and meninges. The sensory neurons are located in the semilunar or Gasserian ganglion. Motor neurons in the motor nucleus of the trigeminal nerve, which is located in the mid-pons area, supply motor innervation to the muscles of mastication, which include the masseter, temporal, internal, and external pterygoid muscles. Neurons in the otic ganglion supply motor fibres to the tensor tympani and tensor veli palatine. Fibres of the trigeminal nerve also supply motor innervation to the mylohyoid muscle and the anterior belly of the digastric muscle via the mylohyoid nerve (Moore 1980; Wilson-Pauwels et al. 1988). Fibres of the ophthalmic division relay sensation of the cornea and are involved in the afferent loop of the corneal reflex (see Chapter 4) (Fig. 13.27).
The corneal reflex
1. Saccadic dysmetria occurs when the saccade over- or undershoots the target. This type of lesion is characteristic of lesions of the dorsal vermis or fastigial nuclei of the cerebellum. In Wallenburg’s syndrome a specific dysmetric pattern that involves overshooting saccades to the side of the lesion and undershooting saccades to the contralateral side occurs. When pure vertical saccades are attempted there is an inappropriate horizontal component to the saccade that results in the eye drifting towards the side of the lesion (Ranalli & Sharpe 1986).
2. Decreases in velocity of the saccade are usually related to dysfunction of the burst neurons. Slow horizontal saccades involve the horizontal burst neurons in the pons, and slow vertical saccades involve the vertical burst neurons in the midbrain. Diseases such as olivopontocerebellar atrophy and progressive supranuclear palsy can affect these neurons, respectively.
3. A mismatch between the pulse phase and the step phase of saccadic movement can result in postsaccadic drift of the eyes or glissades. This condition occurs with dysfunction of the vestibulocerebellar inputs.
4. A combination of slow, hypometric saccades and glissades can occur with disorders such as ocular nerve palsies, myasthenia gravis, and ocular myopathies.
5. Saccades that exhibit an increased latency of action may be caused by dysfunction of the frontal or parietal lobes. They have been reported in Huntington’s disease, supranuclear palsies, and Alzheimer’s disease.
6. Saccadic oscillations are referred to as ocular flutter when they are limited to the horizontal plane and opsoclonus when they are multidirectional in nature such as vertical and/or torsional. These lesions have been reported with various types of encephalitis and neuroblastomas and in association with certain toxins.
Facial nerve (CN VII)
The parasympathetic efferent projections of the facial nerve arise from the nervus intermedius and involve motor axons to the submandibular gland and the lacrimal gland. The motor fibres project in two different pathways and to two different ganglia. The motor projections to the submandibular gland arise from neurons in the superior salivatory nucleus in the medulla. The axons of these neurons emerge from the brainstem in the nervus intermedius and join the facial nerve until the stylomastoid foramen where they separate as the chorda tympani, which traverse the tympanic cavity until they reach and join with the lingual nerve. They travel with the lingual nerve until they reach and synapse on the postganglionic neurons of the submandibular ganglion. The axons from these neurons project to the submandibular glands via the lingual nerve supplying the secretomotor fibres to the gland. Activation of the postganglionic neurons results in dilatation of the arterioles of the gland and increased production of saliva (Moore 1980; Wilson-Pauwels et al. 1988) (Fig. 13.28).
Vestibulocochlear nerve (CN VIII)
The vestibulocochlear nerve, as the name implies, is composed of two separate nerve supplies, the vestibular portion and the cochlear portion.
The axons from the hair cells of the utricle synapse in the superior vestibular ganglion. The axons of the neurons in the superior vestibular ganglion then form the superior vestibular nerve. These axons contribute, along with the axons of the inferior vestibular nerve from the saccule and the cochlear nerve, to form the ipsilateral vestibulocochlear nerve (see Chapter 14).
The cochlear nerves arise from the axons of the bipolar cells of the spiral ganglion which terminate in the ventral or dorsal cochlear nucleus (Moore 1980; Wilson-Pauwels et al. 1988).
The ventral cochlear nucleus (VCN)
1. Anteroventral cochlear nucleus—This division has the most prominent output and projects via the ventral acoustical stria and the trapezoid body to the superior olivary complex (medial and lateral divisions). The anteroventral cochlear nucleus receives input from the ascending branch of the cochlear nerve.
2. Posteroventral cochlear nucleus—This division contributes axons to the trapezoid body and to the lateral superior olive via the intermediate acoustical stria.
