Respiratory system
Respiration is the totality of those processes that ultimately result in energy being supplied to the cells. In pregnancy the respiratory system undergoes significant changes. The increased metabolic needs of the pregnant woman, fetus, and placenta require improved maternal respiratory efficiency to ensure adequate oxygenation. The placenta functions as the respiratory system for the fetus, providing nutrients and oxygen and removing carbon dioxide. This makes the relationship between the mother and fetus not only intimate but also interdependent. At birth, tremendous energy is expended by the neonate to generate sufficient negative pressure to convert to an air-liquid interface in the alveoli and maintain functional residual capacity (FRC). Transitional events continue over the first week of life as the neonate adjusts to the new environment, more alveoli are recruited, and the surfactant system stabilizes. This chapter examines alterations in the respiratory system and acid-base homeostasis during the perinatal period and their implications for the mother, fetus, and neonate. Fetal blood gases and responses to hypoxia are discussed in Chapter 6.
Maternal physiologic adaptations
Antepartum period
Pregnancy is associated with major changes in the respiratory system, including changes in lung volumes and ventilation. Both biochemical and mechanical factors interact to increase the delivery of oxygen and the removal of carbon dioxide.147 Otolaryngeal changes are discussed in Chapter 15.
Factors influencing respiratory function
Mechanical factors.
The gradual enlargement of the uterus leads to changes in abdominal size and shape, shifting the resting position of the diaphragm up to 4 cm above its usual position to accommodate the growing uterus (Figure 10-1).23,43,96,147,247 The thoracic circumference increases by 5 to 7 cm and the transverse diameter of the chest by about 2 cm in response to the increased intraabdominal pressure with a flaring of the lower ribs.43,96,247 The subcostal angle progressively increases from 68 to 103 degrees in late gestation.23,96,247 Not all of these changes can be attributed to intraabdominal pressure, in that the increase in the subcostal angle occurs before the increasing mechanical pressure.147,247,250 Relaxation of the ligamentous rib attachments increases rib cage elasticity. This change is similar to those seen in the pelvis and mediated by similar factors, such as relaxin (see Chapter 15).
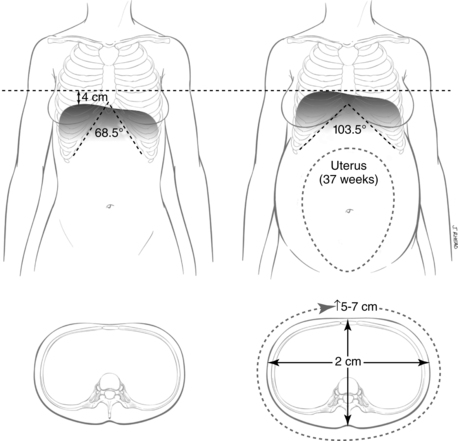
Although the aforementioned changes suggest that diaphragmatic motion decreases, the reverse is actually true. Diaphragmatic movement actually increases about 2 cm during pregnancy, with the major work of breathing being accomplished by the diaphragm rather than by the costal muscles.23,43,96,147 These changes in thoracic structures modify the abdominal space, perhaps in preparation for the increase in uterine size and peak at 37 weeks.96 The changes in thoracic configuration have a major impact on lung volumes, however, to which the increasing intra-abdominal pressure contributes.
Biochemical factors.
