Chapter 95 Respiratory Tract Disorders
Respiratory disorders are the most frequent cause of admission for neonatal intensive care in both term and preterm infants. Signs and symptoms of respiratory distress include cyanosis, grunting, nasal flaring, retractions, tachypnea, decreased breath sounds with or without rales and/or rhonchi, and pallor. A wide variety of pathologic lesions may be responsible for respiratory disturbances, including pulmonary, airway, cardiovascular, central nervous, and other disorders (Fig. 95-1).
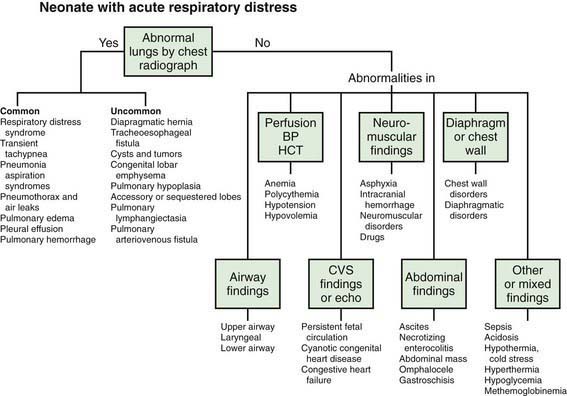
Figure 95-1 Neonate with acute respiratory distress. BP, blood pressure; CVS, cardiovascular system; HCT, hematocrit.
(From Battista MA, Carlo WA: Differential diagnosis of acute respiratory distress in the neonate. In Frantz ID, editor: Tufts University of School of Medicine and Floating Hospital for Children reports on neonatal respiratory diseases, vol 2, issue 3, Newtown, PA, 1992, Associates in Medical Marketing Co.)
95.1 Transition to Pulmonary Respiration
Successful establishment of adequate lung function at birth depends on airway patency, functional lung development, and maturity of respiratory control. Fetal lung fluid must be removed and replaced with gas. This process begins before birth as active sodium transport across the pulmonary epithelium drives liquid from the lung lumen into the interstitium with subsequent absorption into the vasculature. Increased levels of circulating catecholamines, vasopressin, prolactin, and glucocorticoids enhance lung fluid adsorption and trigger the change in lung epithelia from a chloride-secretory to a sodium-reabsorptive mode. Functional residual capacity (FRC) must be established and maintained in order to develop a ventilation-perfusion relationship that will provide optimal exchange of oxygen and carbon dioxide between alveoli and blood (Chapter 415).
95.2 Apnea
Apnea is a common problem in preterm infants that may be due to prematurity or an associated illness. In term infants, apnea is always worrisome and demands immediate diagnostic evaluation. Periodic breathing must be distinguished from prolonged apneic pauses, because the latter may be associated with serious illnesses. Apnea is a feature of many primary diseases that affect neonates (Table 95-1). These disorders produce apnea by direct depression of the central nervous system’s control of respiration (hypoglycemia, meningitis, drugs, hemorrhage, seizures), disturbances in oxygen delivery (shock, sepsis, anemia), or ventilation defects (obstruction of the airway, pneumonia, muscle weakness).
Table 95-1 POTENTIAL CAUSES OF NEONATAL APNEA AND BRADYCARDIA
Central nervous system | Intraventricular hemorrhage, drugs, seizures, hypoxic injury, herniation, neuromuscular disorders, Leigh syndrome, brainstem infarction or anomalies (e.g., olivopontocerebellar atrophy), after general anesthesia |
Respiratory | Pneumonia, obstructive airway lesions, upper airway collapse, atelectasis, extreme prematurity, laryngeal reflex, phrenic nerve paralysis, pneumothorax, hypoxia |
Infectious | Sepsis, meningitis (bacterial, fungal, viral), respiratory syncytial virus, pertussis |
Gastrointestinal | Oral feeding, bowel movement, necrotizing enterocolitis, intestinal perforation |
Metabolic | ↓ Glucose, ↓ calcium, ↓/↑ sodium, ↑ ammonia, ↑ organic acids, ↑ ambient temperature, hypothermia |
Cardiovascular | Hypotension, hypertension, heart failure, anemia, hypovolemia, vagal tone |
Other | Immaturity of respiratory center, sleep state |
Arad-Cohen N, Cohen A, Tirosh E. The relationship between gastroesophageal reflux and apnea in infants. J Pediatr. 2000;137:321-326.
Committee on Fetus and Newborn, American Academy of Pediatrics. Apnea, sudden infant death syndrome, and home monitoring. Pediatrics. 2003;111:914-917.
Kimball AL, Carlton DP. Gastroesophageal reflux medications in the treatment of apnea in premature infants. J Pediatr. 2001;138:355-360.
Litmanovitz I, Carlo WA. Expectant management of pneumothorax in ventilated neonates. Pediatrics. 2008;122:e975-e979.
Martin RJ, Abu-Shaweesh JM, Baird TM. Apnoea of prematurity. Paediatr Respir Rev. 2004;5:S377-S382.
Ramanathan R, Corwin MJ, Hunt CE, et al. Cardiorespiratory events recorded on home monitors: comparison of healthy infants with those at increased risk for SIDS. JAMA. 2001;285:2199-2207.
Schmidt B, Roberts RS, Davis P, et al. Long term effects of caffeine for apnea of prematurity. N Engl J Med. 357, 2007. 1983–1902
Schmidt B, Roberts RS, Davis P, et al. Caffeine therapy for apnea of prematurity. N Engl J Med. 2006;354:2112-2120.
Sreenan C, Lemke RP, Hudson-Mason A, et al. High-flow nasal cannulae in the management of apnea of prematurity: a comparison with conventional nasal continuous positive airway pressure. Pediatrics. 2001;107:1081-1083.
Steer P, Flenady V, Shearman A, et al. High dose caffeine citrate for extubation of preterm infants: a randomized controlled trial. Arch Dis Child Fetal Neonatal Ed. 2004;89:F499-F503.
Tauman R, Sivan Y. Duration of home monitoring for infants discharged with apnea of prematurity. Biol Neonate. 2000;78:168-173.
95.3 Respiratory Distress Syndrome (Hyaline Membrane Disease)
Etiology and Pathophysiology
Surfactant deficiency (decreased production and secretion) is the primary cause of RDS. The failure to attain an adequate FRC and the tendency of affected lungs to become atelectatic correlate with high surface tension and the absence of pulmonary surfactant. The major constituents of surfactant are dipalmitoyl phosphatidylcholine (lecithin), phosphatidylglycerol, apoproteins (surfactant proteins SP-A, SP-B, SP-C, and SP-D), and cholesterol (Fig. 95-2). With advancing gestational age, increasing amounts of phospholipids are synthesized and stored in type II alveolar cells (Fig. 95-3). These surface-active agents are released into the alveoli, where they reduce surface tension and help maintain alveolar stability by preventing the collapse of small air spaces at end-expiration. Because of immaturity, the amounts produced or released may be insufficient to meet postnatal demands. Surfactant is present in high concentrations in fetal lung homogenates by 20 wk of gestation, but it does not reach the surface of the lungs until later. It appears in amniotic fluid between 28 and 32 wk. Mature levels of pulmonary surfactant are present usually after 35 wk. Though rare, genetic disorders may contribute to respiratory distress. Abnormalities in surfactant protein B and C genes as well as a gene responsible for transporting surfactant across membranes (ABC transporter 3 [ABCA3]) are associated with severe and often lethal familial respiratory disease. Other familial causes of neonatal respiratory distress (not RDS) include alveolar capillary dysplasia, acinar dysplasia, pulmonary lymphangiectasia, and mucopolysaccharidosis.
