8 The autonomic nervous system
Organisation of the autonomic nervous system
The autonomic nervous system comprises the major autonomous or non-volitional efferent outflow to all organs and tissues of the body with the exception of skeletal muscle. Anatomically, the autonomic outflow from the spinal cord to the end organ occurs through a chain of two neurons consisting of a pre- and postganglionic component. The preganglionic component neurons live in the grey matter of the spinal cord. The postganglionic component neurons vary in location with some living in the paraspinal or sympathetic ganglia, and others in ganglia distant from the cord, known as stellate ganglia. Although historically only the efferent connections were considered, all of the projections of the autonomic nervous system are reciprocal in nature and involve both afferent and efferent components. The autonomic system can be divided into three functionally and histologically distinct components: the parasympathetic, sympathetic, and enteric systems. All three systems are modulated by projections from the hypothalamus. Hypothalamic projections that originate mainly from the paraventricular and dorsal medial nuclei influence the parasympathetic and sympathetic divisions as well as the enteric division of the autonomic nervous system. These descending fibres initially travel in the medial forebrain bundle and then divide to travel in both the periaqueductal grey areas and the dorsal lateral areas of the brainstem and spinal cord. They finally terminate on the neurons of the parasympathetic preganglionic nuclei of the brainstem, the neurons in the intermediate grey areas of the sacral spinal cord, and the neurons in the intermediolateral cell column of the thoracolumbar spinal cord. Descending autonomic modulatory pathways also arise from the nucleus solitarius, noradrenergic nuclei of the locus ceruleus, raphe nuclei, and the pontomedullary reticular formation (PMRF).
The parasympathetic system communicates via both efferent and afferent projections within several cranial nerves including the oculomotor (CN III) nerve, the trigeminal (CNV) nerve, the facial (CNVII) nerve, the glossopharyngeal nerve, and the vagus (CN X) and accessory (CN XI) nerves (Fig. 8.1). The vagus nerve and sacral nerve roots compose the major output route of parasympathetic enteric system control (Furness & Costa 1980). Axons of the preganglionic nerves of the parasympathetic system tend to be long, myelinated, type II fibres and the postganglionic axons tend to be somewhat shorter, unmyelinated, C fibres (see Chapter 7). The cell bodies of parasympathetic preganglionic neurons are located in discrete nuclei at various levels of the brainstem as described above and in the intermediolateral cell column of levels S2–4 in the spinal cord or vertebral level L1–2. In contrast to the sympathetic system, the preganglionic parasympathetic neurons are generally longer than the postganglionic neurons as they synapse in ganglia that are further from their origin and closer to the effector than the postganglionic neurons innervate.
The neurotransmitter released both pre- and postsynaptically is acetylcholine. Cholinergic transmission can occur through G-protein coupled mechanisms via muscarinic receptors or through inotropic nicotinic receptors. The activity of ACh is terminated by the enzyme acetylcholinesterase, which is located in the synaptic clefts of cholinergic neurons. To date, seventeen different subtypes of nicotinic receptors and five different subtypes of muscarinic receptors have been identified (Nadler et al. 1999; Picciotto et al. 2000).
Cholinergic, nicotinic receptors are present on the postsynaptic neurons in the autonomic ganglia of both sympathetic and parasympathetic systems. Cholinergic, muscarinic receptors are present on the end organs of postsynaptic parasympathetic neurons (Fig. 8.2).
The neurological output from the parasympathetic system is the integrated end product of a complex interactive network of neurons spread throughout the mesencephalon, pons, and medulla. The outputs of the cranial nerve nuclei including the Edinger–Westphal nucleus, the nucleus tractus solitarius, the dorsal motor nucleus, and nucleus ambiguus are modulated via the mesencephalic reticular formation (MRF) and PMRF. This complex interactive network receives modulatory input from wide areas of the neuraxis including all areas of cortex, limbic system, hypothalamus, cerebellum, thalamus, vestibular nuclei, basal ganglia, and spinal cord (Walberg 1960; Angaut & Brodal 1967; Brodal 1969; Brown 1974; Webster 1978). The relationship of the parasympathetic outflow to the immune system has received very little study to date and as a consequence very little is known about the influence of the parasympathetic or the enteric system on immune function.