Hormones and other biochemical factors are important in stimulating changes in the respiratory system in pregnancy. These substances can act centrally via stimulation of the respiratory center or directly on smooth muscle and other tissues of the lung. The most important influences are mediated by progesterone, in combination with estradiol (which increases progesterone receptors in the hypothalamus) and prostaglandins (PGs).108
Serum progesterone levels increase progressively throughout pregnancy and are thought to be a major factor in the changes seen in ventilation.250 Progesterone is a respiratory stimulant. The administration of progesterone to nonpregnant subjects increases minute ventilation and enhances responses to hypercapnia.96,108,147 This suggests an increased sensitivity to carbon dioxide by the respiratory center and that progesterone lowers the carbon dioxide threshold of the respiratory center.3,108,250 For example, in the nonpregnant woman, an increase of 1 mmHg (0.13 kPa) in the PaCO2 results in a 1.5 L/min increase in ventilation, whereas during pregnancy the same 1-mmHg increase in PaCO2 leads to a 6-L/min increase in ventilation.247 More than 60% of the increase in CO2 sensitivity occurs by 20 weeks’ gestation.213
This increased sensitivity most likely contributes to the sensation of dyspnea that is experienced by many pregnant women and may also lead to some of the hyperventilation that occurs during the second stage of labor after pushing efforts (as carbon dioxide levels increase during breath holding).147 Progesterone may also exert a local effect on the lung, causing water retention in the lung that results in decreased diffusion capacity. Therefore hyperventilation is an attempt to maintain normal PO2 levels.247
Lung volume
Changes in lung volumes begin in the middle of the second trimester and are progressive to term (Figure 10-2). The most significant change is a 30% to 40% (from 500 to 700 mL) increase in VT, with a progressive 15% to 20% decrease in expiratory reserve volume (ERV), 20% to 25% decrease in residual volume (RV), and 20% to 30% decrease in FRC.96 The FRC is further decreased in the supine position at term.96 The decreased FRC may increase uptake and elimination of inhaled anesthetics.23 Along with the change in VT, there is a concomitant increase in inspiratory capacity (IC) by 5% to 10%, thereby allowing the total lung capacity (TLC) to remain relatively stable, with a slight decrease of up to 5% by term.23,96,147 Vital capacity (VC) and inspiratory reserve volume (IRV) are essentially unaltered, although some literature notes a small change in IRV.147,247,250 Peak expiratory flow rates tend to decrease during pregnancy, possibly due to mechanical changes in the respiratory system.95 Physiologic dead space is increased by 60 mL.247
The decreased RV, in conjunction with an elevated metabolic rate, increases the risk of hypoxia if respiratory depression occurs.250 On the other hand, forced expiratory volume in 1 second (FEV1) does not change nor does the FEV1/VC ratio.147,250 These assessments are used in individuals with asthma and are generally thought to be reliable in pregnant women.43,147,250 However, recent studies suggest that peak inspiratory flow rates are altered by maternal position (lower in supine versus standing or sitting) and may in fact decrease to some extent during pregnancy.95
Changes in lung volumes result from the elevation of the diaphragm and the changes in the configuration of the chest. The alteration in RV is also the result of a 35% to 40% decrease in chest wall compliance; lung compliance is unchanged. 43,96,147,247 The decreased chest wall compliance is caused by hormonal influences as well as changes in abdominal pressure. This reduction in compliance allows for more inward movement of the chest wall and reduces the amount of trapped air (residual trapped volume) that contributes to the RV. Therefore the RV decreases 200 to 300 mL. This, along with an approximately 200 mL decrease in ERV, brings the total deficit in the FRC to 500 mL (see Figure 10-2). The FRC falls progressively from 20 weeks’ gestation for a change of 10% to 24% by term.147,250
Lung function
Changes in lung function are related to three major factors: ventilation, airflow, and diffusing capacity.147 Oxygen consumption increases during pregnancy; however, the arterial oxygen pressure (PaO2), though it increases, does not change significantly, even though the arteriovenous oxygen difference decreases. This indicates that there must be a change in ventilation.247
Ventilation.