The dorsal cochlear nucleus (DCN)
1. Type II units (thought to arise from vertical cells in the deep DCN region); and
2. Wideband inhibitors with evidence pointing to cells in the posteroventral cochlear nucleus (PVCN) region.
Type II units have very low spontaneous rates of firing and give weak responses to broadband noise. They primarily respond to best frequency tones and provide inhibition to type IV units of the DCN. They are also thought to form inhibitory connections with bushy and multipolar cells of the VCN.
Lateral superior olive (LSO)
A given neuron in the LSO responds best when the intensity of a stimulus reaching one ear exceeds that on the opposite ear by a particular amount. The lateral olive is more efficient at processing high-frequency sounds because the head absorbs short wavelengths better than long wavelengths. This allows for clearer interaural intensity differences. Low-frequency sounds are processed more efficiently by the medial olive.
Plasticity in the auditory cortex
Tinnitus has been referred to as a phantom auditory sensation that may occur because of reorganisation of the auditory cortex following some degree of deafferentation from the cochlea of the inner ear. Cochlear lesions resulting in loss of a specific range of frequencies can lead to reorganisation of the auditory cortex due to replacement of the corresponding cortical areas with neighbouring areas of sound representation (McIntosh & Gonzalez-Lima 1998).
Glossopharyngeal nerve (CN IX)
These nerves exit the brainstem as several rootlets along the upper ventrolateral medulla, just below the exiting rootlets of the vestibulocochlear nerves, inferior to the pontomedullary junction. The nerves then course through the subarachnoid space to exit the skull via the jugular foramen. The nerves subserve a variety of functions including (Moore 1980; Wilson-Pauwels et al. 1988) (Fig. 13.29):
1. The sensation of taste from the posterior third of the tongue via the rostral nucleus solitarius or the gustatory nucleus;
2. Information concerning blood pressure and blood gases via baroreceptors and chemoreceptors in the carotid body via the caudal nucleus solitarius or the cardiorespiratory nucleus;
3. The sensations relating to touch, pain, and temperature from the middle ear, external auditory meatus, pharynx, and posterior third of the tongue via the inferior and superior glossopharyngeal ganglia;
4. Parasympathetic supply to the parotid glands via the inferior salivatory nucleus, the lesser petrosal nerve, and the otic ganglia; and
5. Motor supply to the stylopharyngeus muscle via the nucleus ambiguus of the medulla.
Vagus nerve (CN X)
The branchial motor projections, which include motor supply to the muscles of the pharynx and larynx, arise from the nucleus ambiguus of the medulla. The branchial motor component includes the pharyngeal muscles responsible for the gag reflex and swallowing, and the laryngeal muscles that control the vocal cords. The laryngeal muscles are innervated by two branches of the vagus nerve, the recurrent laryngeal nerve, and the superior laryngeal nerve. The recurrent laryngeal nerve is clinically important because it loops down around the aorta before ascending to the larynx and may be affected by cardiac or aortic involvement leading to a change or harshness in voice tone (Figs 13.31 and 13.32).
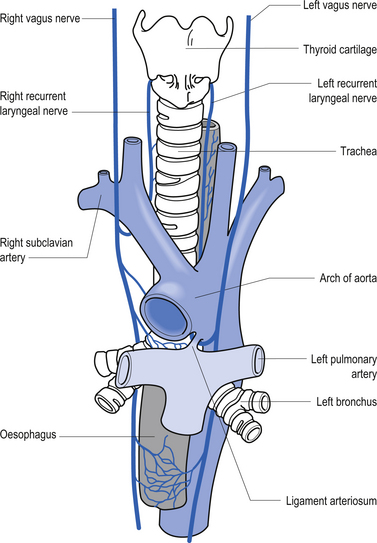
Figure 13.32 The course and relationship of the vagus nerves and their recurrent laryngeal branches.
The parasympathetic motor projections of the vagus nerve arise from the neurons of the dorsal motor nucleus. The cardiac branches are inhibitory, and in the heart they act to slow the rate of the heartbeat. The pulmonary branches are excitatory and in the lungs they act as a bronchoconstrictor as they cause the contraction of the nonstriate muscles of the bronchi. The gastric branch is excitatory to the glands and muscles of the stomach but inhibitory to the pyloric sphincter. The intestinal branches, which arise from the postsynaptic neurons of the mesenteric plexus or Auerbach’s plexus and the plexus of the submucosa or Meissner’s plexus, are excitatory to the glands and muscles of the intestine, caecum, vermiform appendix, ascending colon, right colic flexure, and most of the transverse colon but inhibitory to the ileocaecal sphincter (Fig. 13.31). The ganglia for most of the vagal distribution occur in close association to the effector organs and are referred to as terminal ganglia.
