Chapter 5 T Cell Receptors and MHC Molecules
• The T cell antigen receptor (TCR) is located on the surface of T cells and plays a critical role in the adaptive immune system. Its major function is to recognize antigen and transmit a signal to the interior of the T cell, which generally results in activation of T cell responses.
• TCRs are similar in many ways to immunoglobulin molecules. Both are made up of pairs of subunits (α and β or γ and δ), which are themselves members of the immunoglobulin superfamily, and both recognize a wide variety of antigens via N terminal variable regions. These antigen recognition subunits are associated with the invariant chains of the T cell receptor, the CD3 complex, which perform critical signaling functions.
• The two types of TCR may have distinct functions. In humans and mice, the αβ TCR predominates in most peripheral lymphoid tissues, whereas cells bearing the γδ TCR are enriched at mucosal surfaces.
• Like immunoglobulins, TCRs are encoded by several sets of genes, and a large repertoire of TCR antigen-binding sites is generated by V(D)J recombination during T cell differentiation. Unlike immunoglobulins, TCRs are never secreted and do not undergo class switching or somatic hypermutation.
• Antigen recognition by the αβ TCR requires the antigen to be bound to a specialized antigen-presenting structure known as a major histocompatibility complex (MHC) molecule. Unlike immunoglobulins, TCRs recognize antigen only in the context of a cell–cell interaction.
• Class I and class II MHC molecules bind to peptides derived from different sources. Class I MHC molecules bind to peptides derived from cytosolic (intracellular) proteins, known as endogenous antigens. Class II MHC molecules bind to peptides derived from extracellular proteins that have been brought into the cell by phagocytosis or endocytosis (exogenous antigens).
• Class I and class II MHC present peptide antigens to the TCR in a cell–cell interaction between an antigen-presenting cell (APC) and a T cell.
• In humans the gene loci HLA-A, HLA-B, and HLA-C gene loci encode class I MHC molecules.
• HLA-DP, HLA-DQ, and HLA-DR gene loci encode class II MHC molecules.
• An individual’s MHC haplotype affects susceptibility to disease.
• CD1 is an MHC class I-like molecule that presents lipid antigens.
T cell receptors
As discussed in Chapter 1, the immune system of higher vertebrates can be divided into two components – humoral immunity and cell-mediated immunity.
• targeting them for phagocytosis or complement-mediated lysis;
• neutralizing receptors on the surface of bacteria and viruses; and
TCRs recognize antigen via variable regions generated through V(D)J recombination (see Chapter 3), much like immunoglobulins, but are much more restricted in their antigen recognition capabilities.
TCRs are similar to immunoglobulin molecules
• resemble immunoglobulins in several significant ways (Fig. 5.1);
• are made up of heterodimers (either α and β or γ and δ subunits), which are disulfide-linked;
• are integral membrane proteins with large extracellular domains and short cytoplasmic tails. The extracellular portions are responsible for antigen recognition and contain variable N terminal regions, like antibodies. Both α and β (or γ and δ) subunits contribute to the antigen-binding sites.
The αβ heterodimer is the antigen recognition unit of the αβ TCR
The αβ TCR is the predominant receptor found in the thymus and peripheral lymphoid organs of mice and humans. It is a disulfide-linked heterodimer of α (40–50 kDa) and β (35–47 kDa) subunits and its structural features have been determined by X-ray crystallography (Fig. 5.2).
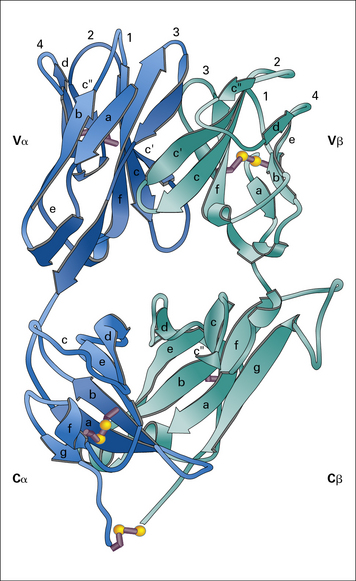
Fig. 5.2 The T cell antigen receptor
(Adapted from Garcia KC, Degano M, Stanfield RL, et al. Science 1996;274:209–219. Copyright AAAS.)
Q. How can receptors that lack intracytoplasmic domains signal to the cell? Give some examples
A. They signal by associating with other membrane molecules that do have intracytoplasmic domains. For example, immunoglobulin associates with Igα and Igβ (see Fig. 3.1), and FcγRI associates with its γ chain dimer (see Fig. 3.17).
The extracellular portions of the α and β chains fold into a structure that resembles the antigen-binding portion (Fab) of an antibody (see Fig. 3.9). Indeed, as in antibodies, the amino acid sequence variability of the TCR resides in the N terminal domains of the α and β (and also the γ and δ) chains.