Supraspinal modulation of autonomic output
Monosynaptic connections between two structures suggest an important functional relationship between the two structures in question. Polysynaptic connections may be important as well but are not as well understood as monosynaptic connections. Monosynaptic connections have been demonstrated to exist between a variety of nuclei in the medulla, pons, diencephalon, and the preganglionic neurons of the IML (Smith & DeVito 1984; Natelson 1985). Nuclei with monosynaptic connections with the neurons of the IML include:
• Areas of the ventral lateral reticular formation including neuron pools in the ventral pons and ventral lateral medulla;
• Neuron pools in the locus ceruleus of the dorsal rostral pons;
• Serotonergic neurons of the raphe nucleus;
• Epinephrine-producing neurons of the caudal ventrolateral medulla;
• Neuron pools of the parabrachial complex;
• Neuron pools of the central grey area and the zona incerta; and
• Neuron pools in the paraventricular and dorsal medial nuclei of the hypothalamus.
The projections from the cerebral cortex and their role in modulation of autonomic function are not well understood. However, existence of direct projections from the cortex to subcortical structures regulating autonomic function has been established (Cechetto & Saper 1990). Neurophysiological studies demonstrating autonomic changes with stimulation and inhibition of the areas of cortex also suggest a regulatory role. The following outlines the established areas of cortex and their projection areas:
1. Medial prefrontal cortex has direct projections to the amygdala, hypothalamus, brainstem, and spinal cord areas involved in autonomic control.
2. The cingulate gyrus has direct projections to the amygdala, hypothalamus, brainstem, and spinal cord areas involved in autonomic control.
3. The insular and temporal pole areas of cortex also demonstrate direct projections to the amygdala, hypothalamus, brainstem, and spinal cord areas involved in autonomic control.
4. Primary sensory and motor cortex are thought not to control autonomic activity directly but to coordinate autonomic outflow with higher mental functions, emotional overlay, and holistic homeostatic necessities of the system.
Most areas modulating the autonomic systems are bilateral structures
It is worth noting at this point that with the exception of a few midline structures in the brainstem, the locus ceruleus, and the raphe nuclei, all other structures that modulate the autonomic output are bilateral structures. This presents the possibility that asymmetric activation or inhibition lateralised to one side or the other may translate to the activity of the end organs and produce asymmetries of function from one side of the body to the other (Lane & Jennings 1995). Accurately assessing the asymmetric functional output of the autonomic nervous system is a valuable clinical tool in evaluating asymmetrical activity levels of cortical or supraspinal structures that project to the output neurons of the autonomic system.
The autonomic ganglion
Incoming and outgoing nerve bundles are attached to the ganglion (Fig. 8.3). The incoming bundles contain afferent fibres from the periphery returning to the spinal cord, preganglionic axons that synapse on the postganglionic neurons in the ganglion, preganglionic axons that pass through the ganglion giving off collateral axons to the interneurons as they do so, and descending axons from cholinergic neurons in the spinal cord that modulate the activity of the interneurons in the ganglion. The interneurons in the ganglion are referred to as small intensely fluorescent cells and they are thought to be dopaminergic in nature. The outgoing bundle contains postganglionic axons, and afferent fibres from the periphery entering the ganglion (Snell 2001). The presence of such a complex structure in the ganglion has led to the suspicion that the ganglion is not just a relay point but an integration station along the pathway of the autonomic projections.