The general somatic sensory projections of the vagus detect pain, temperature, and touch sensations in the pharynx, infratentorial meninges, and a small region of the external auditory meatus. The neuron cell bodies are located in the inferior or nodose ganglion and the superior or jugular ganglion. These ganglia are comparable to the dorsal root ganglion of the spinal cord (Fig. 13.31).
The visceral sensory projections of the vagus carry taste sensations from the epiglottis and pharynx to the rostral nucleus solitarius (gustatory centre), and chemo- and baroreceptor input from the aortic arch receptors to the caudal nucleus solitarius (cardiorespiratory centre). The neuron cell bodies are located mainly in the inferior or nodose ganglia (Moore 1980; Wilson-Pauwels et al. 1988) (Fig. 13.31).
Accessory nerve (CN XI)
These nerves are sometimes referred to as the spinal accessory nerves because some the projection fibres arise in the cervical spine and ascend to exit the skull via the jugular foramen in association with the cranial branches, which are accessory to the vagus nerves. The accessory nerves are formed by the union of the cranial and spinal projection axons but they are associated for only a very brief portion of their course. The cranial portion of the nerve arises in the caudal nucleus ambiguus and exits the lateral surface of the medulla to course via the jugular foramen, where it joins the vagus nerve on exiting. The cranial portion of the nerve supplies motor innervation to the wall of the larynx and pharynx. The spinal portion of the nerves arises in a column of neurons located in the anterior horn of the first five or six cervical segments referred to as the spinal accessory nuclei. The spinal roots exit the spinal cord laterally between the dorsal and ventral roots of the spinal cord to form a trunk that ascends in the subarachnoid space of the spinal canal, through the foramen magnum to exit the skull through the jugular foramen. The spinal portion of the nerve supplies two superficial muscles of the neck, the sternocleidomastoid and trapezius muscles (Fig. 13.33) (Moore 1980; Wilson-Pauwels et al. 1988). The sternocleidomastoid muscles turn the head in the opposite direction. So the right sternocleidomastoid muscle turns the head to the left. This is important clinically because a lower motor neuron lesion of the CN XI nerve will result in an ipsilateral shoulder shrug weakness and a weakness in turning the head to the side opposite the shoulder shrug weakness. The upper motor neuron projections are thought to project to the ipsilateral spinal accessory nuclei, which would also result in weakness of ipsilateral shoulder shrug and turning the head away from the side of the lesion (Blumenfeld 2002).
The hypoglossal nerve (CN XII)
The hypoglossal nerves arise from the hypoglossal nuclei posterior part of the floor of the fourth ventricle in the medulla. These nerves exit the medulla between the olive and the pyramid and course through the subarachnoid space to exit the skull via the hypoglossal canal of the occipital bone. The many rootlets that have exited the medulla unite as they emerge from the hypoglossal canal and then course posterior to the vagus nerve, where they pick up fibres from the cervical roots of C1 and C2 spinal levels. The hypoglossal nerves supply the motor innervation to the tongue, and send collateral branches to the sympathetic trunk and the lingual nerves (Moore 1980; Wilson-Pauwels et al. 1988) (Fig. 13.34).
Clinical testing of cranial nerves
Clinical testing of cranial nerves is covered in detail in Chapter 4. A brief overview will be included here for completeness.
When performing the neurological examination it is most important to remember the functional relationships between the cranial nerves and the various levels of the neuraxis, including the reticular formation and lobes of the brain and cerebellum (Table 13.1).
Brainstem respiratory control centres
The affect of increased partial pressure of carbon dioxide (PCO2) on neuron function
Changes in the H+ concentration in the ECF stimulate the chemoreceptors in the vicinity of the medullary respiratory centres. In other words, the brain ECF H+ concentration is a direct reflection of PCO2 (Champe & Harvey 1994).
Regulation of blood pressure
Sympathetic imbalances may also arise because of altered integration in the brainstem reticular formation or the IML cell column of the spinal cord due to peripheral or descending central influences on the reticular neurons. Visceral afferents or ascending spinoreticular projections from somatic Aδ and C fibres promote activation of the rostral ventrolateral medulla, which increases vasomotor tone (Holt et al. 2006). This alters the systemic vascular resistance and modulates the systemic blood pressure.
Case 13.1
References
Brown L.T. Corticorubral projections in the rat. J. Comp. Neurol.. 1974;154:149-168.
Guyton A.C., Hall J.E. Textbook of Medical Physiology, nineth ed. Philadelphia: WB Saunders, 1996.
Leigh R.J., Zee D.S. The Neurology of Eye Movements, second ed. Philadelphia: Davis, 1991.
Moore K.L. Clinically Orientated Anatomy. Baltimore: Williams and Wilkins, 1980.