The regions of greatest variability correspond to immunoglobulin hypervariable regions and are also known as complementarity determining regions (CDRs). They are clustered together to form an antigen-binding site analogous to the corresponding site on antibodies (see Fig. 5.2). Note, however, that:
• the CDR3 loops (which are the most highly variable regions of the TCR, as they are generated by V(D)J recombination) from both the α and β chains lie at the center of the antigen-binding site, and make extensive contacts with antigen.
The disulfide bond that links the α and β chains is in a peptide sequence located between the constant domain of the extracellular portion of the receptor and the transmembrane domain (shown as the C terminal residue in the α and β chain in Fig. 5.2).
The CD3 complex associates with the antigen-binding αβ or γδ heterodimers to form the complete TCR
The CD3 chains are assembled as heterodimers of γε and δε subunits with a homodimer of ζ chains, giving an overall TCR stoichiometry of (αβ)2, γ, δ, ε2, ζ2. Current data suggest that the TCR complex exists as a dimer (Fig. 5.3).
The negatively charged residues in the transmembrane region of the CD3 chains interact with (and neutralize) the positively charged amino acids in the αβ polypeptides, leading to the formation of a stable TCR complex (see Fig. 5.3).
• a small extracellular domain (nine amino acids);
The cytoplasmic portions of ζ and η chains contain ITAMs
The conserved tyrosine residues in the ITAM motifs are targets for phosphorylation by specific protein kinases. When the TCR is bound to its cognate antigen–MHC complex, the ITAM motifs become phosphorylated within minutes in one of the first steps in T cell activation (see Fig. 8.w1).
• are essential for T cell activation, and mutational substitution of the tyrosines in the motif prevents activation;
• play critical roles in B cell activation, and are present in the B cell receptor chains, Igα and Igβ (see Fig. 3.1).
CD3ζ also functions in another signaling pathway, associating with the low-affinity FcγRIIIa receptor (CD16), which is involved in the activation of macrophages and natural killer (NK) cells (see Fig. 3.17).
The γδ TCR structurally resembles the αβ TCR but may function differently
• extracellular V and C domains;
• a transmembrane segment containing positively charged amino acids; and
• in humans and mice, αβ TCRs are present on more than 95% of peripheral blood T cells and on the majority of thymocytes;
• T cells bearing γδ TCRs are relatively rare in spleen, lymph nodes, and peripheral blood but predominate at epithelial surfaces – they are common in skin and in the epithelial linings of the reproductive tract and are especially numerous in the intestine, where they are found as intraepithelial lymphocytes.
Antigen recognition by γδ T cells is unlike that of their αβ counterparts
• γδ T cells can be found in normal numbers in MHC class I and class II-deficient mice;
• their cognate antigens are not necessarily peptides, and do not require classical processing – indeed, some murine γδ T cells have been found to recognize proteins directly, including MHC molecules and viral proteins, in a manner that requires neither antigen processing nor presentation by MHC.
γδ T cells have a variety of biological roles
• are essential for primary immune responses to certain viral and bacterial pathogens in mouse models, but in many cases their contribution to the primary response can be substituted by αβ T cells, and they rarely contribute to memory responses;
• interact with a variety of lymphocytes, and have been implicated in stimulating immunoglobulin class switch recombination by B cells in response to T-dependent antigens;
• provide regulatory signals to αβ T cells and have been implicated in shaping immune responses (e.g. γδ cells appear to be involved in downregulating inflammation and in this role may be responding to epithelial cells stressed by inflammatory processes rather than to specific antigens borne by pathogens).
TCR variable region gene diversity is generated by V(D)J recombination
As with antibody genes, a highly diverse repertoire of TCR variable region genes is generated during T cell differentiation by a process of somatic gene rearrangement termed V(D)J recombination (see Chapter 3). Variable (V), joining (J), and sometimes diversity (D) gene segments are joined together to form a completed variable region gene.
The mechanism of V(D)J recombination is the same in both T cells and B cells
The TCR genes are flanked by recombination signal sequences, just like their immunoglobulin cousins (see Fig. 3.24), and the same recombination machinery (the RAG proteins) operates in both B and T cells. Indeed, experiments have shown that TCR Dβ and Jγ genes can rearrange appropriately even if transfected into B cells.
TCRs are encoded by several sets of genes
The general arrangement of the genes encoding the α, β, γ, and δ chains of the TCR is remarkably similar to that of the immunoglobulin heavy chain genes (see Figs 3.21–3.23), suggesting a common origin from a primordial rearranging antigen receptor locus.