Minute ventilation (VT x Respiratory rate [RR]) increases up to 30% to 50% during pregnancy.23,147 Changes begin by 8 weeks’ gestation and result in an increase in minute volume, from 6.5 to 7.5 L/min in early pregnancy to 10 to 10.5 L/min at term.108 The elevated resting ventilation exceeds the demands in oxygen consumption, indicating that women tend to hyperventilate during pregnancy.147,247,250 The greater increase in resting ventilation is thought to be due to the stimulatory effects of progesterone on the respiratory center.147 Progesterone may also increase red blood cell levels of carbonic anhydrase B, which facilitates CO2 transfer and lowering of the PCO2.247 The hyperventilation of pregnancy and associated respiratory system alterations are also mediated by the interaction of acid-base balance changes, increased breathing drive, increased central chemoreflex sensitivity, increased metabolism and decreased cerebral blood flow.109–111
The increase in minute ventilation is probably due primarily to the 30% to 40% increase in VT rather than changes in respiratory rate, which usually is unchanged.23,43,96,247 It is much more efficient to increase alveolar ventilation through an increase in VT than with a proportionately equal increase in respiratory rate. The increased metabolic rate and carbon dioxide production also influence VT changes.250 Maximal inspiratory and expiratory pressures are not altered in pregnancy. The reduced RV further enhances alveolar ventilation. This change in RV decreases the amount of gas mixing that occurs with each tidal exchange in the alveolus, thereby improving gas exchange at the alveolar level. Alveolar ventilation increases by 50% to 70% during pregnancy.15,147,247
Airflow.
Airflow is dependent upon resistance encountered in the bronchial tree. Two important determining factors for resistance are smooth muscle tone in the bronchi and the degree of congestion encountered in bronchial wall capillaries. Despite the changes in minute ventilation and the concomitant increase in alveolar ventilation, the work of breathing (airway resistance and lung compliance) remains unchanged. In the larger airways, congestion has very little to do with resistance. Airway resistance does not change significantly during pregnancy because of a balance between bronchoconstricting (PGF2α, decreased RV, and decreased PaCO2) and bronchodilating (PGE2 and progesterone) forces.96,147
Assessment of small airway function is most often determined by evaluation of closing volume (CV) and closing capacity (CC). CV is the point at which the small airways close (collapse and cease to ventilate) in the lowest part of the lung. Small airway (<1 mm) patency is believed to be the result of transpulmonary pressure, compliance of the airway walls, and the presence of sufficient surfactant. Closure in the lung bases normally occurs somewhere between the RV and FRC. CV is usually expressed as a percentage of vital capacity (CV/VC). CC is the term applied to the sum of the CV and RV (CC = CV + RV) and is expressed as a percentage of total lung capacity (CC/TLC).43,147,250
Under normal circumstances, airway closure does not occur during tidal breathing. When closure occurs at a higher than normal volume, however, gas exchange may be affected because ventilation to the lung bases is decreased. In pregnancy, airway closure above the FRC has been reported near term and is attributed to the 20% to 30% decrease in ERV.147 Airway closure is more likely to occur in supine versus sitting.147 This alteration changes gas distribution and may result in a decrease in oxygen content and PaO2 in the third trimester.43,147,247,250 When airway closure is at or above FRC, there is the possibility of altering PaO2 due to ventilation-perfusion (V./Q.) mismatch in the lung bases. If these differences are significant, compensatory maternal physiologic responses such as an increase in respiratory rate may result. However, this is not a usual event in healthy women who compensate by changes in the hemoglobin-oxygen dissociation curve (see “Acid-Base Changes”).26
Diffusing capacity.
Diffusing capacity refers to the ease with which gas is transferred across the pulmonary membrane. Diffusion capacity of carbon dioxide may show an increase or no change in early pregnancy, followed by a decrease, reaching a plateau in the second half of pregnancy in many women. These changes are not thought to be clinically significant.23,33,96,147 Carbon dioxide production increases by 30% due to changes in cholesterol and fat metabolism.250 Oxygen consumption increases by 32 to 58 mL/min.171 This change is accounted for by the needs of the fetus (average of 12 mL/min) and placenta (4 mL/min), and by increased maternal cardiac output (7 mL/min), ventilation (2 mL/min), renal function (7 mL/min), and extra tissue in the breasts and uterus (5 mL/min). The increase in carbon dioxide production exceeds the changes in oxygen consumption, leading to an increase in the respiratory quotient from 0.70 to 0.83 by term.247
Acid-base changes
Oxygen consumption, carbon dioxide production, and basal metabolic rate all increase due to the increased metabolic demands from the mother, placenta, and fetus.96 The normal pregnant woman is in a state of compensated respiratory alkalosis, which is thought to be the result of the effects of progesterone on the respiratory system and lung volume changes, especially the increased minute volume.247,250 The result is a reduction in arterial and alveolar carbon dioxide and a slight increase in PaO2. The fall in PCO2 begins early in pregnancy, paralleling changes in ventilation.147,247 Definitions of common terms used in acid-base physiology are listed in Table 6-1 on p. 165.