Parasympathetic efferent projections
The oculomotor parasympathetic fibres commence in the midbrain. These fibres are the axon projections of neurons located in the Edinger–Westphal nucleus (EWN) or accessory oculomotor nuclei. The parasympathetic projections travel with the ipsilateral oculomotor nerve and exit with the nerve branch to the inferior oblique muscle and enter the ciliary ganglion where they synapse with the postganglionic neurons. The axons of the postganglionic neurons then exit the ganglion via the short ciliary nerves and supply the ciliary muscle and the sphincter pupillae. Activation of the postganglionic neurons causes contraction of both the ciliary muscle, resulting in relaxation of the lens, and the sphincter pupillae muscle, resulting in constriction of the pupil. These actions can be stimulated separately or simultaneously as in the accommodation reflex (Fig. 8.4).
The parasympathetic efferent projections of the facial nerve involve motor axons to the submandibular gland and the lacrimal gland. The motor fibres project in two different pathways and to two different ganglia. The motor projections to the submandibular gland arise from neurons in the superior salivatory nucleus in the medulla. The axons of these neurons emerge from the brainstem in the nervous intermedius and join the facial nerve until the stylomastoid foramen where they separate as the chorda tympani, which traverse the tympanic cavity until they reach and join with the lingual nerve. They travel with the lingual nerve until they reach and synapse on the postganglionic neurons of the submandibular ganglion. The axons from these neurons project to the submandibular glands via the lingual nerve supplying the secretomotor fibres to the gland. Activation of the postganglionic neurons results in dilatation of the arterioles of the gland and increased production of saliva (Fig. 8.5).
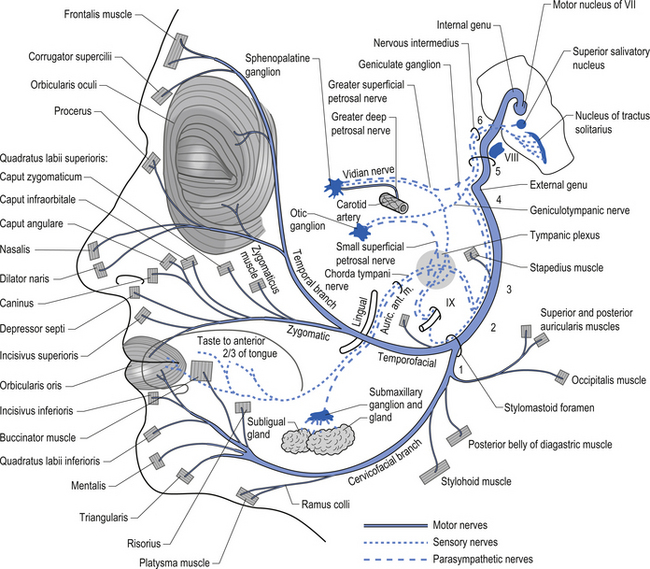
Figure 8.5 This figure outlines the motor and parasympathetic projections of the facial nerve (CN VII).
The motor projections of the vagus nerve arise from the neurons of the dorsal motor nucleus and the nucleus ambiguus of the medulla. The cardiac branches are inhibitory, and in the heart they act to slow the rate of the heartbeat. The pulmonary branch is excitatory and in the lungs they act as a bronchoconstrictor as they cause the contraction of the non-striate muscles of the bronchi. The gastric branch is excitatory to the glands and muscles of the stomach but inhibitory to the pyloric sphincter. The intestinal branches, which arise from the postsynaptic neurons of the mesenteric plexus or Auerbach’s plexus and the plexus of the submucosa or Meissner’s plexus, are excitatory to the glands and muscles of the intestine, caecum, vermiform appendix, ascending colon, right colic flexure, and most of the transverse colon but inhibitory to the ileocaecal sphincter (Fig. 8.6).
The sympathetic system enjoys a wide-ranging distribution to virtually every tissue of the body (Fig. 8.7). The presynaptic neurons live in a region of the grey matter of the spinal cord called the intermediomedial and intermediolateral cell columns located in lamina VII. Axons of these neurons exit the spinal cord via the ventral rami where they further divide to form the white rami communicantes. The fibres then follow one of several pathways (Fig. 8.8):
1. They synapse in the paravertebral or prevertebral ganglia segmentally.
2. They synapse in segmental regions of the paravertebral or prevertebral ganglia other than those at which they exited.
3. They do not synapse in the prevertebral or paravertebral ganglia and continue as presynaptic myelinated fibres into the periphery (Williams & Warwick 1984).