(Courtesy of Mary Williams, MD, University of California, San Francisco.)
Deficient synthesis or release of surfactant, together with small respiratory units and a compliant chest wall, produces atelectasis and results in perfused but not ventilated alveoli, causing hypoxia. Decreased lung compliance, small tidal volumes, increased physiologic dead space, and insufficient alveolar ventilation eventually result in hypercapnia. The combination of hypercapnia, hypoxia, and acidosis produces pulmonary arterial vasoconstriction with increased right-to-left shunting through the foramen ovale and ductus arteriosus and within the lung itself. Pulmonary blood flow is reduced and ischemic injury both to the cells producing surfactant and to the vascular bed results in an effusion of proteinaceous material into the alveolar spaces (Fig. 95-4).
Diagnosis
The clinical course, chest radiographic findings, and blood gas and acid-base values help establish the clinical diagnosis. On radiographs, the lungs may have a characteristic but not pathognomonic appearance that includes a fine reticular granularity of the parenchyma and air bronchograms, which are often more prominent early in the left lower lobe because of superimposition of the cardiac shadow (Fig. 95-5). The initial radiographic appearance is occasionally normal, with the typical pattern developing at 6-12 hr. Considerable variation in film findings may be seen, depending on the phase of respiration (inspiratory vs expiratory radiograph) and the use of CPAP or positive end-expiratory pressure (PEEP); this variation often results in poor correlation between radiographic findings and the clinical course. Laboratory findings are characterized initially by hypoxemia and later by progressive hypoxemia, hypercapnia, and variable metabolic acidosis.
In the differential diagnosis, early-onset sepsis may be indistinguishable from RDS. In pneumonia manifested at birth, the chest roentgenogram may be identical to that for RDS. Maternal group B streptococcal colonization, identification of organisms on gram staining of gastric or tracheal aspirates or a buffy coat smear, and/or the presence of marked neutropenia may suggest the diagnosis of early-onset sepsis. Cyanotic heart disease (total anomalous pulmonary venous return) can also mimic RDS both clinically and radiographically. Echocardiography with color-flow imaging should be performed in infants who show no response to surfactant replacement, to rule out cyanotic congenital heart disease as well as ascertain patency of the ductus arteriosus and assess pulmonary vascular resistance (PVR). Persistent pulmonary hypertension, aspiration (meconium, amniotic fluid) syndromes, spontaneous pneumothorax, pleural effusions, and congenital anomalies such as cystic adenomatoid malformation, pulmonary lymphangiectasia, diaphragmatic hernia, and lobar emphysema must be considered in patients with an atypical clinical course but can generally be differentiated from RDS through radiographic evaluation. Transient tachypnea may be distinguished by its short and mild clinical course and is characterized by low or no need for oxygen supplementation. Congenital alveolar proteinosis (congenital surfactant protein B deficiency) is a rare familial disease that manifests as severe and lethal RDS in predominantly term and near-term infants (Chapter 399). In atypical cases of RDS, a lung profile (lecithin:sphingomyelin ratio and phosphatidylglycerol determination) performed on a tracheal aspirate can be helpful in establishing a diagnosis of surfactant deficiency.
Prevention
Avoidance of unnecessary or poorly timed cesarean section, appropriate management of high-risk pregnancy and labor, and prediction of pulmonary immaturity with possible in utero acceleration of maturation (Chapter 90) are important preventive strategies. In timing of cesarean section or induction of labor, estimation of fetal head circumference by ultrasonography and determination of the lecithin concentration in amniotic fluid by the lecithin:sphingomyelin ratio (particularly useful with phosphatidylglycerol measurement in diabetic pregnancies) decrease the likelihood of delivering a premature infant. Antenatal and intrapartum fetal monitoring may similarly decrease the risk of fetal asphyxia; asphyxia is associated with an increased incidence and severity of RDS.
Treatment
The basic defect requiring treatment in RDS is inadequate pulmonary exchange of oxygen and carbon dioxide; metabolic acidosis and circulatory insufficiency are secondary manifestations. Early supportive care of premature infants, especially in the treatment of acidosis, hypoxia, hypotension (Chapter 92), and hypothermia, may lessen the severity of RDS. Therapy requires careful and frequent monitoring of heart and respiratory rates, oxygen saturation, PaO2, PaCO2, pH, serum bicarbonate, electrolytes, glucose, and hematocrit, blood pressure, and temperature. Arterial catheterization is frequently necessary. Because most cases of RDS are self-limited, the goal of treatment is to minimize abnormal physiologic variations and superimposed iatrogenic problems. Treatment of infants with RDS is best carried out in the NICU.
The general principles for supportive care of any premature infant should be adhered to, including developmental care and scheduled “touch times.” To avoid hypothermia and minimize oxygen consumption, the infant should be placed in an incubator or radiant warmer, and core temperature maintained between 36.5 and 37°C (Chapters 91 and 92). Use of an incubator is preferable in very LBW (VLBW) infants owing to the high insensible water losses associated with radiant heat. Calories and fluids should initially be provided intravenously. For the 1st 24 hr, 10% glucose and water should be infused through a peripheral vein at a rate of 65-75 mL/kg/24 hr. Electrolytes should be added on day 2 in the most mature infants and on days 3 to 7 in the more immature ones. Fluid volume is increased gradually over the 1st week. Excessive fluids (> 140 mL/kg/day) contribute to the development of patent ductus arteriosus (PDA) and BPD.
Infants with respiratory failure or persistent apnea require assisted mechanical ventilation. Reasonable measures of respiratory failure are: (1) arterial blood pH <7.20, (2) arterial blood PCO2 of 60 mmHg or higher, and (3) oxygen saturation <85% at oxygen concentrations of 40-70% and CPAP of 5-10 cm H2O. Infants with persistent apnea also need mechanical ventilation. Intermittent positive pressure ventilation delivered by time-cycled, pressure-limited, continuous flow ventilators is a common method of conventional ventilation for newborns. Other methods of conventional ventilation are synchronized intermittent mandatory ventilation (the set rate and pressure synchronized with the patient’s own breaths), pressure support (the patient triggers each breath and a set pressure is delivered), and volume ventilation (a mode in which a specific tidal volume is set and the delivered pressure varies), and combinations thereof. Assisted ventilation for infants with RDS should always include PEEP (Chapter 65.1). High ventilatory rates (60/min) result in fewer air leaks. With use of high ventilatory rates, sufficient expiratory time should be allowed to avoid the administration of inadvertent PEEP.