The purpose of the respiratory alkalosis seems to be facilitation of carbon dioxide transfer from the fetus to the mother by increasing the arterial carbon dioxide pressure (PaCO2) gradient. Hyperventilation leads to average PaCO2 values of 27 to 32 mmHg (3.59 to 4.25 kPaa) and a concomitant decrease in serum bicarbonate levels to between 18 and 21 mEq/L (mmol/L) with a base deficit of −3 to −4 mEq/L (mmol/L).23,147 The latter is a consequence of increased renal excretion of bicarbonate, reflecting a metabolic compensation for the lower PaCO2. The lower PaCO2 produces a gradient within the intervillous space that enhances fetal offloading of carbon dioxide.23 This may be the primary function of the hyperventilation of pregnancy.160 PaCO2 values vary with the altitude at which the mother resides; the decrease in PaCO2 is greater at higher altitudes (compensatory hyperventilation to maintain and adequate PaO2.23,96,247 The pH increases to the high end of normal (7.40 to 7.45) to maintain homeostasis. These changes are stable throughout pregnancy until the onset of labor.33,43,147 The reduction in blood buffer reduces the mother’s ability to compensate for a metabolic acidosis that could develop during prolonged labor or other states in which tissue perfusion may be reduced.
In contrast, PaO2 levels increase from those of prepregnancy (95 to 100 mmHg [12.63 to 13.30 kPaa]) because of the increase in alveolar ventilation. During the first trimester, PaO2 levels range from 106 to 108 mmHg, dropping to 101 to 104 mmHg (13.43 to 13.83 kPaa) by the third trimester.247 Even though the PaO2 level remains elevated, the alveolar-arterial PO2 gradient (AaDO2) is unchanged throughout most of pregnancy, but may increase near term due to an attempt to offset hyperventilation.147 Oxygen delivery is maintained within normal limits during pregnancy due to the increased carbon dioxide, which compensates for changes in the respiratory system and the decrease in red blood cells.247 Supine positioning versus sitting in late pregnancy further decreases PaO2 levels and increases the AaDO2 gradient.147,247 However, these changes are thought to have little clinical significance.147
Oxygen-hemoglobin dissociation curve.
The oxygen-hemoglobin dissociation curve (see Box 10-1 below) demonstrates that once the plateau of the curve is achieved it takes large changes in oxygen concentration in order to make small changes in SaO2. The P50 increases from 26 to 30 mmHg (3.45 to 3.99 kPaa) by term (Figure 10-3), thus decreasing the affinity of hemoglobin and enhancing transfer of oxygen from mother to fetus.247,250 These changes are mediated by the increase in 2,3-diphosphoglycerate (2,3-DPG), which is stimulated by the increase in maternal pH.43 The increased 2,3-DPG shifts the oxygen-hemoglobin dissociation curve to the right to enhance oxygen release to the fetus.
It is unclear whether the small changes seen in PaO2 levels during pregnancy are significant to the maternal-fetal oxygen gradient and therefore to the fetus. Considering that fetal PaO2 levels are between 25 and 35 mmHg (3.32 and 4.65 kPaa), this seems unlikely. However, this increase may reflect increased maternal pulmonary circulation, with a greater volume of blood contained within the pulmonary vasculature at any given point in time. This may be significant at higher altitudes (see “Effects of Altitude and Air Travel”). SaO2 does not change significantly during pregnancy, although saturations below 96% may be seen in women who smoke.