The output of the preganglionic neurons of the sympathetic system is the summation of a complex interactive process involving segmental afferent input from dorsal root ganglion and suprasegmental input from the hypothalamus, limbic system, and all areas of cortex via the MRF and PMRF (Donovan 1970; Webster 1978; Williams & Warwick 1984). Most postganglionic fibres of the sympathetic nervous system release norepinephrine as their neurotransmitter. The adrenergic receptors bind the catecholamines norepinephrine (noradrenalin) and epinephrine (adrenalin).
These receptors can be divided into two distinct classes, the alpha adrenergic and beta adrenergic receptors (see Fig. 8.2). The chromaffin cells of the adrenal medulla which are embryological homologues of the paravertebral ganglion cells are also innervated by preganglionic sympathetic fibres which fail to synapse in the paravertebral ganglia as described above. When stimulated, these cells release a neurotransmitter/neurohormone that is a mixture of epinephrine and norepinephrine with a 4:1 predominance of epinephrine (Elenkov et al. 2000).
Both epinephrine and norepinephrine are manufactured via the tyrosine–dihydroxyphenylalanine (DOPA) –dopamine pathway and are called catecholamines. When the body is in a neutral environment, catecholamines contribute to the maintenance of homeostasis by regulating a variety of functions such as cellular fuel metabolism, heart rate, blood vessel tone, blood pressure and flow dynamics, thermogenesis, and as explained below, certain aspects of immune function. When a disturbance in the homeostatic state is detected, both the sympathetic nervous system and the hypothalamus–pituitary–adrenal axial system become activated in the attempt to restore homeostasis via the resulting increase in both systemic (adrenal) and peripheral (postganglionic activation) levels of catecholamines and glucocorticoids. In the 1930s, Hans Selye described this series of events or reactions as the general adaptation syndrome or generalised stress response (Selye 1936). Centrally, two principal mechanisms are involved in this general stress response; these are the production and release of corticotrophin releasing hormone produced in the paraventricular nucleus of the hypothalamus and increased norepinephrine release from the locus ceruleus norepinephrine releasing system in the brain stem. Functionally, these two systems cause mutual activation of each other through reciprocal innervation pathways (Chrousos & Gold 1992). Activation of the locus ceruleus results in an increased release of catecholamines, of which the majority is norepinephrine, to wide areas of cerebral cortex, subthalamic, and hypothalamic areas. The activation of these areas results in an increased release of catecholamines from the postganglionic sympathetic fibres as well as from the adrenal medulla.
Functional effects of sympathetic stimulation
Sympathetic projections that innervate structures in the cranial region arise from preganglionic neurons in the spinal IML at the level of T1. Axons from these neurons exit the spinal column and pass uninterrupted through the cervicothoracic ganglion to reach the superior cervical ganglion where they terminate on the postganglionic neurons in the ganglion. Axons from these neurons then project via the internal carotid nerve, which courses with the carotid artery through the carotid canal into the cranium, where it enlarges to form the carotid plexus. Fibres emerging from the carotid plexus accompany all of the cranial nerves to innervate the blood vessels in the distribution of the cranial nerves. Visceromotor and vasomotor fibres course with the oculomotor nerve to the ciliary ganglion where they pass through uninterrupted to form the long ciliary nerves which course to the eyeball. The vasomotor fibres control the extent of vasoconstriction of the arterioles supplying the eyeball. The visceromotor fibres terminate on the dilatator pupillae muscle of the iris where activation results in pupillary dilation (Fig. 8.9). Some fibres course to the levator palpebrae superioris muscles of the upper eyelid, also known as Muller’s smooth muscle. Activation of these fibres results in the contraction of these muscles which retract the eyelid. The sympathetic supply to this muscle only composes a partial segment of the innervation, which is also contributed to by motor fibres in the oculomotor nerve. Other fibres emerge from the ciliary ganglia to innervate the ciliary muscles. Activation of these fibres results in contraction of the ciliary muscles, which causes the lens to relax for better focus of near objects.
