CHAPTER 21 Secretory Membrane System and Golgi Apparatus
Eukaryotic cells transport newly synthesized proteins destined for the extracellular space, the plasma membrane, or the endocytic/lysosomal system through a series of functionally distinct, membrane-bound compartments, including the endoplasmic reticulum (ER), Golgi apparatus, and vesicular transport intermediates. This is the secretory membrane system (Fig. 21-1), which allows eukaryotic cells to perform three major functions: (1) distribute proteins and lipids synthesized in the ER to the cell surface and other cellular sites, (2) modify and/or store protein and lipid molecules after their export from the ER, and (3) generate and maintain the unique identity and function of the ER, Golgi apparatus, and plasma membrane. This chapter describes how the secretory membrane system is organized and operates to fulfill these functions. It also provides a detailed description of the Golgi apparatus whose conserved features are central for the operation of the secretory membrane system.
Overview of the Secretory Membrane System
Newly synthesized transmembrane and lumenal proteins transported through the secretory system are called cargo. These include lumenal proteins destined to be stored within a compartment or secreted to the cell exterior, as well as transmembrane proteins that are retained in a particular compartment (e.g., Golgi processing enzymes), delivered to the plasma membrane, or recycled among compartments (e.g., transport machinery). Transfer of cargo molecules through the secretory system begins with their cotranslational insertion into or across the ER bilayer (see Fig. 20-7). The cargo molecules are next folded and assembled into forms that can be sorted and concentrated within membrane-bound transport intermediates (called vesicular tubular carriers [VTCs]) destined for the Golgi apparatus. Once packaged into and transported by such a carrier, cargo enters the Golgi apparatus, which serves as the central processing and sorting station in the secretory membrane system. Within the Golgi apparatus, numerous enzymes modify the cargo molecules by trimming or elongating the cargo’s glycan side chains or cleaving its polypeptides. Processed cargo is then sorted into membrane-bound carriers that bud out from the Golgi apparatus and move to the plasma membrane, to the endosome/lysosomal system, or back to the ER. In specialized cell types, the Golgi apparatus can sort certain classes of cargo into secretory granules (for storage and later release to the cell exterior in response to specific stimuli) or give rise to transport carriers that target to different polarized plasma membrane domains.
Membrane-enclosed carriers mediate transport within the secretory membrane system (Fig. 21-2). Carriers are shaped as tubules, vesicles, or larger structures. The carriers are too large to diffuse freely in the crowded cytoplasm but are transported over long distances along microtubules or actin filaments by molecular motor proteins. Each carrier selects certain types of cargo before budding from a donor compartment and fuses only with an appropriate target membrane. Molecular markers on the cytoplasmic surface of the carrier, as well as on the acceptor membrane, steer the carrier through the cytoplasm and ensure that it fuses only with the correct target compartment. The carriers continuously shuttle among ER, Golgi apparatus, and plasma membranes, enabling cargo to be distributed to its appropriate target organelle.
During transport of a carrier, the relative orientation (called topology) of lipid and protein in the membrane bilayer, established during synthesis in the ER, is maintained (Fig. 21-2). Hence, one side of the membrane always faces the cytoplasm. The other side initially faces the lumen of the ER. This side remains inside each membrane compartment along the secretory pathway but is exposed on the cell surface if the carrier fuses with the plasma membrane. Selection of proteins and lipids by a carrier, budding of the carrier, and subsequent fusion of the carrier with an acceptor compartment all also occur without leakage of contents from the carrier or the donor and target compartments.
The flow of cargo and lipid forward through the secretory system toward the plasma membrane (anterograde traffic) is balanced by selective retrograde traffic of cargo and lipids back toward the ER (Fig. 21-1). Retrograde traffic allows proteins and lipids involved in membrane transport and fusion to be retrieved for repeated use. Retrograde traffic also returns proteins that have been inadvertently carried forward through the secretory system so they can be redirected to their proper destination. Both anterograde and retrograde flows of membrane within the secretory system are necessary for the ER, Golgi apparatus, and plasma membrane to generate and maintain their distinct functional and morphologic identities.
Advantages of the Secretory Membrane System
A third advantage relates to the differentiation of the plasma membrane. Prokaryotic cells synthesize their proteins at the plasma membrane, so they must keep this surface enriched in loosely packed glycerophospholipids that are pliable enough that newly synthesized proteins can enter into and fold in a hydrophobic environment. Consequently, prokaryotic cells secrete a rigid cell wall as a protective barrier to the outside. In eukaryotes, concentrating protein synthesis in the ER frees the plasma membrane to become enriched in lipids such as cholesterol and sphingolipids that can arrange into highly ordered, flexible arrays. The ordered, flexible arrays of cholesterol and sphingolipids in the plasma membrane provide mechanical stability and an impermeable barrier to water-soluble molecules. As a consequence, eukaryotic cells do not require a cell wall to survive (although some eukaryotes, such as plant and fungal cells, make cell walls) and can employ their plasma membrane in a wide range of functions, such as membrane protrusion for engulfing large extracellular objects (see Chapter 22) and for crawling (see Chapter 38).
Building and Maintaining the Secretory Membrane System
Protein Sorting by the Lipid Gradient across the Secretory Membrane System
A conserved feature of the secretory membrane system is the differential distribution of various classes of lipids along the pathway. These classes of lipids include glycerophospholipids (phosphoglycerides), sphingolipids (e.g., sphingomyelin and glycosphingolipids), and cholesterol (see Figs. 7-4 and 20-13). These lipids play a major role in the sorting of proteins within the secretory membrane system because of their immiscibility (i.e., the property of not mixing) in membranes with different lipid compositions. By not mixing with some lipids while mixing with others, these lipid classes form lateral lipid assemblies, termed microdomains, that can concentrate or exclude specific membrane proteins.
Studies using artificial membranes have demonstrated how lipid immiscibility allows a continuous lipid bilayer to self-organize into distinct lipid domains with unique lipid compositions and biophysical properties. A prime example is an artificial bilayer containing glycerophospholipids and cholesterol to which sphingolipid is added; after sphingolipid is added, the cholesterol and glycerophospholipids partition into distinct domains (Fig. 21-3A–B). Because of van der Waals attraction between the sphingolipid’s long, saturated hydrocarbon chain and cholesterol’s rigid, flat-cylindrical steroid backbone, the cholesterol and sphingolipids associate in the plane of the membrane, whereas glycerophospholipids, which have unsaturated, kinked hydrocarbon chains with much less affinity for cholesterol, are largely excluded from the cholesterol/sphingolipid domains. The domains enriched in cholesterol/sphingolipid are thicker than the surrounding membrane composed of shorter, unsaturated, kinked glycerophospholipids (Fig. 21-3C). Tension on the bilayer (i.e., from binding of proteins that bend or curve the membrane) enhances the tendency of lipids that have different physical properties to separate into distinct phases.