Intrapartum period
The major effect of labor upon the respiratory system is related to the increased muscular work, metabolic rate, and oxygen consumption, which increases 40% to 60% during labor.23 Consequently, alterations in ventilation and acid-base status can be anticipated. The healthy woman tolerates these changes since oxygen delivery is much greater than needs, but this can become a problem in the compromised woman.247
With the onset of labor, there is an increased demand for oxygen; oxygen consumption increases with uterine muscle activity. If there is insufficient time for uterine relaxation and restabilization after a contraction, oxygen content is lower and myometrial hypoxia as well as metabolic acidosis may occur with the next contraction. Over time this can lead to inadequate oxygenation, which increases the severity of the pain experienced. Most studies have evaluated respiratory system changes during active and painful labor. There are few studies evaluating the labor process with the use of sporadic analgesia and psychoprophylaxis. The pain experienced during labor is the result of the interaction of a number of factors (see Chapter 15). The subjective component includes the discomfort that is perceived by the mother. Objective factors are related to changes in the functioning of the cardiorespiratory system, alterations in the autonomic nervous system, and physical changes that occur during labor and delivery.
Ventilatory alterations related to pain vary significantly from patient to patient; therefore, each laboring woman must be evaluated individually. Changes seen during the intrapartum period include an increase in respiratory rate and a change in VT, with a tendency toward further hyperventilation. Hyperventilation is a natural response to pain and becomes evident as the pain and apprehension of labor increase. Hyperventilation is diminished when pain is alleviated.21 Maximal inspiratory pressure decreases during strong expulsive efforts, possibly as a result of development of transient diaphragmatic fatigue.
Although pain seems to be the major cause of this hyperventilatory response, anxiety, drugs, and the use of psychoprophylactic breathing exercises can contribute to the elevated respiratory rate. VT may be further increased during the second stage of labor as hyperventilation following breath holding with expulsive efforts is encountered. Risks of the Valsalva maneuver are discussed in Chapter 4.
Increases in oxygen uptake and minute ventilation are seen during labor, with a significant increase noted between early latent and active phases. Oxygen consumption increases 40% to 60% during labor with further increases (up to twofold) with contractions.33,247 This is balanced by an increase in cardiac output. Women with low oxygen delivery may become compromised with inadequate oxygen delivery to both the woman and fetus.247 The increase in ventilation can lead to a progressive and substantial decline in PaCO2. There are wide variations in values between patients; however, a PaCO2 level around 25 mmHg is representative of what might be encountered during the first stage of labor. A transient decline in PaCO2 occurs with each contraction until cervical dilation is complete, at which time the decline in PaCO2 may be seen even between contractions.
The changes in PaCO2 are markedly reduced or eliminated when continuous lumbar epidural anesthesia is used during labor. Variables affecting PaCO2 levels that need to be considered include breath holding (which elevates PaCO2 levels), compensatory hyperventilation following breath holding, length of contractions, and frequency of contractions. The timing of analgesia administration as well as the frequency and efficacy of the analgesia may also alter PaCO2 values.43 The respiratory alkalosis that ensues from hyperventilation is normally associated with a drop in base excess and possibly a decrease in arterial pH, according to some researchers. Others have documented a rise in pH. In either case, the degree of change indicates that labor and hyperventilation are highly significant events that may lead to alterations in physiologic parameters.21
Significant changes in acid-base status due to hyperventilation and increased oxygen consumption are potentially hazardous to both the mother and fetus.21 Extremely low PaCO2 levels result in cerebral vasoconstriction and possibly reduce intervillous perfusion and blood flow. The alkalemia that results shifts the oxygen-hemoglobin dissociation curve to the left. This shift impairs the release of oxygen from maternal blood to fetal blood, thereby decreasing the availability of oxygen to the fetus during a time when oxygenation may already be impaired due to uterine contractions.21 Hyperventilation may lead to dizziness and tingling in the mother due to low PaCO2 levels. Interventions can include counting respirations out loud to help the mother slow her respiratory rate, letting her know when the contraction is ending so that she can begin to relax, avoiding the Valsalva maneuver, and promoting deep breathing between contractions to cleanse the system and promote oxygenation and restabilization.
