Synaptic transmission
General principles
Electrical synapses
A gap junction is composed of around 100 intercellular channels called connexons that are inserted into the plasma membranes of adjacent cells (Fig. 7.1). Each connexon is composed of a hexagonal array of proteins called connexins, surrounding an aqueous channel that is 2 nm wide. The pores in adjacent cell membranes are aligned to form a ‘tunnel’ between the two cells. These can be opened or closed by a conformational change in the constituent proteins, regulated by phosphorylation state.
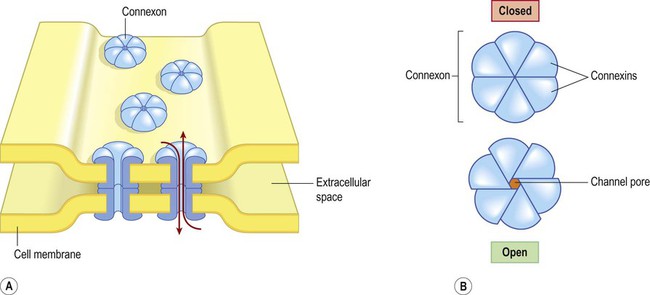
(A) Gap junctions are points of direct communication between the cytoplasm of adjacent cells, formed by protein complexes called connexons; (B) Each connexon is composed of six connexin subunits, surrounding a central pore.
Gap junctions represent a low-resistance pathway that allows charged particles and small molecules to flow freely in either direction and couples the electrical activity of adjoining cells. Groups of cells linked by gap junctions form an electrical syncytium which can generate large, synchronized discharges. This happens in certain brain stem nuclei that control breathing and may contribute to the generation of abnormally synchronized discharges in some forms of epilepsy (Ch. 11).
Chemical synapses
Most central nervous system synapses are chemical. The general structure and arrangement is similar to that of the neuromuscular junction (see Ch. 4) and a great deal of information about central synapses has been derived from experiments at the point of contact between nerve and muscle.
General structure (Fig. 7.2)
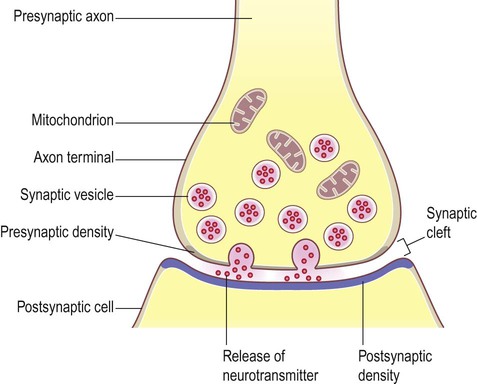
The arrangement is similar to that of the neuromuscular junction between nerve fibres and skeletal muscle.
Peptide transmitters are synthesized in the cell body and pre-loaded into vesicles which are delivered to the axon terminal by fast axonal transport (see Ch. 5). Other transmitters are synthesized within the axon terminal and are loaded into synaptic vesicles by transport pumps in the vesicle membrane. Some are synthesized within the vesicle itself.
Excitatory and inhibitory synapses (Fig. 7.3)
Synapses can be excitatory or inhibitory. If the presynaptic neuron releases an excitatory transmitter (most commonly glutamate) then the membrane of the postsynaptic cell will be depolarized. This is referred to as an excitatory post-synaptic potential (EPSP) and is typically associated with an inward sodium current. If the presynaptic neuron releases an inhibitory neurotransmitter (the most common of which is gamma-aminobutyric acid or GABA) then the postsynaptic membrane will be hyperpolarized (inhibited). This is an inhibitory postsynaptic potential (IPSP) and is often associated with an inward chloride current (or an outward potassium current). EPSPs and IPSPs are graded potentials of a few millivolts that only influence the local membrane and then rapidly decay (see Ch. 6).
