Chapter 17 Stereotactic Irradiation
Linear Accelerator and Gamma Knife
Radiosurgery was first described in 1951 by Lars Leksell,1 a neurosurgeon at the Karolinska Institute in Stockholm, Sweden. The term radiosurgery was selected because of the similarity of this technique to stereotactic neurosurgery. The initial work by Leksell first involved the treatment of patients with functional disorders and benign conditions such as chronic pain and arteriovenous malformations but later included benign and malignant tumors. The first patient was treated with the Gamma Knife in 1968.2 The limited availability, skepticism regarding the technology, and prohibitive costs initially limited the spread of Gamma Knife technology. By the 1980s, linear accelerator-based radiosurgery had evolved and further renewed the interest in stereotactic radiosurgery.
Basic Principles Of Radiosurgery
Radiosurgery can be performed using various devices, including the multicobalt unit known commercially as the Gamma Knife (GK), particle beam devices, or modified linear accelerators. With technologic advances in software and hardware, a clear superiority of one technology over another has disappeared and mature clinical results demonstrate no outcome differences based on technology.3 A comparison of some of the features of the Gamma Knife and linear accelerator techniques is presented in Table 17-1. Because the linear accelerator-based units can also treat non-radiosurgery patients during “downtimes,” cost and volume considerations are key features that determine equipment selection.3
TABLE 17-1 Comparing Gamma Knife and Linear Accelerator Radiosurgery Characteristics
Gamma Knife | Linac Radiosurgery | |
---|---|---|
Clinical experience | Over three decades | Over two decades |
Accuracy | Submillimeter | Submillimeter |
QA | Fewer QA checks | More QA checks |
Machine use | Dedicated machine | Usually not a dedicated machine |
Functional disorders | Longer experience | Shorter experience |
Can be used for extracranial radiosurgery | No | Yes |
Price | High; must replace source every 5-7 years | Less expensive; no source replacement |
Tumor location | Difficult to radiate peripheral lesions | Can treat peripheral lesions |
Fractionated treatments | Not practical | Can treat with fractionated regimens |
Treatment time | About equal | About equal |
QA, quality assurance.
Radiosurgical and neurosurgical approaches are often complementary, but there are key differences. Radiosurgery does not require a craniotomy or general anesthesia and is performed on an outpatient basis. In a cost-effectiveness/cost-utility analysis by Mehta and colleagues4 in the 1990s, the authors showed that the average cost per week of survival for a single brain metastasis was $310 for radiotherapy, $524 for resection plus irradiation, and only $270 for radiosurgery plus whole-brain irradiation. With the advent of shorter hospital stays following neurosurgical procedures, these numbers have probably changed somewhat. Other advantages include lower postoperative risks for bleeding or infection and rapid recovery times. Patients who are working before treatment often can return to work within 1 or 2 days. More importantly, neurosurgically inaccessible lesions and patients deemed unfit for surgery or anesthesia can often be treated with radiosurgery.
The clinical applications of radiosurgery have grown substantially over the past decade. There are multiple disease processes where the use of stereotactic radiosurgery has a well-established and defined role. Current indications, discussed later in greater detail, include arteriovenous malformations, benign brain tumors, malignant brain tumors, and functional disorders (Table 17-2). With improved ability to localize targets throughout the body, the principles of radiosurgery have expanded to include extracranial applications, with emerging roles in the management of lung, hepatic, and spinal tumors, among others. Some concepts of the radiosurgery paradigm have been incorporated into a strategy employing fractionated treatments; this approach, referred to as fractionated stereotactic radiotherapy (FSRT), is applied to both intracranial and extracranial lesions.