The acid-base changes encountered in the first and second stages of labor quickly reverse in the third stage and postpartum period with compensatory respiratory efforts. These efforts are largely due to a decrease in respiratory rate. Acid-base levels return to pregnancy values within 24 hours of delivery and to nonpregnant levels by several weeks after delivery. Table 10-1 summarizes the changes in arterial blood gases during the intrapartum period.
Table 10-1
Maternal Blood Gas Alterations During the Intrapartum Period
STAGE OF LABOR | PARAMETER | RANGE VALUE | ACID-BASE STATUS |
Early | PaCO2 | 21 to 26 mmHg (2.79 to 3.45 kPaa) | Respiratory alkalosis |
Plasma base deficit | −0.9 to 26.9 mEq/L (mmol/L) | ||
Blood pH | 7.43 to 7.49 | ||
End of first stage | PaCO2 | 21 to 35 mmHg (2.79 to 4.65 kPaa) | Mild metabolic acidosis compensated by respiratory alkalosis |
Plasma base deficit | −1.2 to 29.2 mEq/L (mmol/L) | Mild respiratory acidosis during bearing down | |
Blood pH | 7.41 to 7.54 | ||
End of second stage (delivery) | PaCO2 | 16 to 24 mmHg (2.12 to 3.19 kPaa) | Metabolic acidosis uncompensated by respiratory alkalosis |
Plasma base deficit | −2.3 to −12.3 mEq/L (mmol/L) | ||
Blood pH | 7.37 to 7.45 |
From Burgess, A. (1979). The nurse’s guide to fluid and electrolyte balance. New York: McGraw-Hill.
Research on the effects of labor on acid-base status has been conducted in conjunction with the delivery of epidural analgesia. Psychoprophylaxis (psychoanalgesia) has been shown to reduce the need for medication, reduce tension and pain by self-report, and engender a positive attitude toward the labor and delivery experience. Lamaze psychoprophylaxis is based on the hypothesis that childbirth is a natural physiologic process and that pain can be minimized through education and specified exercises. Education and antenatal preparation are designed to reduce anxiety through knowledge of the processes of labor. Relaxation techniques are designed to reduce skeletal muscle spasm and tension that may contribute to pain. Reduction of pain sensations and perception is achieved through distraction by utilizing conditional responses such as breathing patterns and other nonpharmacologic measures (see Chapter 15).
Postpartum period
Chest wall compliance changes immediately after delivery due to a decrease in pressure on the diaphragm and reduction in pulmonary blood volume. A 20% to 25% increase in static compliance has been reported following delivery. Changes in rib cage elasticity may persist for months after delivery.250 VT and RV return to normal soon after delivery, whereas ERV may remain in an abnormal state for several months. As progesterone levels fall in the first 2 days after delivery, PaCO2 levels rise. Diffusing capacity, which at term is slightly below postpartum levels, increases during the postpartum period. Overall, anatomic changes and ventilation return to prepregnant status by 24 weeks after delivery, although the subcostal angle tends to remain about 20% wider than prepregnancy vaues.96
Clinical implications for the pregnant woman and her fetus
The respiratory changes that occur with pregnancy can be annoying as well as limiting in some circumstances. Common complaints and experiences include dyspnea, capillary engorgement of the upper respiratory tract, and altered exercise tolerance. As a result, modifications in activity levels as well as in the activities themselves may need to be considered. Discussions with women regarding their usual activities can help provide sufficient information for determining when change is needed. In addition, changes in the maternal respiratory system can influence the course of disease processes (e.g., asthma in some women), affecting not only the mother but also the fetus. Specific areas addressed in this section include dyspnea of pregnancy, upper respiratory capillary engorgement, respiratory infections, asthma, smoking, and anesthesia. Exercise is discussed briefly; further information can be found in Chapter 9.