In addition to prompting separation of sphingolipids from glycerophospholipids, cholesterol can affect a bilayer composed of glycerophospholipids alone (Fig. 21-3 D). In this case, the cholesterol fills the space between the floppy hydrocarbon chains of glycerophospholipids in the bilayer. This forces the glycerophospholipids into a tighter alignment and increases the distance between their head groups. As a result, the bilayer becomes thicker, resembling the thickness of bilayers enriched in sphingomyelin alone or sphingomyelin plus cholesterol.
Sphingolipids (e.g., glycosphingolipids and sphingomyelin) are synthesized in the Golgi apparatus, while the ER produces cholesterol and glycerophospholipids. Synthesis of these lipids at two different sites, combined with the self-organizing capacity of sphingolipids, cholesterol, and glycerophospholipids, gives rise to a pattern of lipid circulation within the secretory system that plays important roles in membrane sorting (Fig. 21-4A). Newly synthesized cholesterol is continually removed from the ER and redistributed to the Golgi apparatus, where high affinity interactions with sphingolipids prevent it from returning to the ER. The association of cholesterol with sphingolipids in the Golgi apparatus, in turn, triggers the lateral differentiation of domains enriched in these lipids. Through the additional activity of protein-based sorting and trafficking machinery, these domains bud off the Golgi apparatus and move to the plasma membrane, redistributing sphingolipids and cholesterol to the cell surface.
The forward flow of cholesterol, sphingolipids, and glycerophospholipids toward the plasma membrane is balanced by selective retrograde flow. Glycerophospholipids transferred from the ER to the Golgi apparatus are recycled back to the ER. Similarly, sphingolipids delivered to the plasma membrane from the Golgi apparatus are returned to the Golgi apparatus. Cholesterol, in contrast, is not returned through these retrograde pathways to either the ER or the Golgi apparatus but enters and circulates within the endocytic pathway leading to lysosomes. This pattern of lipid circulation creates a gradient of cholesterol, sphingolipids, and glycerophospholipids across the secretory membrane system. Within this gradient, the ER has a low concentration of cholesterol (e.g., sterols) and sphingolipids, the Golgi apparatus has an intermediate concentration, and the plasma membrane has a high concentration (Fig. 21-4 A).
A second function of the lipid gradient is to promote sorting of transmembrane proteins within the secretory system. Each integral membrane protein seeks a lipid bilayer with a thickness that matches the lengths of its transmembrane segments (Fig. 21-4B–D). Because most transmembrane segments are stiff hydrophobic a-helices, it is energetically unfavorable to expose hydrophobic residues of a transmembrane polypeptide to the aqueous environment of the cytoplasm or vesicle lumen or to bury hydrophilic amino acids with the lipid acyl chains in the interior of the membrane. To avoid such hydrophobic mismatches, integral membrane proteins of the secretory system have evolved with transmembrane segments that are matched to the thickness of their target membranes. Hence, resident membrane proteins in the ER and Golgi apparatus typically have shorter transmembrane segments (around 15 amino acids) than do resident plasma membrane proteins (approximately 20 to 25 amino acids). Retention and/or transport of these proteins occurs because the lipid bilayers of carriers budding out from either the ER (toward the Golgi apparatus) or the Golgi apparatus (toward the plasma membrane) are thicker than the bilayers of the donor organelles. Only transmembrane proteins with transmembrane segments long enough to span this thickness enter such carriers.
This lipid-based protein sorting mechanism takes advantage of the lipid gradient established by the self-organizing properties of glycerophospholipids, cholesterol, and sphingolipids to sort and transport proteins within the secretory system. It is not, however, the only mechanism used by cells to organize and transport proteins along the secretory pathway. In addition, a complex protein-based machinery is relied on to bring far greater specificity and efficiency to these processes.
Protein-Based Machinery for Protein Sorting and Transport within the Secretory Membrane System
Sorting and transporting proteins within the secretory membrane system depend on several types of proteins (Fig. 21-5): Specialized “coats” help to generate both small and large transport carriers and sort proteins into them; motor proteins move carriers along the cytoskeleton; “tethering factors” attach carriers to the cytoskeleton and to their destination organelles prior to fusion; and fusion proteins mediate fusion of the carrier with an acceptor membrane. These components also associate with specific organelles, providing organelles with an identity that is both unique and dynamic. Many of the components are peripheral membrane proteins that lack transmembrane domains, so they must be recruited to the cytoplasmic surface of appropriate membranes by binding to either specific lipids, such as phosphoinositides, or to activated GTPases. Cells regulate the distributions of these organelle-specific lipids and GTPases. When infectious agents or stressful conditions disrupt these targeting molecules, secretory membrane trafficking can be disorganized and/or inhibited. The following sections describe the six major protein-based mechanisms that are used for sorting, transport, and fusion in the secretory membrane system.
Arf GTPases
Like other GTPases (see Figs. 4-6 and 4-7), Arfs are molecular switches that alternate between a GTP-bound active form that interacts with effector targets and a GDP-bound inactive form that does not (Fig. 21-6). Active Arf GTPases associate with membranes, where-as inactive GTPases are cytoplasmic. Specific GTP exchange factors (GEFs) recruit Arf proteins to particular membrane surfaces and then catalyze the exchange of GDP for GTP. When associated with particular membranes active Arfs bind their effectors until a GTPase-activating protein (GAP) induces hydrolysis of GTP, reversing membrane association and effector binding. The distribution of GEFs on particular membranes determines the location of specific active Arfs. Similarly, the location of GAPs determines where each type of Arf is inactivated.
Activation of Arfs by exchange of GDP for GTP not only creates a binding site for target proteins (i.e., effectors) but also promotes interaction with the lipid bilayer. A myristoyl group covalently bound to the N-terminus of most Arfs allows them to interact transiently and nonspecifically with membranes. When a specific Arf-GEF on a membrane catalyzes the exchange of GDP for GTP, an amphipathic (hydrophobic on one side, hydrophilic on the other) N-terminal, a-helix is released from a hydrophobic pocket on the GTPase so that the hydrophobic side of the helix can interact with the bilayer (Fig. 21-6 D). The membrane-associated GEFs that are responsible for activating Arfs all contain an evolutionarily conserved domain (referred to as the Sec7 domain). Association of this domain with Arf1-GDP is stabilized in the presence of the toxic fungal metabolite brefeldin A (BFA [Fig. 21-6 D]). This prevents Arf1 conversion to its active, GTP-bound state and thereby blocks Arf1 activity, similar to that of a GDP-locked Arf1 mutant.