Figure 5.w1 illustrates the murine TCR genes, which are similar to those of humans. All four TCR gene families have been strongly conserved across more than 400 million years of evolution of the jawed vertebrates, which suggests a strong selective pressure for the preservation of both αβ and γδ T cell functions.
The TCRB locus includes two sets of D, J, and C genes
The D segments are used in all three reading frames, further contributing to β chain diversity.
The TCRD locus possesses only five Vδ, two Dδ, and six Jδ genes
Q. How may the great number of δ chains be generated when there is only a limited number of V, D, and J gene segments in the TCRD locus?
A. As described in Chapter 3, the two D region segments can be used in all three possible reading frames, and imprecise joining of the VD and DJ junctions produces further diversity.
MHC molecules
Recognition by the αβ TCR requires antigen to be bound to an MHC molecule
As discussed in Chapter 3, antibodies recognize intact proteins, binding either to linear epitopes derived from contiguous amino acids or discontinuous epitopes produced by amino acids that are not near one another in the primary structure but are brought together in the three dimensional structure of the protein. In contrast, T cell receptors only recognize linear epitopes present in the form of short peptides which are generated by degradation of intact proteins within the cell (a process termed antigen processing). This property of T cell receptors is critical to the way the immune system recognizes intracellular pathogens and tumor antigens. Such antigens present a special challenge to the immune system: intracellular antigens are hidden within the cell, and are not available for recognition by antibodies. How can intracellular antigens be recognized by extracellular receptors? The immune system has solved this problem by evolving an elegant means of displaying internal antigens (including those of intracellular pathogens) on the cell surface, allowing their recognition by T cells:
• proteins from within the cell (either produced by the cell or taken into the cell) are digested into short peptide fragments;
• peptides derived from proteins produced within the cell are displayed on the cell surface through binding to specialized antigen-presenting molecules termed MHC class I molecules, which are present on all nucleated cells of the body;
• in a similar fashion, peptides derived from proteins ingested from the extracellular environment by phagocytosis are presented by MHC class II molecules, which are present only on professional antigen presenting cells;
This antigen processing and presentation pathway, upon which both activation and regulation of the immune response rests, is a complex and fascinating subject (see Chapter 8).
In humans the MHC is known as the HLA
There are no functional or structural similarities between these other gene products.
All mammalian species possess the MHC, though details of the complex vary from one species to the next. In humans the locus is known as the HLA (an abbreviation for human leukocyte antigen); in mice it is known as the H-2 locus (Fig. 5.4).
MHC molecules provide a sophisticated surveillance system for intracellular antigens
From a cell’s perspective, there are two types of antigen that must be dealt with:
• endogenous antigens (antigenic peptides from viruses or other pathogens that inhabit the cell);
• exogenous antigens (antigenic peptides from extracellular pathogens that have been taken up by a professional APC).
MHC class I molecules consist of an MHC-encoded heavy chain bound to β2-microglobulin
The overall structure of the extracellular portion of an MHC class I molecule is depicted in Figure 5.5. It comprises a glycosylated heavy chain (45 kDa) non-covalently associated with β2-microglobulin (12 kDa), which is a polypeptide that is also found free in serum.
The class I heavy chain consists of:
The three extracellular domains each comprise about 90 amino acids:
• the α2 and α3 domains both have intrachain disulfide bonds enclosing loops of 63 and 86 amino acids, respectively;
• the α3 domain is structurally homologous to the immunoglobulin constant region domain (C) and contains a site that interacts with CD8 on cytotoxic T cells.
The hydrophilic cytoplasmic domain, 30–40 residues long, may be phosphorylated in vivo.
β2-Microglobulin is essential for expression of MHC class I molecules
• is non-polymorphic in humans, but dimorphic in mice (because of a single amino acid change);
• like the α3 domain, has the structure of an immunoglobulin constant region domain;
• associates with a number of other class I-like molecules, such as the products of the CD1 genes on chromosome 1 in humans (see below) and the Fc receptor, which mediates the uptake of IgG from milk in neonatal rat intestinal cells (see Fig. 3.18). These class I-like molecules, which have a structural similarity to the products of the MHC class I genes, are encoded by genes located in the class I loci and are referred to as class Ib molecules;
• is essential for the expression of all class I molecules at the cell surface – mutant mice lacking β2-microglobulin do not express MHC class I molecules and are severely defective in presenting intrinsic antigens to T cells.
Heavy chain α1, and α2 domains form the antigen-binding groove
X-ray crystallography has shown that the α1 and α2 domains constitute a platform of eight antiparallel β strands supporting two α helices (Fig. 5.6). The disulfide bond in the α2 domain connects the N terminal β strand to the α helix of the α2 domain. A long groove separates the a helices of the α1 and α2 domains.