Vestibuloautonomic reflexes
These reflexes are very complex and it appears that certain neurons within the vestibular system may have a greater effect on the excitatory phase and others have a greater effect on the inhibitory phase. Whatever the case, it should be clear that increased output from the vestibular nucleus or vestibular receptors can increase both sympathetic and parasympathetic activity at the same time. For example, this may result in a rise in blood pressure and sweating due to activation of the RVLM and hypothalamus and increased tearing and bowel activity due to activation of the SSN and the DMN of the vagus. A loss of vestibular activity may lead to orthostatic hypotension due to poor maintenance of vasomotor tone when changing posture. Significant overactivity of the vestibular system could also create the symptoms of light-headedness due to excessive vasomotor tone throughout the carotid tree.
Horner’s syndrome
Disruption of the sympathetic chain at any point from the hypothalamic or supraspinal projections to the oculomotor nerve can result in a spectrum of symptoms referred to as Horner’s syndrome. The classic findings in this syndrome include ptosis, miosis, and anhidrosis but a number of other abnormalities may also be present. Ptosis or drooping of the upper eyelid is caused by the interruption of the sympathetic nerve supply to the muscles of the upper eyelid. Miosis or decreased pupil size is a result of the decreased action of the dilator muscles of the iris due to decreased sympathetic input. This results in the constrictor muscles acting in a relatively unopposed fashion, resulting in pupil constriction. A Horner’s pupil will still constrict when light is shined on the pupil although careful observation is sometimes required to detect the reduced amount of constriction that occurs. Another test that can be used in these cases utilises the ciliospinal reflex, which results in pupil dilation in response to pain. A pinch applied to the neck region will result in bilateral pupil dilation under normal conditions. However, in the case of unilateral disruption of the sympathetic innervation as in Horner’s syndrome, the pupil on the effected side will show decreased or absent dilation response. Occasionally, the appearance of enophthalmos or retraction of the eyeball into the eye socket occurs because of the relaxation of the eyelid muscles. Anhidrosis or reduced sweating capability sometimes also occurs on the ipsilateral face and neck in Horner’s syndrome. The affected skin will usually appear shiny and will feel smooth to the touch compared with the non-involved areas. Anhidrosis is usually not associated with postganglionic lesions or lesions above the superior cervical ganglion because sympathetic projections to the face and neck emerge from the sympathetic chain prior to the superior cervical ganglion. However, if the disruption occurs in the hypothalamic projections to the IML, anhidrosis may actually be present on the entire upper quarter on the ipsilateral side. The causes of Horner’s syndrome can be multiple and varied and include (Fig. 8.10):
• Infarcts or haemorrhage of the lateral brainstem;
• Apical lung tumour, also referred to as Pancoast tumour or syndrome;
• Trauma or tumour of the neck region;
• Injuries or pathology of the carotid plexus including carotid dissection;
• Interruption of the cavernous sinus including thrombus, aneurysm, or neoplasm;
The distribution of the sympathetic system is widespread
• Vasomotor fibres to the brain and eyeball;
• Motor fibres to the pupil and smooth muscles of the upper eyelid;
• Motor fibres to the ciliary muscles of the lens;
• Vasomotor fibres to the meninges of the posterior cranial fossa;
• Vasomotor fibres to the carotid bodies;
• Vasomotor and visceromotor fibres to the sweat glands of the face; and
• Both vasomotor and visceromotor fibres to the thyroid gland;
• Both vasomotor and visceromotor fibres to the parathyroid glands;
• Motor and vasomotor fibres to the trachea;
• Motor and vasomotor fibres to the oesophagus; and
• Vasomotor fibres that accompany the C4 and C5 cervical spinal nerves.