Surfactant deficiency is the primary pathophysiology of RDS. Immediate effects of surfactant replacement therapy include improved alveolar-arterial oxygen gradients, reduced ventilatory support, increased pulmonary compliance, and improved chest radiograph appearance . Treatment is initiated as soon as possible in the hours after birth. Repeated dosing is given via the endotracheal tube every 6-12 hr for a total of 2 to 4 doses, depending on the preparation. Exogenous surfactant should be given by a physician who is qualified in neonatal resuscitation and respiratory management and who is able to care for the infant beyond the 1st hr of stabilization. Additional on-site staff support required includes nurses and respiratory therapists experienced in the ventilatory management of premature infants. Appropriate monitoring equipment (radiology, blood gas laboratory, pulse oximetry) must also be available. Complications of surfactant therapy include transient hypoxia, hypercapnia, bradycardia and hypotension, blockage of the endotracheal tube, and pulmonary hemorrhage (Chapter 95.13).
Pharmacologic Therapies
Metabolic acidosis in RDS may be a result of perinatal asphyxia and hypotension and is often encountered when an infant has required resuscitation (Chapter 94). Sodium bicarbonate, 1-2 mEq/kg, may be administered over 15-20 min through a peripheral or umbilical vein, followed by an acid-base determination within 30 min, or it may be administered over several hours. Often, sodium bicarbonate is administered on an emergency basis through an umbilical venous catheter. Alkali therapy may result in skin slough from infiltration, increased serum osmolarity, hypernatremia, hypocalcemia, hypokalemia, and liver injury when concentrated solutions are administered rapidly through an umbilical vein catheter wedged in the liver.
Monitoring of aortic blood pressure through an umbilical or peripheral arterial catheter or by oscillometric technique is useful in managing the shock-like state that may occur during the 1st hr or so in premature infants who have been asphyxiated or have severe RDS (see Fig. 94-2). The position of a radiopaque umbilical catheter should be checked roentgenographically after insertion (see Fig. 95-5). The tip of an umbilical artery catheter should lie just above the bifurcation of the aorta (L3-L5) or above the celiac axis (T6-T10). Preferred sites for peripheral catheters are the radial or posterior tibial arteries. The placement and supervision should be carried out by skilled and experienced personnel. Catheters should be removed as soon as patients no longer have any indication for their continued use—usually when an infant is stable and the FIO2 is < 40%. Hypotension and low flow in the superior vena cava (SVC) have been associated with higher rates of CNS morbidity and mortality and should be treated with cautious administration of volume (crystalloid) and early use of vasopressors. Dopamine is more effective in raising blood pressure than dobutamine. Hypotension may be refractory to pressors, but responsive to glucocorticoids, especially in neonates < 1,000 g. This hypotension may be due to transient adrenal insufficiency in the ill premature infant. It should be treated with intravenous hydrocortisone (Solu-Cortef) at 1-2 mg/kg/dose q6-12 hr (Chapter 92).
Because of the difficulty of distinguishing group B streptococcal or other bacterial infections from RDS, empirical antibiotic therapy is indicated until the results of blood cultures are available. Penicillin or ampicillin with an aminoglycoside is suggested, although the choice of antibiotics should be based on the recent pattern of bacterial sensitivity in the hospital where the infant is being treated (Chapter 103).
Complications of Respiratory Distress Syndrome and Intensive Care
Air leaks are a common complication of the management of infants with RDS (Chapter 95.12).
Instead of showing improvement on the 3rd or 4th day, which would be consistent with the natural course of RDS, some infants demonstrate an increased need for oxygen and ventilatory support. Respiratory distress persists or worsens and is characterized by hypoxia, hypercapnia, oxygen dependence, and, in severe cases, the development of right-sided heart failure. The chest roentgenogram may reveal pulmonary interstitial emphysema, wandering atelectasis with concomitant hyperinflation, and cyst formation (Fig. 95-6). Four distinct pathologic stages of classic BPD have been identified: acute lung injury, exudative bronchiolitis, proliferative bronchiolitis, and obliterative fibroproliferative bronchiolitis. Histologic study at this stage (10-20 days) shows residual hyaline membrane formation, progressive alveolar coalescence with atelectasis of the surrounding alveoli, interstitial edema, coarse focal thickening of the basement membrane, and widespread bronchial and bronchiolar mucosal metaplasia and hyperplasia. These findings correspond to a severe maldistribution of ventilation. Pathologic examination of infants who die later in the course of BPD reveals cardiac enlargement and pulmonary changes consisting of focal areas of emphysema with hypertrophy of the peribronchial smooth muscle of the tributary bronchioles, perimucosal fibrosis, widespread metaplasia of the bronchiolar mucosa, thickening of basement membranes, and separation of the capillaries from the alveolar epithelial cells.
BPD can be classified according to the need for oxygen supplementation (Table 95-2). Neonates receiving positive pressure support or ≥30% supplemental oxygen at 36 wk or at discharge (whichever occurs 1st) are diagnosed as having severe BPD. Those needing supplementation with 22-29% oxygen at this age are diagnosed as having moderate BPD. Those who need oxygen supplementation for >28 days but are breathing room air at 36 wk or at discharge are diagnosed as having mild BPD. Those receiving <30% oxygen should undergo a stepwise 2% reduction in supplemental oxygen to room air while under continuous observation and with oxygen saturation monitoring to determine whether they can be weaned off oxygen. This test is highly reliable and correlated with discharge home on oxygen, length of hospital stay, and hospital readmissions in the 1st yr of life.
Table 95-2 DEFINITION OF BRONCHOPULMONARY DYSPLASIA: DIAGNOSTIC CRITERIA*
GESTATIONAL AGE | ||
---|---|---|
<32 Wk | ≥32 Wk | |
Time point of assessment |
BPD, bronchopulmonary dysplasia; NCPAP, nasal continuous positive airway pressure; PPV, positive pressure ventilation.
* BPD usually develops in neonates being treated with oxygen and PPV for respiratory failure, most commonly respiratory distress syndrome. Persistence of the clinical features of respiratory disease (tachypnea, retractions, crackles) is considered common to the broad description of BPD and has not been included in the diagnostic criteria describing the severity of BPD. Infants treated with > 21% oxygen and/or PPV for nonrespiratory disease (e.g., central apnea or diaphragmatic paralysis) do not have BPD unless parenchymal lung disease also develops and they have clinical features of respiratory distress. A day of treatment with > 21% oxygen means that the infant received > 21% oxygen for more than 12 hr on that day. Treatment with > 21% oxygen and/or PPV at 36 wk postmenstrual age or at 56 days postnatal age or discharge should not reflect an “acute” event, but should rather reflect the infant’s usual daily therapy for several days preceding and after 36 wk postmenstrual age, 56 days postnatal age, or discharge.
† A physiologic test confirming that the oxygen requirement at the assessment time point remains to be defined. This assessment may include a pulse oximetry saturation range.
From Jobe AH, Bancalari E: Bronchopulmonary dysplasia, Am J Respir Crit Care Med 163:1723–1729, 2001.
American Academy of Pediatrics Committee on Fetus and Newborn. Policy statement—postnatal corticosteroids to prevent or great bronchopulmonary dysplasia. Pediatrics. 2010;126(4):800-808.
Anand KJ, Hall RW, Desai N, et al. Effects of morphine analgesia in ventilated preterm neonates: primary outcomes from the NEOPAIN randomised trial. NEOPAIN Trial Investigators Group. Lancet. 2004;363:1673-1682.