Radiosurgery is an important tool available to the neurosurgeon and radiation oncologist. Proper implementation of the treatment requires collaboration among neurosurgeons, radiation oncologists, and medical physicists. This allows for thoughtful coordination of care, improved quality assurance, reduction in practice variation, a strategic marketing advantage, and improved patient satisfaction. Each specialty brings unique skills that are required for a successful radiosurgery program.5
Physics Of Radiosurgery
Stereotactic radiosurgery (SRS) involves the use of numerous beamlets of radiation aimed precisely at an immobilized target to deliver a single session of high-dose radiation. Though no single beamlet carries significant weight, a large dose is deposited at the intersection of these beamlets with a steep dose fall-off outside the target. As tumor size increases, this fall-off becomes shallower6; typically, radiosurgery becomes prohibitive at sizes in excess of 4 to 5 cm.
Physics Fundamentals
Multicobalt units containing cobalt-60 (60Co) sources include devices such as the Gamma Knife and the Rotating Gamma System (developed in China) (Figs. 17-1 and 17-2). 60Co decays with a half-life of 5.26 years, requiring that sources be replaced every 5 to 7 years. For new sources, total activity is approximately 6000 Ci with a dose rate of 400 cGy/minute. Radioactive decay releases two gamma rays with an average energy of approximately 1.25 MeV. A gamma ray and an x ray cannot be discriminated and differ only in source of origin. Gamma rays result from radioactive decay, whereas x rays are generated by accelerating electrons (in a linear accelerator) and colliding them into a tungsten target.
Components of a Radiosurgery Unit
Both multicobalt and linear accelerator units have at their heart a source of radiation (cobalt for the Gamma Knife [GK] and the linear accelerator for linac-based units), a couch, a stereotactic helmet or frame system to ensure precise localization and immobilization, and sophisticated treatment planning software. When properly calibrated, an isocenter alignment to better than 1 mm can be achieved with either the GK or the linac radiosurgery system, although it is important to recognize that image resolution, which depends on the slice thickness, is usually of a cruder magnitude.7
Pretreatment Quality Assurance
An in-depth description of the various linac radiosurgery systems is beyond the scope of this chapter, and the interested reader is referred to Task Group Report 54 of the American Association of Physicists in Medicine.7 In general, linac radiosurgery systems can be classified into three groups. First, there are couch-mounted systems that use the treatment lasers or implanted fiducial markers as their target verification system; these systems are probably the least accurate because they have an isocenter stability of at best ±2 mm for any couch and gantry angle. Next, there are the so-called floor stand–mounted systems that connect to the treatment couch and allow the user to correct for inaccuracies in the rotation of the couch during treatment. However, these systems do not allow for corrections of inaccuracies in gantry rotation and, therefore, such systems have at best an isocenter stability of ±1 mm for any couch and gantry angle. The most accurate linac radiosurgery system uses a floor stand with a high-precision bearing that is indexed to the linac but does not connect to the treatment couch. In this system, the floor stand is decoupled from the linac treatment couch; this allows for a high accuracy of couch rotation, on the order of a few hundredths of a millimeter. Furthermore, this system has a separate arm rotating around a high-precision bearing that holds the collimator, which is coupled to the linac gantry using a gimbal bearing. Because all translational and rotational degrees of freedom are decoupled from the linac, this system can be calibrated to have an isocenter stability that is equal to that of a GK of ±0.25 mm for any gantry and couch angle.8
Radiobiologic Considerations
Radiobiologically, the use of a single fraction to treat a small volume differs significantly from conventional fractionated external beam irradiation (EBRT) procedures. This poses unique challenges in that the increased biologic effect on normal tissues is more pronounced than it is on tumors9 (Fig. 17-3). The most important factor influencing the risk of developing late side effects is fraction size. Therefore single-fraction radiosurgery needs to be exquisitely accurate and must minimize the dose to normal tissue.
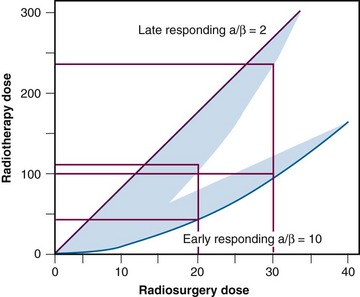
Figure 17-3 Representation of radiosurgery versus radiotherapy dose for both early- and late-responding tissues.