Dyspnea
The sensation of dyspnea is reported by 60% to 70% of pregnant women, usually beginning during the first or second trimester (Figure 10-4). A maximal incidence is reached between 28 and 31 weeks and remains relatively stable to term.147,247,250 Because abdominal girth has not increased significantly at the time of dyspnea onset, intraabdominal pressure cannot be ascribed as the cause.
Dyspnea during pregnancy is a physiologic dyspnea; that is, it occurs even at rest or with mild exertion.250 The exact cause remains unclear, but it is thought to be due to the increased respiratory drive and load, changes in oxygenation, or a combination of these events.250 Increased sensitivity to carbon dioxide and hypoxia may be important contributing factors, especially in early pregnancy. During late pregnancy, mechanical factors may aggravate these changes.171 The increased VT and lower PaCO2 have also been implicated. In addition, the heightened maternal awareness of the normal hyperventilation of pregnancy might result in the sensation of dyspnea. An improvement in symptoms in some women with increasing gestation suggests an adjustment to this normal process.
Dyspnea can be quite uncomfortable and anxiety provoking. The hyperventilation and dyspnea may decrease the ability of some pregnant women to maintain their usual activity levels. Pathologic dyspnea—which may occur with disorders such as pulmonary emboli (a complication sometimes seen in pregnancy)—must be differentiated from physiologic dyspnea during pregnancy. Pathologic dyspnea is characterized by respiratory rates greater than 20, PCO2 below 30 mmHg (3.99 kPaa) or greater than 35 mmHg (4.66 kPaa), or abnormal FEV1.250
Upper respiratory tract capillary engorgement
Hormonal changes (especially the increase in estrogens) along with the increased blood volume, hyperemia, edema, glandular hypersecretion and increased phagocyte activity alter the mucosa of the oro- and nasopharynx with capillary engorgement throughout the respiratory tract.96 Progesterone may contribute to engorgement by inducing vascular smooth muscle relaxation and nasal vascular pooling. Increased circulating blood volume may also play a role in this pooling. The results of these changes can be uncomfortable to some women and may be exacerbated in women with preeclampsia.137 With preeclampsia, the airways may be narrower due to soft tissue edema.169 In some situations these changes can be hazardous. For example, swelling of the airway along with other physiologic changes in pregnancy (e.g., weight gain, changes in the gastrointestinal system, and increased total body water) increase the risk for the pregnant woman who requires intubation for obstetric or nonobstetric problems.23,71,169 The rate of failed intubations is seven to eight times higher during pregnancy.23
The nasopharynx, larynx, trachea, and bronchi may become swollen and reddened. For some individuals this may be uncomfortable, but it usually does not pose any unusual difficulties. These symptoms can be markedly aggravated with minor upper respiratory infections and preeclampsia. The swelling can lead to inflammation (noninfective in nature) that causes changes in the voice (e.g., hoarseness), make nose breathing difficult, and increase the incidence of nosebleeds. Abrasions and lacerations of the mucosa may occur, and bleeding may ensue.21,71,169
Rhinitis (defined as nasal congestion that lasts 6 or more weeks without signs of infection or allergy) is seen in up to 30% of all pregnant women and in approximately two thirds of those who smoke.62,84,96,137 These symptoms may appear any time but usually begin in the second trimester and parallel increasing estrogen levels.137 Estrogens and placental growth hormone (a stimulator of nasal mucosal growth) have been implicated in the etiology of pregnancy rhinitis.62,84,96 Rhinitis usually disappears by 48 hours postpartum, but may take up to 2 weeks in some women.96 Upper respiratory tract changes may also increase snoring and exacerbate sleep-disordered breathing or increase obstructive sleep apnea.23,26 These changes are discussed further in Chapter 15.