Auten RL, Davis JM. The role of oxygen in health and disease—a series of reviews. Pediatr Res. 2009;66:121-127.
Batton B, Zhu X, Fanaroff J, et al. Blood pressure, anti-hypotensive therapy, and neurodevelopment in extremely preterm infants. J Pediatr. 2009;154:351-357.
Been JV, Kornelisse RF, Rours IG, et al. Early postnatal blood pressure in preterm infants: effects of chorioamnionitis and timing of antenatal steroids. Pediatr Res. 2009;66:571-576.
Benders MJNL, Groenendaal F, Van Bel F, et al. Brain development of the preterm neonate after neonatal hydrocortisone treatment for chronic lung disease. Pediatr Res. 2009;66:555-559.
Bhandari A, Bhandari V. Pitfalls, problems, and progress in bronchopulmonary dysplasia. Pediatrics. 2009;123:1562-1573.
Bhandari V, Zhou G, Bizzarro MJ, et al. Genetic contribution to patent ductus arteriosus in the premature newborn. Pediatrics. 2009;123:669-673.
Bidegain M, Greenberg R, Simmons C, et al. Vasopressin for refractory hypotension in extremely low birth weight infants. J Pediatr. 2010;157:502-504.
Brion LP, Primhak RA: Intravenous or enteral loop diuretics for preterm infants with (or developing) chronic lung disease, Cochrane Database Syst Rev (4):CD001453, 2000.
Brooks JM, Travadi JN, Patole SK, et al. Is surgical ligation of patent ductus arteriosus necessary? The Western Australian experience of conservative management. Arch Dis Child. 2005;90:F235-F239.
Brownfoot FC, Crowther CA, Middleton P: Different corticosteroids and regimens for accelerating fetal lung maturation for women at risk of preterm birth, Cochrane Database Syst Rev (4):CD006764, 2008.
Carlo WA, Stark AR, Wright LL, et al. Minimal ventilation to prevent bronchopulmonary dysplasia in extremely-low-birth-weight infants. J Pediatr. 2002;141:370-374.
Castillo A, Sola A, Baquero H, et al. Pulse oxygen saturation levels and arterial oxygen tension values in newborns receiving oxygen therapy in the neonatal intensive care unit: is 85% to 93% an acceptable range? Pediatrics. 2008;121:882-889.
Claure N, D’Ugard C, Bancalari E. Automated adjustment of inspired oxygen in preterm infants with frequent fluctuations in oxygenation: a pilot clinical trial. J Pediatr. 2009;155:640-655.
Clyman RI, Chome N. Patent ductus arteriosus: evidence for and against treatment. J Pediatr. 2007;150:216-219.
Cole FS, Alleyne C, Barks JDE, et al. NIH consensus development conference statement: inhaled nitric-oxide therapy for premature infants. Pediatrics. 2011;127(2):363-369.
Crowther CA, Haslam RR, Hiller JE, et al. Neonatal respiratory distress syndrome after repeat exposure to antenatal corticosteroids: a randomised controlled trial. Lancet. 2006;367:1913-1919.
Czernik C, Lemmer J, Metze B, et al. B-type natriuretic peptide to predict ductus intervention in infants < 28 weeks. Pediatr Res. 2008;64:286-290.
Darlow BA, Graham PJ: Vitamin A supplementation to prevent mortality and short and long-term morbidity in low birthweight infants, Cochrane Database Syst Rev (4):CD000501, 2007.
Davis PG, Henderson-Smart DJ: Nasal continuous positive airway pressure immediately after extubation for preventing morbidity in preterm infants. Cochrane Database Syst Rev (2):CD000143, 2003.
DePaoli AG, Davis PG, Faber B, et al: Devices and pressure sources for administration of nasal continuous positive airway pressure (NCPAP) in preterm neonates, Cochrane Database Syst Rev (1):CD002977, 2008.
El-Khuffash AF, Molloy EJ. Influence of a patent ductus arteriosus on cardiac troponin T levels in preterm infants. J Pediatr. 2008;153:350-353.
Engle WA. Committee on Fetus and Newborn: Surfactant-replacement therapy for respiratory distress in the preterm and term neonate. Pediatrics. 2008;121:419-432.
Finer N, Leone T. Oxygen saturation monitoring for the preterm infant: the evidence basis for current practice. Pediatr Res. 2009;65:375-380.
Finer N, Mannino FL. High-flow nasal cannula: a kinder, gentler CPAP? J Pediatr. 2009;154:160-162.
Greenough A, Dimitriou G, Prendergast M: Synchronized mechanical ventilation for respiratory support in newborn infants, Cochrane Database Syst Rev (1):CD000456, 2008.
Guay J. Multiple courses of antenatal corticosteroids. Lancet. 2008;372:2094-2095.
Halliday HL, Ehrenkranz RA, Doyle LW: Moderately early (7–14 days) postnatal corticosteroids for preventing chronic lung disease in preterm infants, Cochrane Database Syst Rev (1):CD001144, 2003.
Hammerman C, Shchors I, Jacobson S, et al. Ibuprofen versus continuous indomethacin in premature neonates with patent ductus arteriosus: is the difference in the mode of administration? Pediatr Res. 2008;64:291-297.
Henderson-Smart DJ, Davis PG: Prophylactic methylxanthines for extubation in preterm infants, Cochrane Database of Systematic Reviews (1):CD000139, 2003.
Hibbs AM, Walsch MC, Martin RJ, et al. One-year respiratory outcomes of preterm infants enrolled in the nitric oxide (to prevent) chronic lung disease trial. J Pediatr. 2008;153:525-529.
Higgins RD, Bancalari E, Willinger M, et al. Executive summary of the workshop on oxygen in neonatal therapies: controversies and opportunities for research. Pediatrics. 2007;119:790-796.
Hosono S, Mugishima H, Fujita H, et al. Blood pressure and urine output during the first 120 h of life in infants born at less than 29 weeks’ gestation related to umbilical cord milking. Arch Dis Child Feral Neonatal Ed. 2009;94:F328-F331.
Jegatheesan P, Ianus V, Buchh B, et al. Increased indomethacin dosing for persistent patent ductus arteriosus in preterm infants: a multicenter, randomized, controlled trial. J Pediatr. 2008;153:183-189.
Jobe AH, Kallapur SG. Chorioamnionitis, surfactant, and lung disease in very low birth weight infants. J Pediatr. 2010;56:3-4.
Kamlin COF, Davis PG: Long versus short inspiratory times in neonates receiving mechanical ventilation, Cochrane Database Syst Rev (4):CD004503, 2003.
Khemani E, McElhinney DB, Rhein L, et al. Pulmonary artery hypertension in formerly premature infants with bronchopulmonary dysplasia: clinical feature and outcomes in the surfactant era. Pediatrics. 2007;120:1260-1269.
Kinsella JP, Cutter GR, Walsh WF, et al. Early inhaled nitric oxide therapy in premature newborns with respiratory failure. N Engl J Med. 2006;355:354-364.
Kugelman A, Feferkorn I, Riskin A, et al. Nasal intermittent mandatory ventilation versus nasal continuous positive airway pressure for respiratory distress syndrome: a randomized, controlled, prospective study. J Pediatr. 2007;150:521-526.