Arf GTPases of the secretory pathway, in particular Sar1 and Arf1, recruit to membranes many types of effector proteins. These include the coat protein complexes of COPII, COPI, and clathrin/adapters plus other effectors such as phospholipid modifiers (e.g., phospholipase D, a lipid metabolizing enzyme), phosphoinositides, and cytoskeletal components. The coat protein complexes assemble into large polymeric structures (called protein coats) at the cytoplasmic surface of ER, pre-Golgi, and Golgi membranes, from which they sort cargo and promote the budding of transport carriers. The other Arf effectors play roles in differentiating the membrane environment of these carriers and enabling them to move to different locations within the cell. The four other mammalian Arf proteins (Arfs 2 to 6) regulate vesicle formation at other locales in the exocytic and endocytic pathways.
Sar1 assembles the COPII coat complex that is involved in differentiating ER export domains, which are the sites from which transport carriers bud out from the ER. Arf1, by contrast, assembles the COPI coat complex that is involved in the creation of retrograde transport carriers that bud from pre-Golgi and Golgi structures. Arf1 also recruits the clathrin/adapter coat complexes that are involved in budding of transport carriers from the Golgi en route to the endosome/lysosomal system. Disruption of the GTPase cycles of either Sar1 or Arf1 has dramatic consequences for secretory transport and the organization of the secretory pathway (Fig. 21-14). When the GTPase cycles of Sar1 or Arf1 are disrupted, the Golgi apparatus disassembles, and Golgi enzymes return to the ER or to ER exit sites with all secretory transport out of the ER inhibited.
The COPII Coat
The COPII coat complex (Fig. 21-7) is essential for sorting and trafficking secretory cargo out of the ER. It consists of Sar1p GTPase, the Sec23p˙Sec24p subcomplex, and the Sec13p˙Sec31p subcomplex. These components self-assemble into a polymeric, two-dimensional scaffold (called a coat) that then collects specific types of cargo. The intrinsic curvature of the coat promotes the formation of membrane buds that are capable of pinching off the membrane as coated vesicles.
COPII coats assemble by a sequential process (Fig. 21-7 E). A GEF called Sec12p recruits Sar1p-GDP from the cytoplasm to the ER membrane and activates it by exchanging GDP for GTP. Activated Sar1p-GTP then recruits the two COPII subcomplexes: Sec23p˙Sec24p, which is embedded within the ER membrane, and Sec13p˙Sec31p, which is soluble in the cytoplasm. The Sec13p˙Sec31p subcomplexes bound to Sec23p˙Sec24p polymerize into a mesh-like scaffold that coats the membrane. The coat starts as a small aggregate but grows larger as more Sec23p˙Sec24p subcomplexes (which are in direct contact with Sar1p in the membrane) diffuse in from surrounding membrane and become cross-linked by Sec13p˙Sec31p subcomplexes recruited from the cytoplasm. As the lattice grows in size, it bends the patch of membrane into a coated bud that recruits specific types of proteins. The coated bud pinches off as a coated carrier (containing concentrated proteins) or remains as a “metastable” coated structure that participates in the differentiation of membrane domains at ER exit sites.
The Sec24p component of COPII coats recognizes several types of sorting signals in the cytoplasmic domains of transmembrane cargo proteins (Fig. 21-8). These include diacidic motifs that fit the consensus asp-glu-x-asp-glu (DExDE) and short hydrophobic motifs such as phe-phe (FF), phe-tyr (FY), leu-leu (LL), or ile-leu (IL). Some transmembrane cargo proteins lack these sorting signals, raising the question of how these cargo proteins (as well as luminal cargo proteins) are sorted into COPII-coated buds at ER export sites. One possibility is that transmembrane proteins containing these sorting signals escort cargo proteins lacking COPII-sorting signals to COPII-containing ER exit domains. Other transmembrane proteins may be exported from the ER by virtue of having longer transmembrane segments that partition into the potentially thicker bilayers of ER export domains.
The COPI Coat
The COPI coat complex (Fig. 21-9) is found on the cytoplasmic face of pre-Golgi (also called vesicular tubular carrier [VTC]) and Golgi compartments and helps to mediate protein sorting and retrograde transport from these structures back to the ER. This is crucial for these structures to functionally and morphologically differentiate from the ER. Like the COPII coat complex, the COPI coat complex assembles into a lattice (i.e., COPI coat) on a patch of membrane, recruits specific proteins, deforms the membrane patch into a coated bud, and then pinches off as a coated carrier or remains as a “metastable” coated structure.
The formation of the COPI coat (Fig. 21-9 B) begins with a small, soluble GTPase, Arf1, binding to the membrane and recruiting a preformed coatomer complex (Fig. 21-9 A) from the cytoplasm. The coatomer complex consists of at least seven subunits, ranging in mass from 25 to more than 100 kD. Coatomer bound to Arf1 then attracts from the cytoplasm Arf-GAP1, the GTPase-activating protein for Arf1. This complex of a GTPase, a GAP and coatomer is the basic building unit of the COPI coat on membrane. Interactions between coatomer subunits and the cytoplasmic tail of transmembrane cargo proteins concentrates the cargo proteins as the coat polymerizes.
COPI units (consisting of Arf1, coatomer, and GAP) polymerize into a coat by addition of other COPI units diffusing in the plane of the membrane. The COPI coat bends the membrane as it forms a basket-like lattice. Arf-GAP1 is inactive during diffusion of individual units, but curved membranes favor its interaction with Arf1-GTP and hydrolysis of the GTP. (Sar1 and its GAP respond similarly to membrane curvature.) Thus, assembly of the COPI coat on membranes automatically inactivates Arf1, which is released from membranes, destabilizing the lattice of coatomer and Arf-GAP1. This leads to coat disassembly.
A consequence of these dynamic events is that COPI units move into the lattice from the rims and are released from the interior after Arf1 hydrolyzes its GTP, paralleling events occurring in the COPII coat. This results in a continuous flux of coat units through the lattice whether or not a coated vesicle detaches from the membrane. This dynamic behavior of coat units allows for several outcomes (Fig. 21-10A–C). The lattice can grow, disassemble (after budding off the membrane as a coated vesicle), or persist as a coated bud. In the latter case, the rate of addition of coat units to the bud is equal to the rate of unit loss. These behaviors of the coat lattice play key roles in orchestrating the protein sorting and morphologic events that occur at ER export domains to allow for VTC formation (Fig. 21-10 D).
Rab GTPases
The Rab family of GTPases are the molecular switches that control the protein-protein interactions between transport carriers and docking complexes on target membranes (Fig. 21-11). These complexes recruit motor proteins that transport carriers on actin filaments or microtubules and then tether carrier vesicles to an organelle prior to fusion. Mammals express about 70 different Rab proteins to provide specificity at numerous transport steps in the secretory membrane systems of various cell types.