Variations in amino acid sequence change the shape of the binding groove
Ten of the twelve differences between HLA-A2 and HLA-Aw68 are at positions lining the floor and side of the peptide-binding groove (see Fig. 5.6). These differences give rise to dramatic differences in the shape of the groove and on the antigen peptides that it will bind.
Amino acid variations within the peptide-binding groove can vary the positions of the pockets, providing the structural basis for differences in peptide-binding affinity that in turn govern exactly what is presented to a T cell (Fig. 5.7).
MHC class II molecules resemble MHC class I molecules in their overall structure
The products of the MHC class II genes are:
• the α chains have molecular weights of 30–34 kDa;
• the β chains range from 26–29 kDa, depending on the locus involved.
The β1 domain contains a disulfide bond, which generates a 64 amino acid loop.
Despite the differences in length and organization of the polypeptide chains, the overall three-dimensional structure of MHC class II molecules is very similar to that of MHC class I molecules (Fig. 5.8).
Peptide binding properties of MHC molecules
The MHC class II binding groove accommodates longer peptides than MHC class I
The structures of MHC class I and class II molecules reflect their functional differences.
The binding groove of MHC class II molecules is more open than that of MHC class I molecules, to accommodate longer peptides (Figs 5.9 and 5.10):
• MHC class I molecules bind short fragments of eight to ten amino acids; whereas
• MHC class II molecules bind peptides of 13 to 24 amino acids.
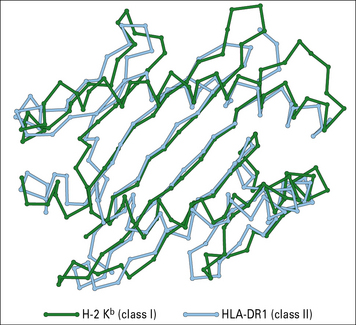
Fig. 5.9 Peptide-binding sites of class I (H-2Kb) and class I (HLA-DR1) MHC molecules
(Redrawn from Stern LJ. Structure 1994;2:245–251. Copyright 1994 with permission from Elsevier.)
Peptides are held in MHC molecule binding grooves by characteristic anchor residues
• foreign peptides from internalized antigens or viral particles; and
• self molecules produced within the cell or endocytosed from extracellular fluids.
Interactions at the N and C termini confine peptides to the binding groove of MHC class I molecules
A number of peptides bound by particular MHC molecules have been sequenced, and characteristic residues identified – one at the C terminus and another close to the N terminus of the peptide. These characteristic motifs distinguish sets of binding peptides for different MHC class I molecules (Fig. 5.11).
• the ends of the peptide-binding groove are closed;
• the peptide is an extended (not α-helical) chain of nine amino acids and the N and C terminals are buried at the ends of the groove;
• some of the side chains extend into the pockets formed within the variable region of the class I heavy chain;
• numerous hydrogen bonds are formed between residues in the class I molecule and those of the peptide along its length;
• in particular, tyrosine residues commonly found at the N terminus of the peptide and a conserved lysine in the MHC class I molecule binding groove stabilize peptide binding (see Fig. 5.10);
• the centers of the peptides bulge out of the groove, so presenting different structures to TCRs.
Peptides may extend beyond the ends of the binding groove of MHC class II molecules
The binding groove of the MHC class II molecule:
• also incorporates a number of binding pockets, though the locations are somewhat different from that on class I molecules;
• is not closed at the ends, so bound peptides extend out of the ends of the groove.
Conserved anchor residues in peptides eluted from MHC class II molecules have been identified (Fig. 5.12).
Peptides binding MHC class II are less uniform in size than those binding MHC class I molecules
• for MHC class I molecules, interactions at the N and C terminals confine the peptide to the cleft;
• for MHC class II molecules, peptides may extend beyond the ends of the cleft.
The peptides that bind to MHC class I molecules come from endogenous proteins synthesized within the cell, which are broken down and transported to the endoplasmic reticulum. The mechanism of antigen processing is explained fully in Chapter 8, but it should be noted here that the internal antigen processing pathways generally produce peptides of an appropriate size to occupy the MHC class I molecule antigen-binding groove.
The MHC class II molecule antigen processing pathway is quite distinct from the MHC class I molecule pathway (see Chapter 7).
Antigen presentation by MHC molecules
The first crystallographic data were derived using a co-crystal of a mouse MHC class I molecule bound to an endogenous cellular peptide and αβ TCR (Fig. 5.13). This structure showed that the axis of the TCR was roughly aligned with the peptide-binding groove on the MHC molecule, but set at 20–30° askew. This means that:
• the first and second CDRs of the TCR α and β chains (see Fig. 5.2) are positioned over residues near the N and C terminals of the presented polypeptide; and
• the most variable CDRs of each chain (CDR3), lying at the center of the TCR binding site, are positioned over the central residues of the peptide that protrude from the groove. Residues from each of the CDRs are positioned to interact with residues from the MHC molecule.