• Vasomotor fibres to the vertebral arteries;
Sympathetic distribution in the thoracic area is consistent with the projections of the paraspinal ganglia at each vertebral segmental level. However, the formation of the splanchnic nerves deserves mention. The splanchnic nerves are formed by preganglionic myelinated fibres that pass through the paraspinal ganglia without synapsing, although some evidence suggests that collateral branching of these fibres which do synapse in the ganglia may occur (see Fig. 8.3).
The autonomic innervation of several clinically important areas will be considered in detail.
Innervation of the heart
Preganglionic parasympathetic neurons that modulate the heart rate reside in the medulla and synapse with postganglionic neurons adjacent to the heart. Parasympathetic fibres project from the nucleus tractus solitarius, dorsal vagal nucleus, and the nucleus ambiguus and course to the periphery in the glossopharyngeal (CN IX) and vagus (CN X) cranial nerves. Direct connections exist between the sensorimotor cortex and the NTS, DMV, and RVLM. These direct cortical projections to the NTS/DMV provide the anatomical basis for cortical influences on both the baroreceptor reflex and cardiac parasympathetic control (Zamrini et al. 1990). These connections also display an ipsilateral predominance.
The neurons of the NTS, DMN, and nucleus ambiguus also send projection fibres to the preganglionic sympathetic neurons in the IML and to other brainstem nuclei that modulate sympathetic outflow (Lane & Jennings 1995). The right and left vagal projections demonstrate an asymmetric distribution with the right vagal projections innervating some aspects of the anterior right and left ventricles and the left vagal projections innervating the posterior lateral aspects of the ventricles. However, the predominant innervation of the vagal projections terminates on the atrial aspects of the heart and include the sinus (SA) node, which usually determines the rate of the heartbeat. The influence of the vagal projections on the ventricles appears to be limited to counteracting the sympathetic innervation (Rardon & Bailey 1983).
Sympathetic innervation of the heart can be separated into left and right sympathetic limbs, based on physiological studies. The right postganglionic sympathetic projections arising from the paravertebral sympathetic ganglia including the stellate ganglia course to the heart and innervate the atria and the anterior surfaces of the right and left ventricles. The left sympathetic projections have a more posterior lateral distribution and innervate the atrioventricular (AV) node and the left ventricle (Levy et al. 1966; Randall & Ardell 1990). Stimulation of the sympathetic projections results in different physiological effects on the heart. Stimulation of the right stellate ganglia produces mainly chronotropic effects such as increases in heart rate, and stimulation of the left stellate ganglia mainly results in inotropic effects such as altered contractility, changes in rhythm, and increase in systemic blood pressure (Levy et al. 1966; Rogers et al. 1978) (Fig. 8.11). Increased stimulation to either or both ganglia results in a decreased fibrillation threshold (Schwartz 1984; Swartz et al. 1994). With respect to cortical control of cardiovascular function, the research suggests that asymmetries in brain function can influence the heart through ipsilateral pathways. It is quite clear that stimulation or inhibition at various levels on the right side of the neuraxis results in greater changes in heart rate, while increased sympathetic tone on the left side of the neuraxis results in a lowered ventricular fibrillation threshold. This occurs because parasympathetic mechanisms are dominant in the atria, while sympathetic mechanisms are dominant in the ventricles (Lane et al. 1992).