Rab proteins cycle between the cytoplasm, where they are found in the GDP-bound form, and membranes, where they contain bound GTP (Fig. 21-11). In the cytoplasm, Rabs are complexed with a carrier protein called a guanine nucleotide dissociation inhibitor (GDI), which prevents exchange of GDP to GTP. GDI also sequesters the hydrophobic geranylgeranyl groups. Proteins called GDI displacement factors facilitate Rab recruitment to membranes by displac-ing GDI.
Tethering Factors
Tethering factors are rod-shaped proteins that extend about 15 nm from membranes into the cytoplasm (Fig. 21-11). They tether membrane carriers to target organelles prior to fusion and play structural roles as components of a Golgi matrix or scaffold for the assembly of other factors important for fusion and/or cargo sorting. Heterogeneous in sequence and structure, tethering factors can be divided into two general classes:
SNAP Receptor Components
The SNAP receptor (SNARE) family of proteins participates in the fusion of carriers with their appropriate acceptor compartment (Fig. 21-12). Most SNAREs are transmembrane proteins with their functional N-terminal domains in the cytoplasm and their C-termini anchored to the bilayer. Each contains a heptad repeat (i.e., “SNARE motif”) of 60 to 70 amino acids that can form a coiled-coil. Multiple SNAREs assemble a SNARE complex consisting of a bundle of α-helices. Members of the SNARE protein family were originally grouped according to whether they were v-SNAREs or t-SNAREs, referring to whether they conferred function to the vesicle (v-SNARE) or target (t-SNARE) compartment. For example, synaptobrevin is a v-SNARE found on synaptic vesicles involved in neurotransmission (see Fig. 11-9), whereas syntaxin 1 is a t-SNARE found on presynaptic densities to which synaptic vesicles fuse to trigger neurotransmitter release.
The formation of a SNARE complex occurs by the pairing of cognate v- and t-SNAREs. This generates a four-helix bundle with one α-helix contributed by one SNARE and the other three α-helices contributed by an oligomerized t-SNARE. The four-helix bundle of SNAREs depends on interactions of an arginine from one helix with glutamines from three other helices. This requirement has led to an alternative classification of these proteins as either R-SNAREs or Q-SNAREs, based on the presence of these critical arginine (R) or glutamine (Q) residues.
Fusion of a carrier with a target membrane transforms a trans-SNARE complex into a cis-SNARE complex on the cytoplasmic face of the fused membrane. Following completion of fusion, the cis-SNARE complex is disassembled by a ubiquitous AAA ATPase (see Box 36-1) called NSF (for N-ethyl maleimide [NEM]–sensitive factor). The sulfhydryl alkylating reagent NEM inactivates NSF and prevents all carrier transport in the cell. SNAP proteins recruit NSF to the membrane. NSF uses the energy from ATP hydrolysis to dissociate the cis-SNARE complex and recycles the SNAREs for another round of membrane fusion.
Most SNARE proteins are anchored to membranes by a transmembrane segment that inserts into ER membranes after translation (i.e., they are tail-anchored proteins; see Chapter 20). Thus, SNARES must traverse the secretory pathway to reach specific organelles, but little is known about the mechanism they use. The length of their transmembrane domains and their capacity to interact with protein coats are likely to be important. For example, SNAREs involved in ER to Golgi transport are packaged into COPII coats at ER export sites for delivery to the Golgi apparatus, where they mediate homotypic fusion (i.e., fusion of two like transport containers that have identical cis-SNARE pairs) among incoming carriers as well as heterotypic fusion (i.e., fusion of two distinct membrane structures that have different cis-SNARE pairs) of these carriers with the Golgi membrane. The SNAREs are then packaged into COPI coats for retrieval to the ER. This allows them to function repeatedly in ER-to-Golgi apparatus transport.
Secretory Transport from the Endoplasmic Reticulum to the Golgi Apparatus
Transport of newly synthesized proteins out of the ER takes place in specialized areas called ER export domains. These structures are approximately 1 to 2 μm in diameter and appear in fluorescent images as dispersed, punctate structures that are scattered over the surface of the ER (Fig. 21-13A). An individual ER export domain is organized into two zones (Fig. 21-13B-C). One is a region of smooth ER membrane studded with COPII-coated buds and uncoated tubules. The other is a central cluster of vesicles and tubules with the capacity to detach and traffic to the Golgi apparatus. The ER membrane is continuous between these two zones until the vesicle-tubule cluster and its associated cargo detach from the ER and move to the Golgi apparatus as a transport intermediate, called vesicular tubular carrier (VTC) (Fig. 21-10 D). Cargo proteins are actively sorted into ER export domains through binding of signal motifs within their cytoplasmic tails to the COPII coat, and/or by lateral partitioning into the specialized lipid environment of this region. Partitioning is thought to occur once the transmembrane segments of the cargo proteins match the thickness of the ER exit site lipid bilayer.
The morphologic and biochemical differentiation of the ER export domain into a motile VTC is a multistep process orchestrated by the sequential action of the Sar1, Rab1, and Arf1 GTPases and their effectors (Fig. 21-14). Sar1 GTPase initiates ER export domain formation through COPII-mediated sorting of specific integral membrane proteins (including the p24 family proteins and SNAREs) and the formation of coated buds. The presence of coated buds and specialized cargo in this region, together with the membrane tension produced by the coated buds, leads to changes in bilayer lipid composition. This, in turn, promotes partitioning of other transmembrane proteins into the ER export domain, including proteins with longer-than-average transmembrane domains that lack COPII recognition motifs in their cytoplasmic domains. Additional cytoplasmic proteins are then also recruited to the ER exit site, including Rab1 and p115, which interact with tethering factors (such as GM130 and giantin), SNARE proteins, and GBF1 (the GEF for Arf1). Together, these molecules stimulate the membrane budding and fusion events that differentiate the ER export domain and VTC. The SNARE proteins, for example, allow the COPII-coated vesicles and membrane tubules that bud out from the smooth ER to fuse with themselves to form a tubule cluster; GM130 and giantin tether these membranes to the cytoskeleton; and Arf1 effectors differentiate the membrane further by initiating retrieval of specific proteins back to the ER. Disruption of the GTPase cycle of Sar1 through expression of a GDP-locked form prevents ER export domain formation, whereas disruption of the GTPase cycle of Arf1—through expression of a GDP-locked form of Arf1 or by BFA treatment—blocks VTC formation (Fig. 21-14). By blocking membrane delivery into the secretory pathway, both treatments also cause the disappearance of the Golgi apparatus, which depends on continuous membrane input to maintain its structure.