From Mehta MP: The physical, biologic, and clinical basis of radiosurgery, Curr Probl Cancer 19(5):265-329, 1995.
Despite these theoretical disadvantages of single-fraction radiation, efficacy with minimal toxicity has been shown in clinical trials in a multitude of disease sites. Radiosurgery, by treating in a single-fraction regimen, minimizes the deleterious effects of repopulation (tumor regrowth between fractions). Further, the high doses of radiation could potentially overcome the disadvantages of single-fraction delivery. Recent observations suggest the DNA-centric model of classical radiobiology is more complex than initial experiments appreciated, including effects on membrane-bound signaling pathways.10 It appears that radiation effects on the tumor microvasculature are also an important component of tumor response and control.11 Higher ablative doses of radiation have been shown to induce marked endothelial apoptosis with substantial downstream effects on the competency of tumor microvasculature.11 At doses of more than 8 Gy per fraction, the linear quadratic (LQ) model does not accurately predict cell kill or toxicity.12 This poses significant clinical challenges in developing dose-volume normal tissue constraints for SRS and stereotactic body radiotherapy (SBRT), as the LQ model is often used to account for differences in dose per fraction when extrapolating from toxicity analyses obtained using conventional regimens.
Treatment Planning
Both GK and conventional linac-based SRS treatment planning procedures begin with the placement of the stereotactic head frame on the patient. The head frame is used for precise localization and rigid immobilization to guarantee that the patient’s position from the time of imaging to the time of treatment does not change. Before the stereotactic CT is acquired, a stereotactic localizer ring is attached to the head frame. For linac radiosurgery, the most commonly used independent stereotactic localization system is the Brown-Roberts-Wells (BRW) system (Fig. 17-4). The BRW localizer has an inner diameter of 29 cm and a height of 16 cm and consists of nine carbon fiber rods that are arranged into three N-shaped structures between two aluminum rings, which are placed at 120-degree angles around the rings. Because three N-shaped fiducials are used, one obtains a system of linear equations that allow for definition of an independent, absolute coordinate system. Therefore one does not have to QA the table indexing of the CT scanner, because the slice position of each scan can be uniquely determined.
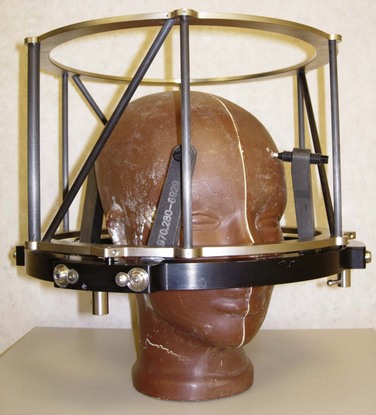
Figure 17-4 Phantom with head ring as well as the Brown-Roberts-Wells (BRW) coordinate system in place.
The origin of the BRW system lies within the localizer and therefore divides the brain into eight distinct quadrants. Any point within this geometric configuration can be uniquely defined by specifying anterior-posterior, lateral, and axial coordinates. During the stereotactic CT scan, portions of these N-shaped structures are included in each image, which allows for their identification during treatment planning. To reduce the error in localization, the slice thickness of the CT scan should be the smallest attainable on the scanner. Once the stereotactic CT scan has been acquired, it is transferred to the treatment planning system and the BRW coordinate system is defined by localizing each of the nine rods in each of the CT slices (Fig. 17-5).