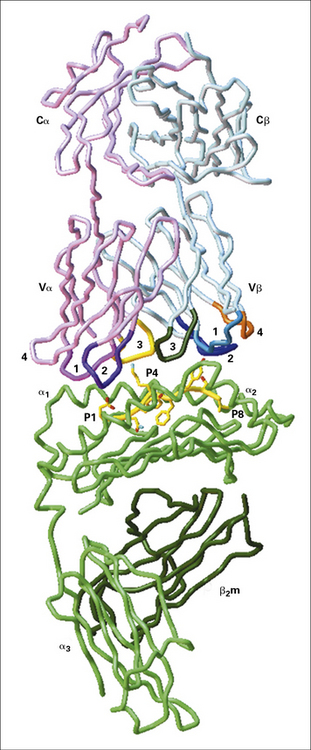
Fig. 5.13 Interaction of a T cell receptor and MHC-peptide complex
(Illustration kindly provided by Dr Christopher Garcia, from Science 1996;274:209–219. Copyright 1996 AAAS.)
Aggregation of TCRs initiates T cell activation
Although basic models of T cell activation by antigen–MHC show one receptor being triggered by one complex (e.g. Fig. 1.12), this is simplistic.
What, then, is the minimum signal for T cell activation? In practice:
• only a few peptide–MHC–TCR interactions are needed – perhaps 100 specific interactions, involving about 0.1% of the MHC molecules on the APC;
• moreover, the interactions can take place over a period of time – it is not necessary for all 100 TCRs to be engaged simultaneously.
The model of the TCR shown in Figure 5.3 suggests that it can form a dimeric structure clustered around the signaling molecules of the CD3 complex.
The auxiliary molecules CD4 and CD8 are also important in T cell activation, and the presence of CD4 or CD8 can help stabilize the interaction of the TCR and MHC–peptide. In addition, kinases associated with these molecules are brought into proximity with CD3 so they can phosphorylate the ζζ dimer that initiates activation (Fig. 5.14). The ensuing steps are described in Chapter 8.
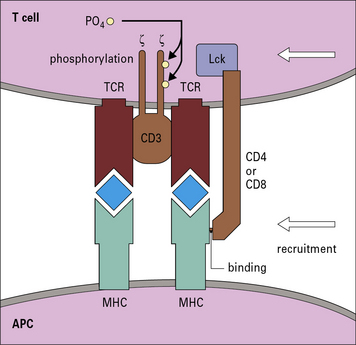
Fig. 5.14 The role of CD4 and CD8 in T cell activation
After aggregation of MHC–peptide on the APC with the TCR, either CD4 or CD8 can join the complex. CD4 binds to MHC class II molecules and CD8 to MHC class I molecules. The kinase Lck is attached to the intracytoplasmic portion of CD4 or CD8. Binding of the CD4 or CD8 molecules to specific sites of the MHC molecules brings the kinase into proximity with the ITAMs on the CD3 ζζ dimer (see Fig. 5.3). The kinase phosphorylates the motifs, as the first step in T cell activation.
Antigenic peptides can induce or antagonize T cell activation
Understanding TCR affinity could provide insight into how T cells become tolerant to self antigens during development (see Chapter 19).
What constitutes T cell specificity?
Finally we should consider the question of what constitutes T cell specificity. When the specificity of lymphocytes was first explained in Chapter 1, it was stated that each lymphocyte binds to just one antigen using its receptor. Although this is a useful starting point for understanding immune responses, it is not strictly true.
In Chapter 3 it was seen that immunoglobulins could bind to different antigens if they had epitopes that were sufficiently similar. This chapter has shown that antigenic peptides can be mutated and still bind and trigger T cell activation.
Later, when we consider how antigenic peptides from microorganisms can trigger autoimmune diseases (see Chapter 20), we learn that one possible mechanism is that a self peptide and a foreign peptide are sufficiently similar to bind to the same T cell, so causing a breakdown in self tolerance. One conclusion from the work cited above is that such peptides do not have to be identical to be cross-reactive.
Genetic organization of the MHC
The three principal human MHC class I loci are HLA-A, HLA-B, and HLA-C

Fig. 5.15 Genes within the human MHC class I region
The human MHC class I region lies telomeric to the MHC class II region (see Fig. 5.5). In addition to the genes encoding the classic transplantation antigens (HLA-A, HLA-B, and HLA-C), several other principal class I-like genes have been identified (HLA-E, HLA-F, and HLA-G). Mutations in the HLA-H gene are associated with hemochromatosis, a disorder that causes the body to absorb excessive amounts of iron from the diet. A number of other non-classical class I genes and pseudogenes are present, mostly with unknown functions.