Innervation of the urinary bladder
The innervation of the bladder is complex. Afferent sympathetic fibres emerge from the muscle tissue of the bladder, the detrusor muscle, and course through the hypogastric nerve to the upper lumbar sympathetic ganglia. They then course with the posterior nerve roots to the IML neurons at the levels T9–L2 in the spinal cord. These fibres probably transmit proprioceptive and nociceptive information from the bladder. Efferent sympathetic fibres project from the IML neurons at the levels T11–12 and course with the white rami to the hypogastric plexus where they synapse and join the hypogastric nerve to reach the detrusor muscle and internal sphincter of the bladder. Excitation of these fibres results in contraction of the internal sphincter muscle and inhibition of the detrusor muscle. Parasympathetic innervation involves both afferent and efferent projections. The afferent projections arise from the bodies of the detrusor and internal sphincter muscles and course with the pudendal nerve to the S2–4 posterior nerve roots, terminating in the anterolateral grey areas of the spinal cord at these levels. These fibres probably carry proprioceptive, nociceptive, touch, temperature, and muscle stretch information from the bladder tissues. The efferent parasympathetic fibres pass from the S2–4 segments of the spinal cord to the hypogastric plexus where they synapse and project to the detrusor and internal sphincter muscles. Excitation of these fibres results in excitation of the detrusor and inhibition of the internal sphincter muscles.
Cortical control over micturition also exists. Areas in the paracentral lobule of the cortex evoke excitation of bladder contractions; this may play a role in the voluntary control over micturition (Chusid 1982) (Fig. 8.12).
Erection of the penis and clitoris
Ejaculation is accomplished through the action of the sympathetic nervous components that arise in the grey matter of the spinal cord at the spinal cord levels L1–2. The preganglionic fibres synapse in the lumbar ganglia. The axons of the postganglionic neurons then course to the vas deferens, the seminal vesicles, and the prostate gland through the hypogastric plexuses. Excitation of the postganglionic projections results in waves of contraction of the smooth muscles of these structures and the ejaculation of sperm (Snell 2001).
Innervation of the uterus
The innervation of the uterus is mainly through the preganglionic neurons that arise from the T12–L1 levels of the spinal cord. The preganglionic fibres course through the paraspinal ganglion to the inferior hypogastric plexus where they synapse. The axons of the postganglionic neurons then project to the smooth muscle of the uterus where excitation results in vasoconstriction and muscular contraction. Parasympathetic preganglionic fibres arise from neurons in the spinal cord levels S2–4 and synapse in the hypogastric plexuses before projecting to the smooth muscle of the uterus. Excitation of these fibres results in relaxation of the uterine muscles and dilation of the blood vessels. The uterus is also under a high degree of hormonal control as well as neuronal control (Snell 2001).
Referred visceral pain
Since most of the viscera is only innervated by autonomic projections, afferent autonomic nerve pathways must relay nociceptive, temperature, and proprioceptive information back to the central nervous system. Usually, visceral pain is poorly localised and difficult to qualitatively describe. Frequently, information transferred by afferent autonomic fibres is perceived in other areas of the body also innervated by the same segmental levels. This phenomenon is called referred pain. Certain areas of the body have been consistently identified with referred pain from specific organs. These are referred to as referred pain patterns (Fig. 8.13).
Sympathetic control of blood flow
all influence resistance of the vessel.
• A slight change in diameter of the vessel can bring about a large change in flow rate because of the resistance being inversely proportional to the fourth power of the radius.
• The arterial system acts as a pressure reservoir to maintain flow rate when the heart is relaxing, i.e. the large vessels extend and then compress because of the presence of large amounts of elastin in the walls of the vessel. A greater amount of blood enters the same then leaves it during systole – about a third of the amount leaves.
• Capillary flow stays the same during the cardiac cycle.
• Mean arterial pressure is the main driving force of flow rate. It is equal to diastole + τσ diastole.
• Mean arterial pressure = cardiac output × total peripheral resistance.
• Arterioles are the main resistance vessels – capillaries do not offer as much resistance.
• There is a significant drop in mean pressure in the arterioles because of a high degree of arteriolar resistance (from −93 to 37 mmHg). This helps to create the pressure differential and the driving force for blood flow.
Sympathetic innervation causes constriction in most vessels, but heart and skeletal muscle are capable of strongly overriding the vasoconstrictor effect through powerful local metabolic mechanisms. For example, an increase in exercise-induced sympathetic innervation to the heart leads to a greater cardiac output and increased overall sympathetic vasoconstrictor tone. Vessels in the heart and active skeletal muscle will dilate in response to greater metabolic activity and benefit from an overall increase in upstream driving force. Skeletal and heart muscle also have beta2 receptors for epinephrine (adrenalin) that is released from the adrenal medulla in response to increased sympathetic innervations – beta2 receptor activation reinforces the metabolically induced vasodilation in these areas.