Detachment of the VTC from the ER export domain and its maturation and delivery to the Golgi apparatus are the next steps in protein trafficking from ER to Golgi apparatus. Mammalian cells use motors to detach VTCs from ER export domains and to carry them along microtubules toward the Golgi apparatus located near the microtubule-organizing center (Fig. 21-1). During this process, the VTC matures by a process that is orchestrated by Arf1 and its effectors. Activated Arf1 recruits dozens of cytoplasmic proteins to VTCs (and to Golgi membranes). Among these, the COPI coat binds to and clusters specific proteins, enabling them to be retrieved back to the ER. Lipid-modifying enzymes such as phosphatidylinositol kinases and phosphatases create a lipid environment that is distinct from that in the ER membrane, permitting tethering factors and matrix proteins to bind to the motile VTC membrane. Ankryrin and spectrin proteins (see Fig. 7-10) form a scaffold for other cytoskeletal proteins, including actin, tubulin, dynactin, and dynein. Among these, the dynactin complex (see Fig. 37-2) mediates dynein-dependent clustering of VTCs by movement on microtubules toward centrioles at the center of the cell.
Sorting from the Trans-Golgi Network
After transport through the Golgi system, cargo leaves the trans or exit face of the Golgi apparatus (Fig. 21-15). The exit region is called the trans-Golgi network (TGN) because of its tubular network organization. This organization is characteristic of other sorting compartments, such as that of the VTC, the cis Golgi, and sorting endosomes (see Chapter 22). Depending on the cell type, the cargo that arrives in the TGN can be distributed, via distinct transport carriers, to several different intracellular locations, including the plasma membrane or cell exterior, the endosome/lysosomal system, or specialized secretory organelles or granules. The intracellular route taken by each protein depends on sorting properties that are encoded in the polypeptide chain.
Constitutive Transport of Cargo to the Plasma Membrane or Cell Exterior
A steady stream of both proteins and lipids from the TGN to the cell surface occurs constitutively through tubular transport carriers that bud out from the TGN (Fig. 21-16). No known coat proteins function in the formation of these structures. Instead, cargo proteins conveyed to the plasma membrane by these structures have transmembrane segments that partition into lipid domains containing sphingolipids and cholesterol. Activation of specific lipid-modifying enzymes such as phosphatidylinositol 4-kinase in the sphingolipid/cholesterol-enriched sorting domain of the TGN then results in the domains forming tubules that pinch off the TGN. Because the tubules have a higher volume-to-surface ratio than small vesicles, bulk soluble markers are also carried to the plasma membrane by these structures. Tubule extension is facilitated by motors moving on microtubules and/or by actin filaments, while tubule severing is mediated by dynamin-2, a GTPase localized in TGN. In mammalian cells, motor proteins such as kinesins move the constitutive membrane carriers outward from the Golgi apparatus along microtubules. Fusion of the carriers with the plasma membrane releases cargo within the lumen of the carrier vesicle into the extracellular space. After fusion, membrane lipids and proteins redistribute laterally by diffusion in the plane of the plasma membrane.
Sorting to the Endosome/Lysosomal System
Proteins that are sorted into the endosome/lysosomal system (Fig. 21-17) include a large and diverse class of hydrolytic enzymes contained within lysosomes, the digestive centers of the cell (see Chapter 23). Newly synthesized hydrolytic enzymes are prevented from entering constitutive tubular carriers destined for the plasma membrane by their binding to mannose-6-phosphate receptors (MPRs [Fig. 21-17 A]). MPRs are integral membrane proteins with a single transmembrane domain. The luminal domain binds individual prohydrolase molecules that have been modified with mannose-6-phosphate (M6P), whereas the cytoplasmic domain encodes sorting motifs that interact with the clathrin/adapter sorting machinery (see Chapter 22) that directs packaging into carriers as they leave the TGN destined for endosomes. After MPRs discharge their cargo, other carriers transfer the unoccupied MPRs back from the endosome to the TGN (Fig. 21-17).
AP1 complexes direct clathrin coat assembly at the TGN (Fig. 21-17 B). They interact directly with either tyrosine-based or dileucine sorting motifs on the MPR receptor tail. Recruitment and assembly of the AP1-containing clathrin coat on the TGN occur through direct interaction with the same small guanosine triphosphate (GTPase) Arf1 that also triggers COPI assembly (Fig. 21-6). The assembly of the clathrin-AP1 coat drives receptor clustering and budding off the TGN membrane of clathrin-coated transport vesicles. Several lysosomal membrane proteins are also sorted into these clathrin-coated vesicles by virtue of tyrosine-based sorting motifs on the cytoplasmic domains of the membrane protein. After budding off the TGN in clathrin-AP1-coated vesicles, both lysosomal hydrolases and lysosomal membrane proteins are delivered to lysosomes by way of endosomal intermediates.
Secretory Granule Formation and Transport
An additional sorting pathway from the TGN occurs in specialized endocrine, exocrine, or neuronal cells that concentrate and package selected proteins in storage granules for eventual mobilization and discharge from the cell in response to hormonal or neural stimulation. This is the so-called regulated secretory pathway (Fig. 21-18), which is used for discharging most of the body’s polypeptide hormones, enzymes used in the digestive tract, and many other products that are needed intermittently rather than continuously.
Our mechanistic understanding of secretory granule formation and sorting processes is hindered by the apparent lack of a universal sorting signal on proteins that are destined for inclusion into regulated secretory granules. Instead, secretory granule formation appears to involve physical sorting, selective retention, and condensation (Fig. 21-19). Condensation of luminal content during secretory granule biogenesis involves charge neutralization, protein aggregation and active extrusion of ions.
In some cells that produce and store peptide hormones, aggregation involves only selected products of proteolytic processing of hormone precursors. For example, production of insulin requires proteolytic enzymes in immature granules that cleave proinsulin at two sites, generating insulin and C-peptide. Insulin condenses with zinc ion in the granule core, whereas C-peptide is excluded and so accumulates around this core. As a consequence, more C-peptide is shed into unstimulated secretory pathways that originate from the immature granule. Very tight regulation of insulin secretion is important for controlling the glucose concentration in the blood plasma. This regulation is compromised in certain forms of diabetes.
Trafficking to the Plasma Membrane in Polarized Cells
In contrast to nonpolarized cells, polarized cells have functionally (and thus compositionally) distinct apical and basolateral domains separated by tight junctions that cement neighboring cells together and prevent diffusion between the domains (Fig. 21-20). Most of our knowledge of membrane sorting in polarized cells has come from studying epithelial cells. As expected, the trafficking complexity increases as destination options increase, and three distinct mechanisms for the polarized sorting of plasma membrane proteins have been revealed (Fig. 21-21). One mechanism involves selective packaging of apically or basolaterally destined proteins into distinct carrier vesicles at the TGN for delivery to the appropriate surface. A second mechanism involves the random delivery of newly synthesized proteins to both surfaces, followed by selective retention or depletion so that, at steady state, they become differentially abundant because they are more stable at one surface than at the other. A third mechanism involves delivery of newly synthesized proteins to the basolateral surface, followed by selective internalization, sorting in the endosomal compartment, and de-livery to the apical surface in a process termed trans-cytosis. Most epithelial cells use different combinations of these three mechanisms to generate and maintain cell polarity.