Kwinta P, Bik-Multanowski M, Mitkowska Z, et al. Genetic risk factors of bronchopulmonary dysplasia. Pediatr Res. 2008;64:682-688.
Lago P, Bettiol T, Salvadori S, et al. Safety and efficacy of ibuprofen versus indomethacin in preterm infants treated for patent ductus arteriosus: a randomized controlled trial. Eur J Pediatr. 2002;161:202-207.
Laughon M, Bose C, Allred E, et al. Factors associated with treatment for hypotension in extremely low gestational age newborns during the first postnatal week. Pediatrics. 2007;119:273-280.
Lavoie PM, Pham C, Jang KL. Heritability of bronchopulmonary dysplasia, defined according to the consensus statement of the National Institutes of Health. Pediatrics. 2008;122:479-485.
Lee BH, Stoll BJ, McDonald SA, et al. Adverse neonatal outcomes associated with antenatal dexamethasone versus antenatal betamethasone. Pediatrics. 2006;117:1503-1510.
Levit O, Jiang Y, Bizzarro MJ, et al. The genetic susceptibility to respiratory distress syndrome. Pediatr Res. 2009;66:693-697.
McCallion N, Davis PG, Morley CJ: Volume-targeted versus pressure-limited ventilation in the neonate, Cochrane Database Syst Rev (3):CD003666, 2005.
Meneses J, Bhandari V, Alves JG, et al. Noninvasive ventilation for respiratory distress syndrome: a randomized controlled trial. Pediatrics. 2011;127:300-307.
Mercier JC, Hummler H, Durrmeyer X, et al. Inhaled nitric oxide for prevention of brobchopulmonary dysplasia in premature babies (EUNO): a randomized controlled trial. Lancet. 2010;376:346-352.
Mestan KKL, Marks JD, Hecox K, et al. Neurodevelopmental outcomes of premature infants treated with inhaled nitric oxide. N Engl J Med. 2005;353:23-32.
Miller SP, Mayer EE, Clyman RI, et al. Prolonged indomethacin exposure is associated with decreased white matter injury detected with magnetic resonance imaging in premature newborns at 24 to 28 weeks’ gestation at birth. Pediatrics. 2006;117:1626-1631.
Montan S, Arul Kumaran S. Neonatal respiratory distress syndrome. Lancet. 2006;367:1878-1879.
Morley CJ, Davis PG, Doyle LW, et al. Nasal CPAP or intubation at birth for very preterm infants. N Engl J Med. 2008;358:700-708.
Moya FR, Sinha SS, Segal RS, et al. Comparison of incidences of all-cause mortality between the novel surfactant, Surfaxin (lucinactant) and the animal derived surfactants Survanta (beractant) and Curosurf (poractant alfa). Pediatr Res. 2004;56:497.
Ng PC, Lee CH, Bnur FL, et al. A double-blind, randomized, controlled study of a “stress dose” of hydrocortisone for rescue treatment of refractory hypotension in preterm infants. Pediatrics. 2006;117:367-375.
Nori S, Seri I. Treatment of the patent ductus arteriosus: when, how, and for how long? J Pediatr. 2009;155:774-776.
Parikh NA, Lasky RE, Kennedy KA, et al. Postnatal dexamethasone therapy and cerebral tissue volumes in extremely low birth weight infants. Pediatrics. 2007;119:265-272.
Pfister RH, Soll R, Wiswell TE: Protein containing synthetic surfactant versus animal derived surfactant extract for the prevention and treatment of respiratory distress syndrome, Cochrane Database Syst Rev (4):CD003311, 2007.
Polin RA. Bubble CPAP: a clash of science, culture, and religion. J Pediatr. 2009;154:633-634.
Richards J, Johnson A, Fox G, et al. A second course of ibuprofen is effective in the closure of a clinically significant PDA in ELBW infants. Pediatrics. 2009;124:e287-e293.
Roberts D, Dalziel S: Antenatal corticosteroids for accelerating fetal lung maturation for women at risk of preterm birth, Cochrane Database Syst Rev (3):CD004454, 2006.
Rojas MA, Lozano JM, Rojas MX, et al. Very early surfactant without mandatory ventilation in premature infants treated with early continuous positive airway pressure: a randomized controlled trial. Pediatrics. 2009;123:137-142.
Schmölzer GM, Te Pas AB, Davis PG, et al. Reducing lung injury during neonatal resuscitation of preterm infants. J Pediatr. 2008;153:741-745.
Seger N, Soll R: Animal derived surfactant extract for treatment of respiratory distress syndrome, Cochrane Database Syst Rev (2):CD007836, 2009.
Seri N. Hydrocortisone and vasopressor-resistant shock in preterm neonates. Pediatrics. 2006;117:516-518.
Shah PS, Shah VS: Continuous heparin infusion to prevent thrombosis and catheter occlusion in neonates with peripherally placed percutaneous central venous catheters, Cochrane Database Syst Rev (2):CD002772, 2008.
Shah SSS, Ohlsson A, Halliday H, et al: Inhaled versus systemic corticosteroids for the treatment of chronic lung disease in ventilated very low birth weight preterm infants (review), Cochrane Database Syst Rev (4):CD002057, 2007.
Shulenin S, Nogee LM, Annilo T, et al. ABCA3 gene mutations in newborns with fatal surfactant deficiency. N Engl J Med. 2004;350:1296-1303.
Smith VC, Zupanoc JAF, McCormick MC, et al. Trends in severe bronchopulmonary dysplasia rates between 1994–2002. J Pediatr. 2005;146:469-473.
Sosenko IRS, Bancalari E. NO for preterm infants at risk of bronchopulmonary dysplasia. Lancet. 2010;376:308-310.
Stark AR, Carlo WA, Tyson JE, et al. Adverse effects of early dexamethasone treatment in extremely-low-birth-weight infants. N Engl J Med. 2001;344:95-101.
Stevens TP, Blennow M, Myers EH, et al: Early surfactant administration with brief ventilation vs. selective surfactant and continued mechanical ventilation for preterm infants with or at risk for respiratory distress syndrome, Cochrane Database Syst Rev (4):CD003063, 2007.
Stevens TP, Harrington EW, Blennow M, et al: Early surfactant administration with brief ventilation vs. selective surfactant and continued mechanical ventilation for preterm infants with or at risk for respiratory distress syndrome (review), Cochrane Database Syst Rev (4):CD003063, 2007.
Subramaniam P, Henderson-Smart DJ, Davis PG: Prophylactic nasal continuous positive airways pressure for preventing morbidity and mortality in very preterm infants, Cochrane Database Syst Rev (3):CD001243, 2005.
SUPPORT Study Group of the Eunice Kennedy Shriver NICHD Neonatal Research Network. Early CPAP versus surfactant in extremely preterm infants. N Engl J Med. 2010;362(21):1970-1978.
SUPPORT Study Group of the Eunice Kennedy Shriver NICHD Neonatal Research Network. Target ranges of oxygen saturation in extremely preterm infants. N Engl J Med. 2010;362(21):1959-1968.
SUPPORT Study Group of the Eunice Kennedy Shriver NICHD Neonatal Research NetworkCarlo WA, Finer NN, et al. Target ranges of oxygen saturation in extremely preterm infants. N Engl J Med. 2010;362(21):1959-1969.