Release of neurotransmitter (Fig. 7.4)
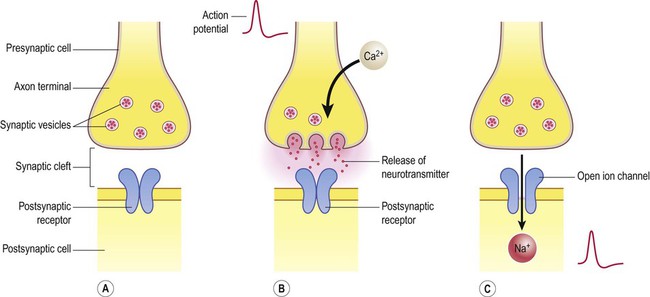
(A) Neurotransmitter molecules are stored in the presynaptic element within membrane-bound synaptic vesicles; (B) Arrival of an action potential at the axon terminal triggers calcium influx via voltage-gated calcium channels which causes the vesicles to fuse with the presynaptic membrane and discharge their contents into the synaptic cleft; (C) The transmitter diffuses across the synaptic cleft and interacts with receptors on the postsynaptic membrane, leading to ion fluxes that will either inhibit or (as in this case) excite the postsynaptic neuron.
Neurotransmitters
Classical neurotransmitters
Classical, small molecule neurotransmitters are stored in clear-cored vesicles that are approximately 50 nm in diameter. The first to be identified was acetylcholine (ACh) and it remains the best understood. Acetylcholine is found at the neuromuscular junction and throughout the autonomic nervous system. It differs from other types of neurotransmitter and has a distinct chemical structure, biosynthesis and mechanism of inactivation (Fig. 7.5). Acetylcholine is synthesized in axon terminals from two metabolites that are present in all cells: acetyl-coA and choline. This is achieved by the enzyme choline acetyltransferase (ChAT) which is only present in cholinergic neurons. The remaining small molecule neurotransmitters are divided into amino acids and biogenic amines.
Amino acid transmitters
The majority of fast neurotransmission in the central nervous system involves amino acid neurotransmitters. The principal excitatory transmitter of the CNS is glutamate (together with the related amino acid aspartate, which acts at the same receptors). The main inhibitory transmitter of the brain and spinal cord is gamma-aminobutyric acid (GABA). It is synthesized from glutamic acid by the enzyme glutamic acid decarboxylase (GAD) which removes one of its two carboxylic acid groups (Fig. 7.6). Another amino acid, glycine, is an important inhibitory transmitter in the spinal cord.
Biogenic amines
This group of neurotransmitters includes noradrenaline (norepinephrine), adrenaline (epinephrine) and dopamine. These transmitters are produced from the amino acid tyrosine by decarboxylation (removal of the carboxylic acid group) leaving a single amino group (–NH2) to form a monoamine. They are classified as catecholamines due to the presence of a catechol ring, which is composed of six carbon atoms. The common biosynthetic pathway for the catecholamines is illustrated in Figure 7.7.
Serotonin (5-hydroxytryptamine, 5-HT) is also a biogenic amine, but contains an indole group which has a bicyclic (or two-ring) structure rather than a single catechol ring. It is therefore classified as an indolamine. Serotonin is synthesized from the amino acid tryptophan and its pathway for biosynthesis and degradation is separate from that of the catecholamines (Fig. 7.8).
Postsynaptic receptors
Types of receptor
Metabotropic receptors
These channels are linked to GTP-binding proteins (G-proteins) which influence the cell via effector proteins which may be ion channels, enzymes or elements within an intracellular cascade (Fig. 7.9). For instance, receptor binding may activate an enzyme that alters the phosphorylation state of the target: activation of kinases leads to phosphorylation (addition of phosphate group) whereas phosphatases have the opposite effect. If the target is an ion channel, alteration of the phosphorylation state may open or close it (Fig. 7.10). Alternatively, intracellular cascades may lead to changes in second messengers such as cyclic AMP (cAMP, the concentration of which is controlled by the enzyme adenylate cyclase) or cytosolic free calcium levels. In some cases there are long-term changes in gene expression caused by activation of nuclear transcription factors. Metabotropic receptors are therefore responsible for long-term neuromodulation rather than fast neurotransmission.
Glutamate receptors
Glutamate is synthesized and stored in perisynaptic glia and in the neuronal axon terminal. It acts both at ionotropic and metabotropic receptors, which can be assembled from an array of subunits (Fig. 7.11).