Direct targeting uses basolateral targeting signals in the cytoplasmic domains of proteins to sort these molecules during secretory transport or during endocytosis by recycling the proteins from endosomes back to the appropriate membrane domain. Examples include receptors for low-density lipoprotein, transferrin, MPRs, and polymeric immunoglobulin receptor. Alternatively, direct targeting occurs by lateral partitioning of proteins into sphingomyelin- and cholesterol-rich subdomains (called lipid rafts) (see Fig. 7-7) formed in the TGN or at the plasma membrane. GPI-anchored proteins or other integral membrane proteins that directly associate with these lipid rafts based on physical properties of their transmembrane domains are selectively targeted to the apical surface. The unique physical properties of these lipid subdomains render them resistant to detergent solubilization.
Regulated Fusion with the Plasma Membrane
All transport carriers leaving the TGN contain components of the vesicle targeting and fusion machinery (i.e., v-SNAREs, members of the synaptobrevin/VAMP family, Rab proteins) required to direct their fusion with the appropriate target organelle containing cog-nate t-SNAREs (members of the syntaxin, SNAP, and Sec1p families). Secretory granules carry additional regulatory factors that are superimposed on this constitutive machinery for docking and fusion. This ensures that fusion takes place only on demand. Regulated fusion has been studied extensively in the context of synaptic vesicle release, in endocrine cells, and in mast cells. In all cases, regulated secretion can be divided into three steps: docking, priming, and fusion (Fig. 21-22). Docking is the slowest step and is believed to involve interactions of v-SNARE and t-SNAREs regulated by Rab GTPases. In vitro reconstitution studies have suggested a role for a phosphatidylinositol transfer protein, a phosphatidylinositol 5-kinase, and phosphatidylinositol 4,5-bisphosphate (PIP2, the product of PI-5 kinase), in priming steps required for regulated secretion in neuroendocrine cells. A cytoplasmic protein, CAPS (calcium activator protein for secretion), is recruited to the secretory vesicle via interactions with PIP2 and is required for calcium-triggered fusion of dense core secretory granules. In most cases, fusion is triggered by an influx of Ca2+, a process called calcium-secretion coupling. Synaptotagmins, part of a family of transmembrane vesicle proteins that also bind calcium and interact with the fusion machinery, are believed to act as clamps, inhibiting fusion until calcium triggers their release.
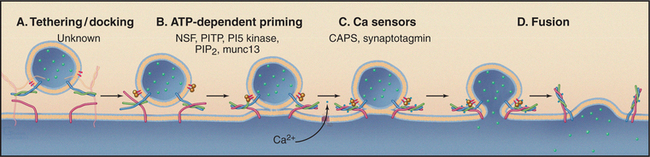
Figure 21-22 Four terminal steps (A–D) in ca2+-triggered membrane fusion during regulated secretion. Docking/tethering and fusion are mechanistically similar to other vesicle fusion events (Fig. 21-15). Additional steps prepare proteins on both the secretory vesicles and plasma membrane to respond rapidly to Ca2+ influx, which triggers fusion. CAPS, calcium activator protein for secretion; munc 13, mammalian homolog for Caenorhabditis elegans UNC13 (unknown function, critical for Ca2+-triggered fusion of primary vesicles); NSF, NEM-sensitive factor; PTP, phosphatidylinositol transfer protein.
Diverse signals lead to the calcium influx that triggers fusion. These include ligand activation of G-protein-coupled receptors on neuroendocrine cells, activation of immunoglobulin E receptors and kinase cascades in mast cells (see Fig. 28-5), and membrane depolarization in neurons (see Figs. 11-8 and 11-9).
The Golgi Apparatus: Function, Structure, and Dynamics
The Golgi apparatus (Fig. 21-23) performs three primary functions within the secretory membrane system. First, it acts as a carbohydrate factory in which glyco-proteins, polysaccharides (in plants) and proteoglycans received from the ER are further processed. Such processing permits these molecules to participate in numerous specialized biological functions at the cell surface. Second, the Golgi apparatus functions as a protein-sorting station for the delivery of proteins to many different destinations within the cell. This includes transport to the plasma membrane, secretion to the cell exterior, sorting to the endosome/lysosomal system, or retrieval back to the ER. Third, the Golgi apparatus serves as the site where sphingomyelin and glycosphingolipids are synthesized within the cell. These lipids are capable of packing tightly in the membrane, which causes the bilayer to thicken and be less permeable to water-soluble molecules (Fig. 21-3). Affinity of these lipids for each other when cholesterol is present furthermore results in the formation of discrete membrane microdomains called lipid rafts that can concentrate or exclude specific membrane proteins. Such domains can serve as platforms for the association of diverse signaling molecules and can initiate the formation of transport carriers that bud out from the Golgi apparatus.
Golgi Morphology and Dynamics
The Golgi apparatus in many animal cells appears as a ribbon-like structure adjacent to the nucleus and close to centrosomes, which are the microtubule organizing centers of the cell (Fig. 21-23 A). In electron microscope images, the Golgi apparatus exhibits a distinctive morphology consisting of a series of stacked, flattened, membrane-enclosed cisternae that resemble a stack of pancakes (Fig. 21-23 B). Cross-linking of cisternae by Golgi-associated tethering factors results in their tight, parallel alignment within the stack. Tubules and vesicles at the rims of the stacks interconnect the stacks into a single ribbon-like structure by a process dependent on microtubules. If microtubules are experimentally depolymerized, the ribbon-like Golgi structure reorganizes into single stacks (i.e., fragments) found at ER exit sites (Fig. 21-24). This distribution resembles the distribution of Golgi stacks in plant cells. There, hundreds of single stacks are localized adjacent to ER exit sites rather than being joined together as a single ribbon.
The stacks of Golgi cisternae in animal and plant cells all exhibit a cis to trans polarity that reflects the passage of cargo through this organelle. As was mentioned before, proteins from the ER enter at the stack’s cis face (entry face). After passing through the cisternae in the middle of the stack, cargo then leaves the Golgi at the trans face, which is at the opposite end of the stack. Membrane sorting and transport activities of the Golgi are thought to be especially high at the cis and trans faces and within the tubular-vesicular elements (noncompact zone) that interconnect the stacks (Fig. 21-23B).