SUPPORT Study Group of the Eunice Kennedy Shriver NICHD Neonatal Research NetworkFiner NN, Carlo WA, et al. Early CPAP versus surfactant in extremely preterm infants. N Engl J Med. 2010;362(21):1970-1979.
Tan A, Schulze AA, O’Donnell CPF, et al: Air versus oxygen for resuscitation of infants at birth, Cochrane Database Syst Rev (2):CD002273, 2005.
Thome UH, Carlo WA, Pohlandt F. Ventilation strategies and outcome in randomized trials of high frequency ventilation. Arch Dis Child Fetal Neonatal Ed. 2005;90:F466-F473.
Truog WE. 21st-Century use for surfactant? Pediatrics. 2009;123:173-174.
Truog WE, Ballard PL, Norberg M, et al. Inflammatory markers and mediators in tracheal fluid of premature infants treated with inhaled nitric oxide. Pediatrics. 2007;119:670-678.
Tyson JE, Wright LL, Oh W, et al. Vitamin A supplementation for extremely-low-birth-weight infants. N Engl J Med. 1999;340:1962-1968.
Urlesberger B, Grossauer K, Pocivalnik M, et al. Regional oxygen saturation of the brain and peripheral tissue during birth transition of term infants. J Pediatr. 2010;157:740-747.
Walsh MC, Yao Q, Gettner P, et al. Impact of a physiologic definition on bronchopulmonary dysplasia rates. for the National Institute of Child Health and Human Development Neonatal Research Network. Pediatrics. 2004;114:1305-1311.
Watkinson M. Hypertension in the newborn baby. Arch Dis Child Fetal Neonatal Ed. 2002;86:F78-F81.
Watterberg KL, Gerdes JS, Cole CH, et al. Prophylaxis of early adrenal insufficiency to prevent bronchopulmonary dysplasia: a multicenter trial. Pediatrics. 2004;114:1649-1657.
Yeh TF, Lin YJ, Lin HC, et al. Outcomes at school age after postnatal dexamethasone therapy for lung disease of prematurity. N Engl J Med. 2004;350:1304-1312.
Yeh TF, Ling HC, Chang CH, et al. Early intratracheal instillation of budesonide using surfactant as a vehicle to prevent chronic lung disease in preterm infants: a pilot study. Pediatrics. 2008;121:e1310-e1318.
95.5 Aspiration of Foreign Material (Fetal Aspiration Syndrome, Aspiration Pneumonia)
During prolonged labor and difficult deliveries, infants often initiate vigorous respiratory movements in utero because of interference with the supply of oxygen through the placenta. Under such circumstances, the infant may aspirate amniotic fluid containing vernix caseosa, epithelial cells, meconium, blood, or material from the birth canal, which may block the smallest airways and interfere with alveolar exchange of oxygen and carbon dioxide. Pathogenic bacteria may accompany the aspirated material, and pneumonia may ensue, but even in noninfected cases, respiratory distress accompanied by roentgenographic evidence of aspiration is seen (Fig. 95-7).
Postnatal pulmonary aspiration may also occur in newborn infants as a result of prematurity, tracheoesophageal fistula, esophageal and duodenal obstruction, gastroesophageal reflux, improper feeding practices, and administration of depressant medicines. To avoid aspiration of gastric contents, the stomach should be aspirated using a soft catheter just before surgery or other major procedures that require anesthesia or conscious sedation. The treatment of aspiration pneumonia is symptomatic and may include respiratory support and systemic antibiotics (Chapters 103.8 and 389). Gradual improvement generally occurs over 3-4 days.
95.6 Meconium Aspiration
Meconium-stained amniotic fluid is found in 10-15% of births and usually occurs in term or post-term infants. Meconium aspiration syndrome (MAS) develops in 5% of such infants; 30% require mechanical ventilation, and 3-5% die. Usually, but not invariably, fetal distress and hypoxia occur before the passage of meconium into amniotic fluid. The infants are meconium stained and may be depressed and require resuscitation at birth. The pathophysiology is shown in Figure 95-8. Infants with MAS are at increased risk of persistent pulmonary hypertension (Chapter 95.7).
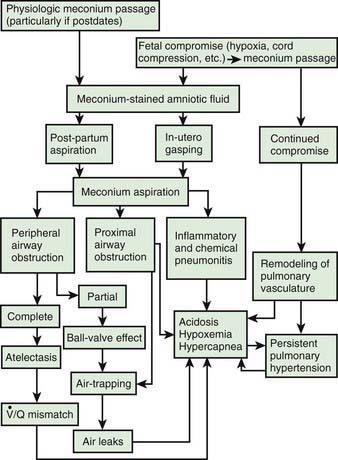
Figure 95-8 Pathophysiology of meconium passage and the meconium aspiration syndrome. , ventilation-perfusion ratio.
(From Wiswell TE, Bent RC: Meconium staining and the meconium aspiration syndrome: unresolved issues, Pediatr Clin North Am 40:955–981, 1993.)
Clinical Manifestations
Either in utero or with the 1st breath, thick, particulate meconium is aspirated into the lungs. The resulting small airway obstruction may produce respiratory distress within the 1st hours, with tachypnea, retractions, grunting, and cyanosis observed in severely affected infants. Partial obstruction of some airways may lead to pneumomediastinum, pneumothorax, or both. Overdistention of the chest may be prominent. The condition usually improves within 72 hr, but when its course requires assisted ventilation, it may be severe with a high risk for mortality. Tachypnea may persist for many days or even several weeks. The typical chest roentgenogram is characterized by patchy infiltrates, coarse streaking of both lung fields, increased anteroposterior diameter, and flattening of the diaphragm. A normal chest roentgenogram in an infant with severe hypoxemia and no cardiac malformation suggests the diagnosis of pulmonary hypertension (Chapter 95.7).
Treatment
Treatment of MAS includes supportive care and standard management for respiratory distress. The beneficial effect of mean airway pressure on oxygenation must be weighed against the risk of pneumothorax. Administration of exogenous surfactant and/or iNO to infants with MAS and hypoxemic respiratory failure or pulmonary hypertension requiring mechanical ventilation decreases the need for ECMO support, which is needed by the most severely affected infants who show no response to therapy. Severe meconium aspiration may be complicated by persistent pulmonary hypertension. Patients with MAS that is refractory to conventional mechanical ventilation may benefit from HFV or ECMO (Chapter 95.7).
Fraser WD, Hofmeyr J, Lede R, et al. Amnioinfusion for prevention of the meconium aspiration syndrome. N Engl J Med. 2005;353:909-917.
Vain NE, Szyld EG, Prudent LM. Oropharyngeal and nasopharyngeal suctioning of meconium-stained neonates before delivery of their shoulders: multicentre, randomized controlled trial. Lancet. 2004;364:597-602.
Wiswell TE, Gannon CM, Jacob J, et al. Delivery room management of the apparently vigorous meconium-stained neonate: results of the multicenter, international collaborative trial. Pediatrics. 2000;105:1-7.