Closer analysis of this region has revealed multiple additional MHC class I genes.
Mice have two or three MHC class I loci
The mouse MHC (H-2) has two or three MHC class I loci, but the number of MHC class I genes varies between haplotypes (Fig. 5.w2).
• the H-2K gene encodes the H-2K antigen expressed on most cell types and recognized serologically; whereas
• the H-2K2 gene exhibits varied patterns of expression, depending on the strain.
The numbers of genes in the H-2D and H-2L loci are variable (see Fig. 5.w2).
Qa, Tla, and M genes encode MHC class Ib molecules
The Qa, Tla, and M genes of the mouse MHC class I region (see Figs 5.4 and 5.w2) encode class Ib molecules, of which the functions are mostly unknown:
• the Qa locus comprises about 200 kb of DNA distal to H-2D/L and encodes the serologically detectable molecules Qa-1, -2, -3, -4, and -5, and encompasses a cluster of eight (BALB/c) to ten (B10) MHC class I genes;
• Qa-1b corresponds to HLA-E, which is involved in NK cell recognition (see Fig. 10.6);
• the Tla region, although defined initially as encoding the TL (thymus leukemia) antigen, has subsequently been shown to contain the largest number of class I genes and the greatest number of differences in organization between the B10 and BALB/c haplotypes;
• the M region contains a number of new MHC class I genes, termed M1–M7.
Human MHC class II genes are located in the HLA-D region
The HLA-D region encodes at least six α and ten β chain genes for MHC class II molecules (Fig. 5.16). Three loci (DR, DQ, and DP) encode the major expressed products of the human MHC class II region, but additional genes have also been identified:
• the DR family comprises a single α gene (DRA) and up to nine β genes (DRB1–9), including pseudogenes and several different gene arrangements occur within the locus;
• the DQ and DP families each have one expressed gene for α and β chains and an additional pair of pseudogenes.
• the DPA1and DPB1gene products associate to generate the HLA-DP class II molecules detected using specific antibodies;
The organization and length of the DRB region varies in different haplotypes (Fig. 5.17), with different numbers of β chains expressed.
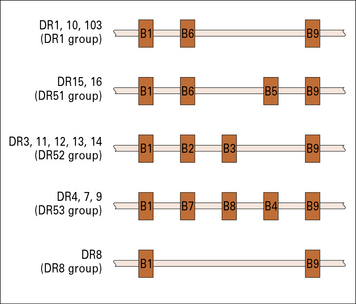
Fig. 5.17 The number of DRB loci varies with different haplotypes
The numbers of DRB loci varies between individuals. For example, a person who has a haplotype producing molecules of the type DR1 (see Appendix 1) has three loci for DRB (top line). Not all of these loci produce mRNA for DR β chains.
The MHC class II region also contains genes that encode proteins involved in antigen presentation that are not expressed at the cell surface (see Chapter 8).
Mouse MHC class II genes are located in the H-2I region
The α and β chains of mouse MHC class II molecules are encoded by separate genes located in the I region of the H-2 complex (see Figs 5.4 and 5.16). The gene nomenclature indicates first the locus and then the type of chain it encodes:
• the Ab and Aa genes thus encode the β and α chains of the A molecule; and
• the Eb and Ea genes likewise encode the two chains of the E molecule (see Fig. 5.4).
Several other class II b and a genes for which no gene product is known, have been cloned:
Different strains of mice vary in their MHC class II genes (see Fig. 5.16).
MHC polymorphism is concentrated in and around the peptide-binding cleft
Q. Correlate the domains that show structural variability and structural conservation with the molecules that they interact with
A. The α1 and α2 domains interact with the variable antigen peptides and the TCR (see Fig. 5.13), while the α3 domain interacts with the monomorphic CD8 molecule (see Fig. 5.14).
• most polymorphism occurs in DRβ and DQβ chains, whereas DPβ chains are slightly less polymorphic;
• DQα is polymorphic whereas DRα chains are virtually invariant, being represented by just two alleles.
Most of the polymorphic amino acids in MHC class I and class II molecules are clustered on top of the molecule around the peptide-binding site (see Fig. 5.6). Variation is therefore centered in the base of the antigen-binding groove or pointing in from the sides of the α helices. This polymorphism affects the ability of the different MHC molecules to bind antigenic peptides.
MHC haplotype and disease susceptibility
Genetic variations in MHC molecules affect:
• the ability to make immune responses, including the level of antibody production;
• resistance or susceptibility to infectious diseases;
• resistance or susceptibility to autoimmune diseases and allergies.