The size, the appearance, and even the existence of the Golgi apparatus depend on the amount and speed of cargo movement through the secretory pathway. The yeast Saccharomyces cerevisiae, for example, has a poorly developed Golgi apparatus because secretory transport is normally too fast for elaborate Golgi structures to accumulate. However, conditions that slow cargo transport out of the Golgi apparatus in these cells lead to the Golgi apparatus enlarging and rearranging into compact stacks similar to those seen in most animal and plant cells.
The transient and dynamic association of mole-cules with the Golgi apparatus makes this organelle sensitive to malfunctions of many cellular systems. As mentioned before, experimental depolymerization of microtubules causes the pericentriolar Golgi apparatus of a mammalian cell to become relocated adjacent to ER export domains (Fig. 21-24 B). This occurs because Golgi enzymes that are undergoing continuous recycling back to the ER cannot return to the pericentriolar region in the absence of microtubules. Instead, they accumulate together with Golgi scaffolding, tethering, and structural coat proteins at ER export domains. Given hundreds of ER export domains scattered across the ER, hundreds of distinct Golgi elements appear within the cell upon microtubule depolymerization.
A more dramatic example of the sensitivity of the Golgi apparatus to membrane trafficking perturbations is the Golgi’s response to the drug BFA (brefeldin A). BFA prevents Arf1 from exchanging GTP for GDP (Fig. 21-6 D) and thereby prevents the membrane recruit-ment of cytoplasmic Arf1 effectors. Within minutes of BFA treatment, resident transmembrane proteins of the Golgi are recycled to the ER where they are retained, and the Golgi apparatus vanishes. On BFA washout, the Golgi apparatus reforms by outgrowth of membrane from the ER.
The Golgi apparatus disassembles during mitosis in many eukaryotic cells and then reassembles in interphase (Fig. 21-25). This process superficially resembles the effects of BFA and BFA washout, since many Golgi enzymes return to the ER or to ER export sites during mitosis and reemerge from the ER at the end of mitosis. Furthermore, Arf1 is inactivated during mitosis. However, mitotic cells also inactivate mitotic kinases (see Chapter 40) that phosphorylate tethering factors and other matrix proteins of the Golgi apparatus. This has led to a competing explanation for Golgi disassembly during mitosis in which the Golgi undergoes a direct breakdown into small vesicles and fragments without being absorbed into the ER.
Golgi-Specific Processing Activities
Glycoprotein and Glycolipid Processing
Much of the organization and specialization of the Golgi apparatus is directed toward achieving the correct glycosylation (i.e., sugar modification) of proteins and lipids. The sugar-modified molecules, called glycoproteins and glycolipids, constitute the majority of cell surface and extracellular proteins and lipids, and participate in numerous biological functions, including cell-cell and cell-matrix interactions, intracellular and intercellular trafficking, and signaling.
The most widely recognized glycosylation event occurring within the Golgi involves the modification of N-linked oligosaccharides on glycoproteins (Fig. 21-26). These N-linked sugar chains are added as preformed complexes (consisting of 14 sugar residues) to asparagine side chains of the protein in the ER. Following delivery to the Golgi, the N-linked sugar chains of the glycoprotein undergo extensive further modifications in an ordered sequence. The first modification is the removal of mannose residues. This is followed by the sequential addition of N-acetylglucosamine, the further removal of mannoses, the addition of fucose and more N-acetylglucosamine, and the final addition of galactose and sialic acid residues. Cell biologists have used the N-linked glycan-processing steps that take place in the mammalian Golgi apparatus as experimental signposts for the passage of glycoproteins through the secretory pathway.
Transporters first transfer sugar-nucleotide donors made in the cytoplasm into the lumen of the Golgi apparatus (see Chapter 9). They function as antiporters (see Fig. 9-4), exchanging nucleotide sugars (such as UDP-N-acetylglucosamine, UDP-galactose, and CMP-N-acetylneuramic acid) for nucleoside monophosphates formed during glycosyl transfer. Glycosyltransferases then use the high-energy sugar-nucleotides as substrates to add new sugars to an oligosaccharide chain. Most glycosyltransferases are specific for sugar-nucleotide donors and particular oligosaccharide acceptors, but the oligosaccharides are synthesized without a tem-plate, so they vary more than polypeptides and polynucleotides, which are synthesized on templates. Finally, glycosidases trim sugars from the branched core oligosaccharides prior to addition of other sugars. They include mannosidase I and II, which clip outer-branch mannose residues on N-linked oligosaccharides prior to the addition of N-acetylglucosamine.
The Golgi enzymes also add oligosaccharides to the hydroxyl groups of serine and threonine residues of selected proteins, such as proteoglycans, heavily glycosylated proteins in secretory granules, and the extracellular matrix (see Figs. 29-13 and 29-14). This process, called O-linked glycosylation, begins with the addition of one of three short oligosaccharides to selected serine and threonine residues of a proteoglycan core protein. Glycosyltransferases in the Golgi then add many copies of the same disaccharide unit to the growing polysaccharide. Other enzymes then add sulfates to a few of the sugar residues before the molecule exits the Golgi system.
Enzymes in the Golgi apparatus also mark specific proteins for transport to lysosomes by phosphorylation of the 6-hydroxyl of mannose. This modification, as was mentioned before, is the sorting signal that enables lysosomal enzymes to interact with MPRs in the trans Golgi for targeting to lysosomes. The N-linked oligosaccharides on these enzymes are initially processed within the ER by trimming of glucose and mannose residues. However, on transport to the Golgi, they become the unique substrates for two enzymes that act sequentially to generate terminal mannose 6-phosphates, which are the lysosomal targeting signal. Human patients with the fatal disease mucolipidosis II (called I-cell disease) fail to phosphorylate the mannose residues required for targeting to lysosomes (see Chapter 23). As a result, the lysosomal enzymes are secreted from the cell, and lysosomes fail to degrade waste materials. Lysosomes become engorged with undigested substrates, leading to fatal cell and tissue abnormalities.
Proteolytic Processing of Protein Precursors
A number of proteins, particularly peptide hormones, are cleaved into active fragments in the Golgi apparatus and its secretory vesicles. Such proteins are synthesized as large precursors with one or more small hormones embedded in long polypeptides. One example is a yeast mating pheromone. Another is pro-opiomelanocortin, the precursor to no less than six small peptide hormones. Proteolytic enzymes called prohormone convertases cleave the precursor proteins into active hormones in the TGN and post-TGN transport intermediates. The mixture of products depends on the prohormone convertases expressed in particular cells. Proteolysis in the Golgi also affects the final folding state and activity of many other proteins. Inherited defects in these processing pathways lead to a number of diseases, including hormone insufficiency and a hereditary amyloid disease.