95.7 Persistent Pulmonary Hypertension of the Newborn (Persistent Fetal Circulation)
Pathophysiology
Persistence of the fetal circulatory pattern of right-to-left shunting through the PDA and foramen ovale after birth is due to excessively high PVR. Fetal PVR is usually elevated relative to fetal systemic or postnatal pulmonary pressure. This fetal state normally permits shunting of oxygenated umbilical venous blood to the left atrium (and brain) through the foramen ovale, from which it bypasses the lungs through the ductus arteriosus and passes to the descending aorta. After birth, PVR normally declines rapidly as a consequence of vasodilation secondary to filling of the lungs with gas, a rise in postnatal PaO2, a reduction in PaCO2, increased pH, and release of vasoactive substances. Increased neonatal PVR may be (1) maladaptive from an acute injury (not demonstrating normal vasodilation in response to increased oxygen and other changes after birth); (2) the result of increased pulmonary artery medial muscle thickness and extension of smooth muscle layers into the usually nonmuscular, more peripheral pulmonary arterioles in response to chronic fetal hypoxia; (3) due to pulmonary hypoplasia (diaphragmatic hernia, Potter syndrome); or (4) obstructive as a result of polycythemia or total anomalous pulmonary venous return, or of alveolar capillary dysplasia, which is a lethal autosomal recessive disorder characterized by thickened alveolar septa, increased muscularization of the pulmonary arterioles, a reduced number of capillaries, and misalignment of the intrapulmonary veins. Regardless of etiology, profound hypoxemia from right-to-left shunting and normal or elevated PaCO2 are present (Fig. 95-9).
Clinical Manifestations
Infants with PPHN become ill in the delivery room or within the 1st 12 hr of life. PPHN related to polycythemia, idiopathic causes, hypoglycemia, or asphyxia may result in severe cyanosis with tachypnea, although initial signs of respiratory distress may be minimal. Infants who have PPHN associated with meconium aspiration, group B streptococcal pneumonia, diaphragmatic hernia, or pulmonary hypoplasia usually exhibit cyanosis, grunting, flaring, retractions, tachycardia, and shock. Multiorgan involvement may be present (see Table 92-1). Myocardial ischemia, papillary muscle dysfunction with mitral and tricuspid regurgitation, and biventricular dysfunction produce cardiogenic shock with decreases in pulmonary blood flow, tissue perfusion, and oxygen delivery. The hypoxemia is often labile and out of proportion to the findings on chest roentgenograms.
Baquero H, Soliz A, Neira F, et al. Oral sildenafil in infants with persistent pulmonary hypertension of the newborn: a pilot randomized blinded study. Pediatrics. 2006;117:1077-1083.
Barrington KJ, Finer N: Inhaled nitric oxide for respiratory failure in preterm infants. Cochrane Database Syst Rev (3):CD000509, 2007.
Chambers CD, Hernandez-Díaz S, van Marter LJ, et al. Selective serotonin-reuptake inhibitors and risk of persistent pulmonary hypertension of the newborn. N Engl J Med. 2006;354:579-587.
Finer N, Barrington KJ: Nitric oxide for respiratory failure in infants born at or near term, Cochrane Database Syst Rev (4):CD000399, 2006.
Kulik TJ, Rhein LM, Mullen MP. Pulmonary arterial hypertension in infants with chronic lung disease: will we ever understand it? J Pediatr. 2010;157(2):186-190.
Mugford M, Elbourne D, Field D: Extracorporeal membrane oxygenation for severe respiratory failure in newborn infants, Cochrane Database Syst Rev (16):CD001340, 2008.
Nakajima W, Ishida A, Arai H, et al. Methaemoglobinaemia after inhalation of nitric oxide in infants with pulmonary hypertension. Lancet. 1997;350:1002-1003.
Sen P, Thakur N, Stockton DW, et al. Expanding the phenotype of alveolar capillary dysplasia (ACD). J Pediatr. 2004;145:646-651.
Steinhorn RH, Kinsella JP, Pierce C, et al. Intravenous sildenafil in the treatment of neonates with persistent pulmonary hypertension. J Pediatr. 2009;155:841-847.
Wiswell TE, Knight GR, Finer NN, et al. A multicenter, randomized, controlled trial comparing Surfaxin (lucinactant) lavage with standard care for treatment of meconium aspiration syndrome. Pediatrics. 2002;109:1081-1087.
95.8 Diaphragmatic Hernia
A diaphragmatic hernia is defined as a communication between the abdominal and thoracic cavities with or without abdominal contents in the thorax (Fig. 95-10). The etiology may be congenital or traumatic. The symptoms and prognosis depend on the location of the defect and associated anomalies. The defect may be at the esophageal hiatus (hiatal), paraesophageal (adjacent to the hiatus), retrosternal (Morgagni), or at the posterolateral (Bochdalek) portion of the diaphragm. The term congenital diaphragmatic hernia typically refers to the Bochdalek form. These lesions may cause significant respiratory distress at birth, can be associated with other congenital anomalies, and have significant mortality and long-term morbidity. The overall survival from the CDH Study Group is 67%. The Bochdalek hernia accounts for up to 90% of the hernias seen in the newborn period, with 80-90% occurring on the left side. The Morgagni hernia accounts for 2-6% of congenital diaphragmatic defects. The size of the defect is highly variable, ranging from a small hole to complete agenesis of this area of the diaphragm.
Congenital Diaphragmatic Hernia (Bochdalek)
Diagnosis and Clinical Presentation
CDH can be diagnosed on prenatal ultrasonography (between 16 and 24 wk of gestation) in > 50% of cases. High-speed fetal MRI can further define the lesion. Findings on ultrasonography may include polyhydramnios, chest mass, mediastinal shift, gastric bubble or a liver in the thoracic cavity, and fetal hydrops. Certain imaging features may predict outcome; these include lung to head size ratio (LHR). Nonetheless, no definitive characteristic reliably predicts outcome. After delivery, a chest radiograph is needed to confirm the diagnosis (Fig. 95-11). In some infants with an echogenic chest mass, further imaging is required. The differential diagnosis may include a cystic lung lesion (pulmonary sequestration, cystic adenomatoid malformation) requiring a CT scan or an upper gastrointestinal radiographic series to confirm the diagnosis.
Treatment
Ventilation Strategies
NO is a selective pulmonary vasodilator. Its use reduces ductal shunting and pulmonary pressures and results in improved oxygenation. Although it has been helpful in PPHN, randomized trials have not demonstrated improved survival or reduced need for ECMO when NO is used in newborns with CDH. Nonetheless, it is used in patients with CDH before ECMO is started (Chapter 95.7).
Extracorporeal Membrane Oxygenation
The availability of ECMO and the utility of preoperative stabilization have improved survival of babies with CDH. ECMO combined with paralysis and nasogastric suction may produce a dramatic reduction of the volume of herniated viscera. ECMO is the therapeutic option in children in whom conventional ventilation or conventional ventilation and HFOV fail. ECMO is most commonly used before repair of the defect. Several objective criteria for ECMO have been developed (Chapter 95.7).