Next, the target volume, or gross tumor volume (GTV), is outlined. Because magnetic resonance imaging (MRI) often provides better tumor visualization than CT scanning, the GTV is often delineated directly from MRI studies. MRI alone does not provide a sufficient database for stereotactic treatment planning, because magnetic field nonuniformity, gradient field nonlinearity, eddy current effects, and susceptibility artifacts at air/tissue interfaces can introduce significant geometric image distortions that affect the accuracy of treatment plans generated using MRI alone. A three-dimensional volumetric MRI is acquired either before or after head ring placement. After acquiring the stereotactic CT scan, the MRI dataset (which may or may not be stereotactically generated) is fused onto the CT image space through correlation of anatomic landmarks in both image sets. During treatment planning, the regions of interest and dose distribution may be displayed on either the CT image or the fused MRI images, but dosimetric calculations are ideally performed on the underlying CT database (Fig. 17-6). If the MRI is to be obtained after head frame placement, magnetic resonance–compatible head rings need to be used.
Conventional linac radiosurgery uses multiple non-coplanar arc sets to treat the target volume through a variety of collimator diameters ranging in size from 4 mm to 50 mm, depending on the system (Fig. 17-7). For irregularly shaped lesions such as vestibular schwannomas and arteriovenous malformations (AVMs), arcs delivered through a single circular collimator would lead to the inclusion of a large amount of normal brain tissue, which would yield inferior conformality. In these cases, it is advantageous to use multiple isocenters to conform the prescription isodose shell to the GTV (Fig. 17-8). The dose is typically prescribed to the 70% to 90% isodose line. Whenever multiple isocenters are used, homogeneity is sacrificed for conformality. Ideally, a desirable conformality index (prescribed isodose volume divided by target volume) should be 2 or less and the heterogeneity index (maximum dose divided by prescribed dose) should also be 2 or less.
Clinical Applications
Both the GK and linac-based forms of radiosurgery have been used in a variety of benign and malignant diseases. Table 17-2 summarizes the most common clinical applications for radiosurgery. In this section we outline some of the basic applications of radiosurgery and results of therapy. Specifics pertaining to each disease are discussed in greater detail in its respective chapter.
Benign Tumors
Meningiomas
Two of the largest series that have examined results of SRS are from the Mayo Clinic and the University of Pittsburgh, both of which have shown local control in more than 90% for benign meningiomas at 5 years.13,14,15 The Mayo Clinic series had a significant complication rate of 13% versus 7% for the University of Pittsburgh series.
Based on published reports, the optimal dose to the margin of the tumor appears to be 12 to 16 Gy. A recent report by Kollova and colleagues16 showed that doses above 16 Gy increased the risk of treatment-related edema without a corresponding improvement in tumor control. Inclusion of the dural tail within the treatment volume remains a topic of controversy. Limited histopathologic analysis has shown that microscopic tumor extension into the dural tail is limited to 2 mm.17 The most common toxicities include cranial nerve deficits for basal tumors and peritumoral edema for nonbasal tumors. The risk of optic neuropathy is very low, with maximum dose constraints to the optic nerves and chiasm of between 8 and 10 Gy.13,18 In addition to the dose, a treatment volume of more than 5 cm3, a tumor-brain contact interface of 1 cm2 or more, the presence of pretreatment edema, and parasagittal location each increase the risk of peritumoral edema.16,19,20,21 These factors should be considered when deciding whether or not to include none of the dural tail or only a portion of it within the clinical target volume (CTV), which for all practical purposes is the same as the GTV. With SRS, radiologic response is seen in 50% to 60% of patients, and the remainder of those without progression demonstrated tumor stability.16,22
Based on these data, it is reasonable to conclude that small meningiomas can be controlled with radiosurgery in the majority of patients, with initial results comparable to those of complete resection (Fig. 17-9). It must, however, be borne in mind that most cases in which this approach has been used have had relatively short follow-up studies and that long-term results (more than 10 years of follow-up studies for each patient) are indeed very sparse. The University of Pittsburgh recently updated their 18-year experience in a cohort of 972 patients. Local control rates in grade 1 meningioma or lesions without histology were 91% and 95%, respectively, in 75 patients with a minimum follow up of 10 years.