Lipid Biosynthesis and Metabolism
Another Golgi-specific processing activity is the synthesis of sphingolipids. Sphingolipids, including sphingomyelin (SM) and the glycosphingolipids glucosylceramide and galactosylceramide, play central roles in membrane sorting within the Golgi apparatus as well as sorting within post-Golgi compartments. As was described earlier, these lipids have affinity for cholesterol, act as donors of intermolecular hydrogen bonds, and have saturated lipid chains, resulting in the denser packing of these lipids compared to glycerophospholipids. When densely packed, the sphingolipids and cholesterol form long cylinders that cause an increase in the thickness of the bilayer relative to a bilayer containing glycerophospholipids alone (e.g., ER [Fig. 21-3]). An enrichment of sphingolipids and cholesterol along the secretory pathway from Golgi to plasma membrane results in an increased thickness of the membranes along this route relative to the ER. This plays a vital role within the secretory system because of a protein’s tendency to match the length of its transmembrane domain with that of the lipid bilayer.
The backbone of all sphingolipids, ceramide (see Chapter 20), is synthesized in the ER and then transported to the Golgi complex, where it is modified to form glucosylceramide and sphingomyelin. Glucosylceramide synthesis is catalyzed on the cytoplasmic surface of Golgi membranes by the enzyme UDP-glucose:ceramide glucosyltransferase. Glucosylceramide can then be transported to the plasma membrane or translocated to the luminal leaflet of Golgi membranes, where galactosylation of the head group results in the formation of lactosylceramide. Sequential glycosylation of lactosylceramide by glycosyltransferases of the Golgi lumen generates complex glycolipids and gangliosides for the plasma membrane.
Sphingomyelin synthesis is catalyzed by sphingomyelin-synthase, an enzyme on the luminal leaflet of Golgi membranes. The enzyme transfers phosphorylcholine from phosphatidylcholine to ceramide, releasing the signaling lipid diacylglycerol (DAG) in the process. This mechanism therefore couples consumption of the signaling lipid ceramide (see Fig. 26-11) with the production of the signaling lipid DAG (see Fig. 26-8). If DAG accumulates in the Golgi apparatus, phosphorylcholine can be transferred from sphingomyelin back to DAG, forming phosphatidylcholine and ceramide. Alternatively, DAG can be digested by lipases.
Glycosphingolipid microdomains, or rafts, are thought to form spontaneously by lateral lipid-lipid associations in the luminal leaflet of Golgi membranes. GPI-anchored proteins (see Fig. 7-7), doubly acylated proteins, and many transmembrane proteins physically partition into these microdomains and are thereby enriched in lipid structures that sort preferentially to the plasma membrane. The ability of the Golgi apparatus to drive glycosphingolipid synthesis therefore contributes to its function as a sorting station.
Altan-Bonnet N, Sougrat R, Lippincott-Schwartz J. Molecular basis for Golgi maintenance and biogenesis. Curr Opin Cell Biol. 2004;16(4):364-372.
Antonny B. Membrane deformation by protein coats. Curr Opin Cell Biol. 2006;18:1-9.
Bankaitis VA, Morris AJ. Lipids and the exocytic machinery of eukaryotic cells. Curr Opin Cell Biol. 2003;15:389-395.
Barlowe C. Signals for COPII-dependent export from the ER: What’s the ticket out? Trends Cell Biol. 2003;13:295-300.
Baumgart T, Hess ST, Webb WW. Imaging coexisting fluid domains in biomembrane models coupling curvature and line tension. Nature. 2003;425:821-824.
Behnia R, Munro S. Organelle identity and the signposts for membrane traffic. Nature. 2005;438:597-604.
Bi X, Corpina RA, Goldberg J. Structure of the Sec23/24-Sar1 pre-budding complex of the COPII vesicle coat. Nature. 2002;419:271-277.
Bonifacino JS, Glick BS. The mechanisms of vesicle budding and fusion. Cell. 2004;116:153-166.
Bonifacino JS, Lippincott-Schwartz J. Coat proteins: Shaping membrane transport. Nat Rev Mol Cell Biol. 2003;4:409-414.
Bretscher MS, Munro S. Cholesterol and the Golgi apparatus. Science. 1993;261:1280-1281.
Godi A, DiCampli A, Konstantakopoulos A, et al. FAPPs control Golgi-to-cell-surface membrane traffic by binding to Arf and PtdIns(4)P. Nat Cell Biol. 2004;6:393-404.
Gurkan C, Stagg SM, Lapointe P, Balch WE. The COPII cage: Unifying principles of vesicle coat assembly. Nature Rev Mol Cell BIol. 2006;7:727-738.
Holthius JCM, Pomorski T, Raggers RJ, et al. The organizing potential of sphingolipids in intracellular membrane transport. Physiol Rev. 2001;81:1689-1723.
Keller P, Simons K. Post-Golgi biosynthetic trafficking. J Cell Science. 1997;110:3001-3009.
Kepes F, Rambourg A, Satiat-Jeunemaitre B. Morphodynamics of the secretory pathway. Int Rev Cytol. 2005;242:55-120.
Killian JA. Hydrophobic mismatch between proteins and lipids in membranes. Biochim Biophys Acta. 1998;1376:401-416.
Lee MCS, Miller EA, Goldberg J, et al. Bi-directional protein transport between the ER and Golgi. Annu Rev Cell Dev Biol. 2004;20:87-123.
Lippincott-Schwartz J, Roberts TH, Hirschberg K. Secretory protein trafficking and organelle dynamics in living cells. Annu Rev Cell Dev Biol. 2000;16:557-589.
Miller EA, Beilharz TH, Malkus PN, et al. Multiple cargo binding sites on the COPII subunit Sec24p ensure capture of diverse membrane proteins into transport vesicles. Cell. 2003;114:497-509.
Nishimura N, Balch WE. A di-acidic signal required for selective export from the endoplasmic reticulum. Science. 1997;277:556-558.
Palmer KJ, Stephens DJ. Biogenesis of ER-to-Golgi transport carriers: Complex roles of COPII in ER export. Trends Cell Biol. 2004;14:57-61.
Renault L, Gulbert B, Cherfils J. Structural snapshots of the mechanism and inhibition of a guanine nucleotide exchange factor. Nature. 2003;426:525-530.
Van Meer G, Sprong H. Membrane lipids and vesicular traffic. Curr Opin Cell Biol. 2004;16:373-378.
Varki A. Factors controlling the glycosylation potential of the Golgi apparatus. Trends Cell Biol. 1998;8:34-40.
Whyte JRC, Munro S. Vesicle tethering complexes in membrane traffic. J Cell Science. 2002;115:2627-2637.