Surgical Repair
The ideal time to repair the diaphragmatic defect is under debate. Most centers wait at least 48 hr after stabilization and resolution of the pulmonary hypertension. Good relative indicators of stability are the requirement for conventional ventilation only, a low PIP, and a FIO2 <50. If the newborn was on HFOV, repair is delayed until the child can return to conventional ventilation. If the newborn was on ECMO, an ability to be weaned from this support should be a consideration before surgical repair. In some centers, the repair is done with the cannulas in place; in other centers, the cannulas are removed. A subcostal approach is the most frequently used (Fig. 95-12). This allows for good visualization of the defect and, if the abdominal cavity cannot accommodate the herniated contents, a polymeric silicone (Silastic) patch can be placed. Both laparoscopic and thoracoscopic repairs have been reported, but these should be reserved for only the most stable infants.
Congenital Diaphragmatic Hernia Study Group. Surfactant replacement therapy on ECMO does not improve outcomes in neonates with congenital diaphragmatic hernia. J Pediatr Surg. 2004;39:1632-1637.
Harrison MR, Keller RL, Hawgood SB, et al. A randomized trial of fetal endoscopic tracheal occlusion for severe congenital diaphragmatic hernia. N Engl J Med. 2003;349:1916-1924.
Jaillard SM, Pierrat V, Dubois A, et al. Outcome at two years of infants with congenital diaphragmatic hernia: a population based study. Ann Thorac Surg. 2003;75:250-256.
Konduri GG, Vohr B, Robertson C, et al. Early inhaled nitric oxide therapy for term and near-term newborn infants with hypoxic respiratory failure: neurodevelopmental follow-up. J Pediatr. 2007;150:235-240.
Morini F, Goldman A, Pierro A. Extracorporeal membrane oxygenation in infants with congenital diaphragmatic hernia: a systematic review of the evidence. Eur J Pediatr Surg. 2006;16:385-391.
Smith NP, Jesudason EC, Featherstone NC, et al. Recent advances in congenital diaphragmatic hernia. Arch Dis Child. 2005;90:426-428.
Van Meures K, Congenital Diaphragmatic Hernia Study Group. Is surfactant therapy beneficial in the treatment of the term newborn infant with congenital diaphragmatic hernia? J Pediatr. 2004;145:312-316.
95.9 Foramen of Morgagni Hernia
Akhil Maheshwari and Waldemar A. Carlo
The anteromedial diaphragmatic defect through the foramen of Morgagni accounts for 2-6% of diaphragmatic hernias. Failure of the sternal and crural portions of the diaphragm to meet and fuse produces this defect. These defects are usually small, with a greater transverse than anteroposterior diameter, and are more commonly right-sided (90%) but may be bilateral (Fig. 95-13). The transverse colon or small intestine or liver is usually contained in the hernial sac. The majorities of children with these defects are asymptomatic and are diagnosed beyond the neonatal period. The diagnosis is usually made on chest radiograph when a child is evaluated for another reason. Theanteroposterior radiograph shows a structure behind the heart, and a lateral film localizes the mass to the retrosternal area. Chest CT will confirm the diagnosis. When symptoms occur, they can be recurrent respiratory infections, cough, vomiting, or reflux; in rare instances, incarceration may occur. Repair is recommended for all patients, in view of the risk of bowel strangulation, and can be accomplished laparoscopically or by an open approach. Prosthetic material is rarely required.
95.11 Eventration
Boloker J, Bateman DA, Wung JT, et al. Congenital diaphragmatic hernia in 120 infants treated consecutively with permissive hypercapnia/spontaneous respiration/elective repair. J Pediatr Surg. 2002;37:357-366.
Congenital Diaphragmatic Hernia Study Group. Estimating disease severity of congenital diaphragmatic hernia in the first 5 minutes of life. J Pediatr Surg. 2001;36:141-145.
Congenital Diaphragmatic Hernia Study Group. Surfactant replacement therapy on ECMO does not improve outcomes in neonates with congenital diaphragmatic hernia. J Pediatr Surg. 2004;39:1632-1637.
Harrison MR, Keller RL, Hawgood SB, et al. A randomized trial of fetal endoscopic tracheal occlusion for severe congenital diaphragmatic hernia. N Engl J Med. 2003;349:1916-1924.
Hutcheon JA, Butler B, Lisonkova S, et al. Timing of delivery for pregnancies with congenital diaphragmatic hernia. BJOG. 2010;117:1658-1662.
95.12 Extrapulmonary Air Leaks (Pneumothorax, Pneumomediastinum, Pulmonary Interstitial Emphysema, Pneumopericardium)
Asymptomatic pneumothorax, usually unilateral, is estimated to occur in 1-2% of all newborn infants; symptomatic pneumothorax and pneumomediastinum are less common (Chapter 94). The incidence of pneumothorax is increased in infants with lung diseases such as meconium aspiration and RDS; in those who have undergone vigorous resuscitation or are receiving assisted ventilation, especially if high ventilator support is necessary; and in infants with urinary tract anomalies or oligohydramnios.
Diagnosis
Pneumothorax and other air leaks should be suspected in newborn infants who show signs of respiratory distress, are restless or irritable, or have a sudden change in condition. The diagnosis of pneumothorax is established by radiography, with the edge of the collapsed lung standing out in relief against the pneumothorax (Fig. 95-14); pneumomediastinum is signified by hyperlucency around the heart border and between the sternum and the heart border (Fig. 95-15). Transillumination of the thorax is often helpful in the emergency diagnosis of pneumothorax; the affected side transmits excessive light. Associated renal anomalies are identified by ultrasonography. Pulmonary hypoplasia is suggested by signs of uterine compression (extremity contractures), a small thorax on chest roentgenograms, severe hypoxia with hypercapnia, and signs of the primary disease (hypotonia, diaphragmatic hernia, Potter syndrome).
Treatment
Without a continued air leak, asymptomatic and mildly symptomatic small pneumothoraces require only close observation. Conservative management of a pneumothorax is effective even in selected infants requiring ventilatory support. Frequent small feedings may prevent gastric dilatation and minimize crying, which can further compromise ventilation and worsen the pneumothorax. Breathing 100% oxygen in term infants accelerates the resorption of free pleural air into blood by reducing the nitrogen tension in blood and producing a resultant nitrogen pressure gradient from the trapped gas in the blood, but the clinical effectiveness is not proven and the benefit must be weighed against the risks of oxygen toxicity. With severe respiratory or circulatory embarrassment, emergency aspiration using a soft small catheter introduced with a needle is indicated. Either immediately or after catheter aspiration, a chest tube should be inserted and attached to underwater seal drainage (see Fig. 95-14). If the air leak is ongoing, continuous suction (−5 to −20 cm H2O) may be needed to evacuate the pneumothorax completely. A pneumopericardium requires prompt evacuation of entrapped air. Severe localized interstitial emphysema may respond to selective bronchial intubation. Judicious use of sedation in an infant fighting a ventilator may reduce the risk of pneumothorax. Surfactant therapy for RDS reduces the incidence of pneumothorax.
95.13 Pulmonary Hemorrhage
Acute pulmonary hemorrhage may rarely occur in previously healthy full-term infants. The cause is unknown. Pulmonary hemorrhage may manifest as hemoptysis or blood in the nasopharynx or airway with no evidence of upper respiratory or gastrointestinal bleeding. Patients present with acute, severe respiratory failure requiring mechanical ventilation. Chest radiographs usually demonstrate bilateral alveolar infiltrates. The condition usually responds to intensive supportive treatment (Chapter 401).