In summary, and based on much broader literature searches, the best bet at this point seems to be that hemisphericity will more likely (and in more cases) be associated with decreased forehead skin temperature on the same side. Do not forget also that if hemisphericity is associated with a loss of inhibition of ipsilateral IML, then one would expect to see greater vasoconstriction of forehead vessels anyway. The integrity of the PMRF and vestibular systems is vital in all this. Sometimes, forehead skin temperature will be seen to be decreased on the same side as vestibular escape because of excitatory vestibulosympathetic reflexes – however, the side of vestibular escape will often be the same side as decreased hemisphericity due to decreased contralateral vestibulocerebellar function (Sexton 2006).
Clinical examination of autonomic function
What components of the neurological, physical, or orthopaedic examinations allow one to gain information about autonomic function? Complete examination techniques are covered in Chapter 4. However, due to the importance of the autonomic examination in determining the functional state of the neuraxis the autonomic examination is reviewed again here.
Ophthalmoscopy – vein to artery (V:A) Ratio and vessel integrity
The V:A ratio refers to the difference in size of the veins and arteries that branch from the central retinal artery. A large difference may be due to increased sympathetic output, which causes greater peripheral resistance and constriction of arteries. The condition of blood vessels can also be helpful as an indicator of cerebrovascular integrity (Figs 8.14 and 8.15).
Heart auscultation
Arrhythmias and changes in heart sounds can occur due to altered CIS of the PMRF. A detailed discussion is beyond the scope of this book.
Skin and tympanic temperature and blood flow
This is particularly useful as a pre- and post-adjustment check. Profound changes in skin temperature asymmetry can occur following an adjustment. These changes are side dependent. An adjustment on the side of decreased forehead skin temperature will commonly result in greater symmetry or reversed asymmetry. Conflicting results are likely to be dependent on a number of factors, which are currently being investigated further. Remember that forehead skin temperature depends on fuel requirements of the brain, and vestibular and cortical influences on autonomic function among other things (Sexton 2006).
Dermatographia – the ’flare’ or red response
The red response is often observed in patients who suffer from chronic inflammatory conditions or heightened sensitivity to pain. See complex regional pain syndrome in Chapter 7.
Case 8.2
8.2.1
Preganglionic parasympathetic neurons that modulate the heart rate reside in the medulla and synapse with postganglionic neurons adjacent to the heart. The neurons of the NTS, DMN, and nucleus ambiguus also send projection fibres to the preganglionic sympathetic neurons in the IML and to other brainstem nuclei that modulate sympathetic outflow (Lane & Jennings 1995). The right and left vagal projections demonstrate an asymmetric distribution with the right vagal projections innervating some aspects of the anterior right and left ventricles and the left vagal projections innervating the posterior lateral aspects of the ventricles. However, the predominant innervation of the vagal projections terminates on the atrial aspects of the heart and include the sinus (SA) node, which usually determines the rate of the heartbeat. The influence of the vagal projections on the ventricles appears to be limited to counteracting the sympathetic innervation.
References
Brodal A. Neurological Anatomy. London: Oxford University Press, 1969.
Brown L.T. Corticorubral projections in the rat. J. Comp. Neurol.. 1974;154:149-168.
Donovan B.T. Mammillian Neuroendocrinology. New York: McGraw-Hill, 1970.
Furness J.B., Costa M. Types of nerves in the enteric nervous system. Neuroscience. 1980;5:1-20.
Natelson B.H. Neurocardiology—an interdisciplinary area for the 80s. Arch. Neurol.. 1985;42:178-184.
Williams P.L., Warwick R. Gray’s Anatomy. Edinburgh: Churchill Livingston, 1984.