All nucleated cells of the body express MHC class I molecules
By contrast, MHC class II molecules are used by APCs to present antigens to helper T cells. Consequently the distribution of class II molecules is much more limited (see Fig. 8.4).
The specificity of the TCR and MHC explains genetic restrictions in antigen presentation
The key experiment that demonstrated the importance of the MHC in antigen presentation revealed a phenomenon called genetic restriction (also known as MHC restriction). In essence, it was noted that cytotoxic T cells from a mouse infected with a virus are primed to kill cells of the same H-2 haplotype infected with that virus; they do not kill cells of a different haplotype infected with the same virus (Fig. 5.18).
Presentation of lipid antigens by CD1
The genes encoding CD1 molecules are located outside the MHC and are not polymorphic. In humans, they consist of five closely linked genes, of which four are expressed (Fig. 5.19), encoding proteins that fall into two separate groups:
Murine CD1b has been crystallized and analyzed by X-ray crystallography. This shows that the molecule has a deep electrostatically neutral antigen-binding groove, which is highly hydrophobic and can accommodate lipid or glycolipid antigens (see Fig. 5.w3). One model for the binding places hydrophobic acyl groups of the lipids into the large hydrophobic pockets, leaving the more polar groups of the antigens such as phosphate and carbohydrate on the top, where they can interact with the TCR.
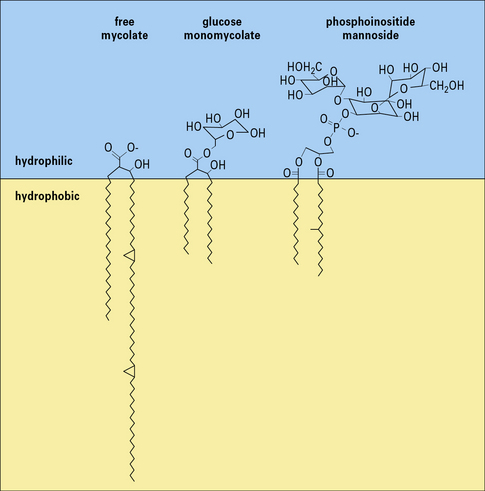
Fig. 5.w3 Glycolipid antigens presented by CD1
(Information based on Porcelli SA, Segelke BW, Sugita M, et al. Immunol Today 1998;19:362–368.)
The antigens presented by the group I CD1 molecules and CD1d are different. For example, group I molecules present lipoarabinomannan, a component of the cell wall of mycobacteria (see Fig. 14.1), whereas CD1d cannot do this.
• MHC class I molecules are loaded with antigenic peptides in the endoplasmic reticulum, and this requires transport of the peptides from the cytoplasm (see Chapter 8).
• group I CD1 molecules appear to be loaded in an acidic endosomal compartment because they do not bind to lipid antigens unless they are partially unfolded at low pH.
There is some debate about the physiological functions of the CD1 molecules in host defense:
• group I CD1 molecules present lipids from mycobacteria and Haemophilus influenzae and can stimulate both CD4+ and CD8+ cytotoxic T cells, and therefore appear to have a role in antimicrobial defense.
• most CD1d molecules appear to bind self antigens, though they also present lipids from parasites such as Plasmodium falciparum and Trypanosoma brucei (see Chapter 15) to T cells that use a restricted group of TCRs, indicating a role in defense against single-celled protozoal parasites.
Critical thinking: Somatic hypermutation (see p. 434 for explanation)
The specificity of T cells (see p. 434 for explanation)
2. Explain why the SM/J cells and the F1 cells can present antigen to the T cells, but the Balb/c cells cannot.
Peptide | Amino acid sequence | T cell activation |
---|---|---|
12–36 | QLEDARRLKAIYEKKKNELGLSQESV | − |
80–102 | SPSIAREIYEMYEAVSMQPSLRS | +++ |
73–88 | ILKVSVEEFSPSIAREIY | − |
80–94 | SPSIAREIYEMYEAVS | ++ |
84–98 | AREIYEMYEAVSMQP | − |
3. Explain why peptides 80–102 and 80–94 activate the T cells while the others do not.
4. What term is used to describe this kind of mutated peptide? What would you predict about the binding affinity of this peptide within the TCR–MHC–peptide complex?
Bentley G.A., Mariuzza R.A. The structure of the T cell antigen receptor. Annu Rev Immunol. 1996;14:563–590.
Bjorkman P.J., Parham P. Structure, function and diversity of class I major histocompatibility complex molecules. Annu Rev Biochem. 1990;59:253–288.
Bjorkman P.J., Saper M.A., Samraoui B., et al. The structure of the human class I histocompatibility antigen HLA-A2. Nature. 1987;329:506–512.
Bjorkman P.J., Samraoui B., Bennett W.S., et al. The foreign antigen binding site and T-cell recognition regions of class I histocompatibility antigens. Nature. 1987;329:512–516.