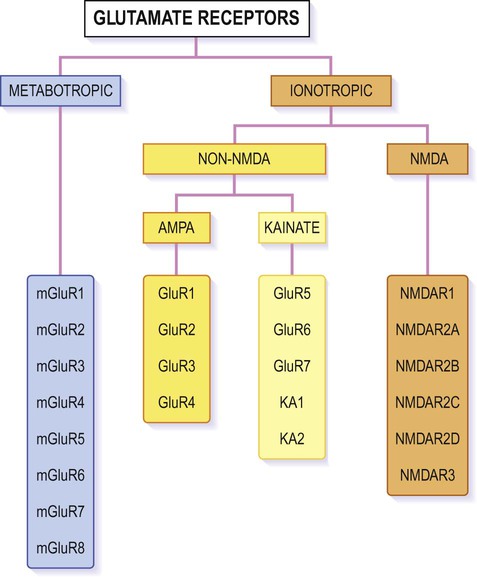
The figure shows the various subunits from which individual receptors of each type can be assembled, in various combinations. Ionotropic receptors are tetrameric, whereas metabotropic receptors form heterodimers. Most AMPA receptors incorporate the GluR2 subunit which makes them impermeable to calcium; those lacking the GluR2 subunit behave more like NMDA receptors and take part in learning, memory and excitotoxicity.
Ionotropic glutamate receptors
NMDA receptors and calcium
At the resting membrane potential the ion channels of NMDA receptors are inactivated by magnesium ions (Mg2+), as shown in Figure 7.12. Once the neuronal membrane has been depolarized and this magnesium block is lifted, sodium and calcium ions are able to enter the cell. NMDA receptors therefore have the unique property of being both ligand-gated and voltage-sensitive. Also, because of their high calcium permeability, they are able to effect long-term changes in neuronal biochemistry by initiating downstream, calcium-dependent events.
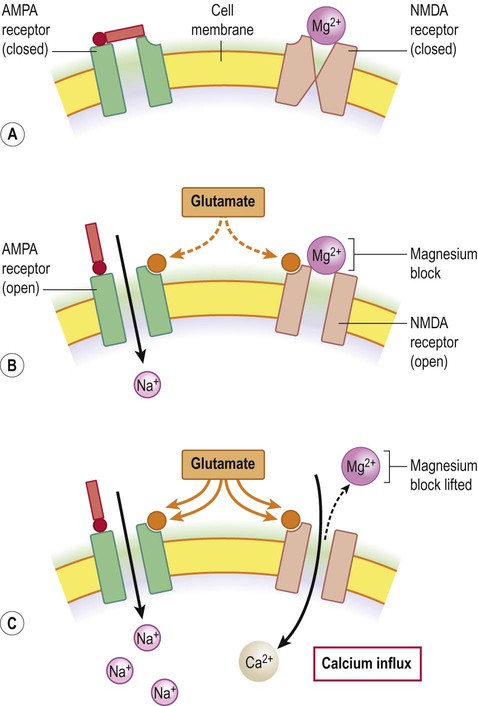
(A) NMDA receptors co-exist with non-NMDA (e.g. AMPA) receptors in the neuronal membrane; (B) Stimulation by glutamate opens AMPA receptors which depolarizes the cell by admitting sodium ions. NMDA receptors are also stimulated but the channel pore is occluded by magnesium ions; (C) With prolonged stimulation the magnesium block is lifted (as magnesium ions are repelled from the mouth of the channel pore by the increasingly positive interior face of the membrane). Once this has happened, calcium is able to enter the cell via the liberated NMDA channels.
Metabotropic glutamate receptors
Numerous G-protein-coupled glutamate receptors have been identified, many of which consist of a heterodimeric assembly of subunits (selected from mGLUR1-8; see Fig. 7.11). They fall into three main groups, but the majority are postsynaptic and excitatory. Some are presynaptic autoreceptors which help to regulate transmitter release at the synapse.
Memory and learning
Associative phenomena
The mechanism of long-term potentiation, which depends upon controlled elevation of free cytosolic calcium, explains why glutamate is neurotoxic at high levels. Very strong glutamatergic stimulation leads to excessive calcium influx via NMDA receptors and GluR2-negative AMPA receptors, which damages the cell and may trigger programmed cell death. This process is termed excitotoxicity and is important in several nervous system disorders (see Ch. 8). Glutamate is therefore described as an excitotoxin when present at abnormally high concentrations.
GABA receptors
Ionotropic GABA receptors
The GABAA receptor is the target of several anti-anxiety drugs and its action is also modulated by alcohol (Clinical Box 7.1). It is a pentameric chloride ion channel assembled from more than a dozen varieties of alpha, beta, gamma and delta subunits. The reversal potential is around –65 mV, so that activation tends to maintain the hyperpolarized (inhibited) state of the cell.