Bodmer J.G., Marsh S.E., Albert E.D., et al. Nomenclature for factors of the HLA system, 1994. Tissue Antigens. 1994;44:1–18.
Brenner M.B., MacLean J., Dialynas D.P., et al. Identification of a putative second T-cell receptor. Nature. 1986;322:145–149.
Brown J.H., Jardetzky T.S., Gorga J.C., et al. Three-dimensional structure of the human class II histocompatibility antigen HLA-DR1. Nature. 1993;364:33–39.
Burdin N., Kronenberg M. CD1-mediated immune responses to glycolipids. Curr Opin Immunol. 1999;111:326–331.
Carosella E.D., Dausett J., Kirzenbaum H. HLA-G revisited. Immunol Today. 1996;17:407–409.
Chien Y.-H., Jores R., Crowley M.P. Recognition by γδ T cells. Annu Rev Immunol. 1996;14:511–532.
Davis M.M., Bjorkman P.J. T-cell antigen receptor genes and T-cell recognition. Nature. 1988;334:395–402.
Davis M.M., Boniface J.J., Reich Z., et al. Ligand recognition by αβ T cells. Annu Rev Immunol. 1998;16:523–544.
Garcia K.C., Degano M., Stanfield R.L., et al. An αβ T cell receptor structure at 2.5Å and its orientation in the TCR–MHC. Complex Sci. 1996;274:209–219.
Garcia K.C., Teyton G.L., Wilson I.A. Structural basis of T cell recognition. Annu Rev Immunol. 1999;17:369–398.
Garratt T.P.J., Saper M.A., Bjorkman P.J., et al. Specificity pockets for the side chains of peptide antigens in HLA-w68. Nature. 1989;342:692–696.
Hass W., Pereira P., Tonegawa S. Gamma/delta cells. Annu Rev Immunol. 1993;11:637–685.
Hayday A.C. γδ cells: a right time and a right place for a conserved third way of protection. Annu Rev Immunol. 2000;18:975–1026.
Hunkapiller T., Hood L. Diversity of the immunoglobulin gene superfamily. Adv Immunol. 1989;44:1–63.
Lefranc M.-P., Rabbitts T.H. The human T-cell receptor γ (TRG) genes. Trends Biochem Sci. 1989;14:214–218.
Leiden J.M. Transcriptional regulation of T cell receptor genes. Annu Rev Immunol. 1993;11:539–570.
Madden D.R., Gorga J.C., Strominger L., et al. The structure of HLA-B27 reveals nonamer self-peptides bound in an extended conformation. Nature. 1991;353:321–325.
Manning T.C., Kranz D.M. Binding energetics of T-cell receptors: correlation with immunological consequences. Immunol Today. 1999;20:417–422.
Porcelli S.A., Segelke B.W., Sugita M., et al. The CD1 family of lipid antigen-presenting molecules. Immunol Today. 1998;19:362–368.
Powis S.H., Trowsdale J. Human major histocompatibility complex genes. Behring Inst Mitt. 1994;94:17–25.
Raulet D.H. How γδ T cells make a living. Curr Biol. 1994;4:246–251.
Roth D.B. Generating antigen receptor diversity. Lewin B., ed. Immunology module of Virtual Text. 2005. www.ergito.com. Chapter 5
Salter R.D., Benjamin R.J., Wesley P.K., et al. A binding site for the T-cell co-receptor CD8 on the α3 domain of HLA-A2. Nature. 1990;345:41–46.
San José E., Sahuquillo A.G., Bragado R., Alarcón B. Assembly of the TCR/CD3 complex: CD3 epsilon/delta and CD3 epsilon/gamma dimers associate indistinctly with both TCR alpha and TCR beta chains. Evidence for a double TCR heterodimer model. Eur J Immunol. 1998;28:12–21.
Sloan-Lancaster J., Allen P.M. Altered peptide–ligand induced partial T cell activation: molecular mechanisms and role in T cell biology. Annu Rev Immunol. 1996;14:1–27.
Stern L.J., Wiley D.C. Antigenic peptide binding by class I and class II histocompatibility proteins. Structure. 1994;2:245–251.
Stern L.J., Brown J.H., Jardetzky T.S., et al. Crystal structure of the human class II MHC protein HLA-DR1 complexed with an influenza virus peptide. Nature. 1994;368:215–221.
Weiss A., Littman D.R. Signal transduction by lymphocyte antigen receptor. Cell. 1994;76:263–274.
Zeng Z.H., Castaño L.H., Segelke B., et al. The crystal structure of mouse CD1: an MHC-like fold with a large hydrophobic binding groove. Science. 1997;277:339–345.