Synaptic integration
Spatial and temporal summation
The impact of a single excitatory postsynaptic potential on a target neuron is small, but if a number of convergent impulses from different nerve cells all reach the postsynaptic neuron together then the combined effect off these disparate influences may be sufficient to depolarize the cell to threshold and generate an action potential (Fig. 7.13). This is referred to as spatial summation.
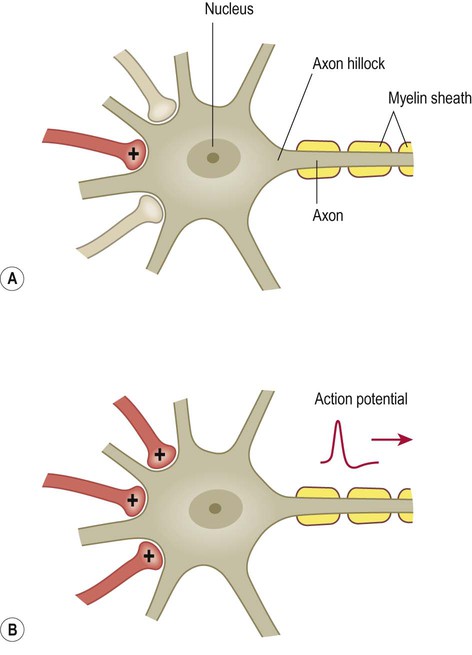
Excitatory projections from one or a small number of afferent fibres may be insufficient to cause depolarization in a target neuron (A), but it may be possible to trigger an action potential if several relatively weak impulses converge on the same target cell (B).
Similarly, although the influence of a single excitatory or inhibitory postsynaptic potential is small, the graded potential takes up to 15 milliseconds to decay – and may be additive if several nerve impulses arrive in rapid succession. This is referred to as temporal summation (Fig. 7.14) which operates together with spatial summation.
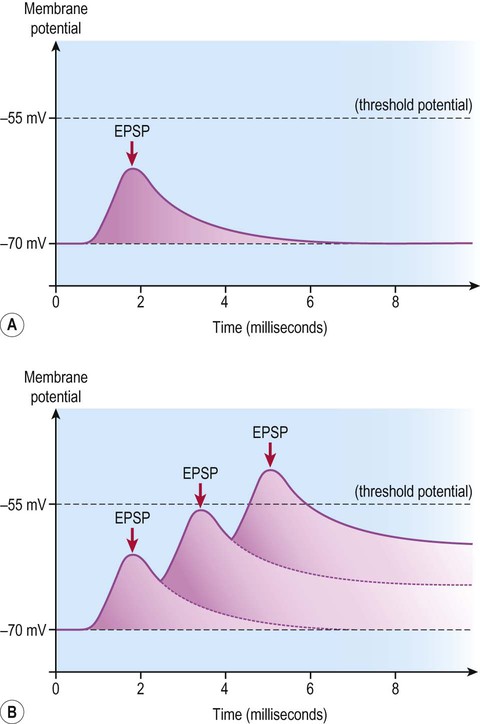
A single, relatively weak excitatory postsynaptic potential (EPSP) may be insufficient to reach the threshold for generation of an action potential (A), but if several excitatory postsynaptic potentials arrive in quick succession (before the previous ones have completely decayed) then they may be additive and take the cell membrane to threshold (B).
The trigger zone for action potential generation is the initial segment of the axon. Summation of excitatory and inhibitory influences in this region of the membrane is critical for nerve impulse generation, so incoming synapses that are closer to this area stand a better chance of influencing neuronal firing rate than those at the distant reaches of the dendritic tree (Fig. 7.15).
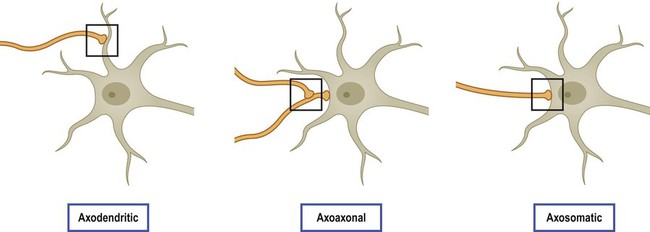
Since action potentials are triggered at the initial segment of the axon, synapses that are closer to this point (on the proximal dendrites or soma, rather than at the distant reaches of the dendritic tree) have a stronger influence on the target cell.
Some neurons exert a more powerful excitatory or inhibitory effect on their targets by making direct synaptic contact with the axon terminal. This is termed presynaptic facilitation or presynaptic inhibition, illustrated in Figure 7.16.