Exercise
The effect of exercise on the respiratory system is related to alveolar ventilation and is dependent upon the age, body weight, body composition, and physical condition of the individual. Cardiovascular function, uterine blood flow, respiratory function, blood gases, aerobic capacity, metabolism, temperature, and psychologic state are all affected by exercise. Cardiorespiratory changes with exercise during pregnancy are discussed further in Chapter 9.
The usual changes in maternal respiratory function during pregnancy are similar to those with mild to moderate exercise.111,180 Studies of respiratory rates with exercise in pregnant and nonpregnant women demonstrate that respiratory rates in pregnant women are higher than those in nonpregnant women during mild exercise, but this difference disappears during moderate exercise. VT and minute volume remain higher in pregnant subjects during all levels of exercise.180 Ventilation increases 38% and oxygen consumption is 15% greater during exercise in pregnant versus nonpregnant women.147,180 The efficiency of gas exchange does not seem to be impaired in the pregnant woman during exercise. During prolonged exercise, however, PaO2 increases and PaCO2 decreases. This decrease in PaCO2 is most likely due to the effects of progesterone on the respiratory center. Oxygen consumption also increases with advancing gestational age. Part of this increase is due to the increased work of carrying the extra weight associated with pregnancy. Exercise-induced changes in acid-base balance are similar in pregnant and nonpregnant women.
Effects of altitude and air travel
With increasing altitude, compensatory decreases in PaO2 and changes in other parameters can be seen. These are an attempt by the maternal system to maintain higher PaO2 levels under relatively hypoxic conditions.147,153 The change in altitude has a significant effect on oxygen saturation and changes the oxygen-hemoglobin dissociation curve. Pregnant women living at high altitude have a higher minute ventilation, FRC, TLC, forced VC, forced expiratory volume, and diffusion capacity than women at sea level.23,121 The hyperventilation of pregnancy is also accentuated, and maternal dyspnea may be more prominent; therefore patients need to be given assurance that it is normal.8 The woman increases her ventilation and oxygen saturation during the first trimester, while diffusing capacity decreases to the third trimester, leading to decreased oxygen content by term. These changes are not significant if the woman comes from a family that has lived at altitude for at least 3 generations.23,121 Pregnancy at high altitude is also associated with morphologic differences in placental villi with increased capillary diameter.64 Women living at altitude have lower birth weight infants. Birth weight is decreased by an average of 100 g for each 3000 ft (1000 m) increase in altitude.23
Commercial aircraft are usually pressurized to 6000 to 8000 feet (1829 to 2434 meters) above sea level, so flying results in a transient exposure to altitude. During ascent, the pregnant woman may experience transient cardiopulmonary adaptations (increased heart rate and blood pressure; decreased aerobic capacity).4 The fetal heart rate may also increase transiently during both takeoff and landing, but stays within normal limits.71 Pregnant women who travel by airplane may also experience an increase in dyspnea and respiratory rate as their bodies attempt to compensate for the increased altitude. Fetal hemoglobin (HbF) and the fetal circulation protect the fetus from desaturation during commercial flights.201 Thus flying in commercial aircraft is generally not a risk in women with uncomplicated pregnancies.4,35,71,145 The American College of Obstetricians and Gynecologists states that “in the absence of obstetric or medical complications, pregnant women can observe the same general precautions for air travel as the general population and can fly safely.”4 Most airlines allow travel up to 36 weeks of pregnancy, with travel up to 7 days before the woman’s expected due date permitted with a certificate from their health care provider.4 For international travel the cutoff may be earlier.
Pregnant women may be at greater risk of thromboembolism during air travel due to changes in hemostasis (see Chapter 8) and hemoconcentration due to the low cabin humidity (<25%) and should be sure to stretch, perform isometric exercises, and walk around the cabin at regular intervals.201 However, a retrospective study did not find any increased risk of thromboembolism up to 2 weeks after air travel.71 Because of the low cabin humidity, the woman should also maintain hydration with frequent intake of nonalcoholic beverages, because hydration is important for placental blood flow.201
Pulmonary disease and pregnancy
Pulmonary disorders may be aggravated by the changes in the respiratory system during pregnancy. Respiratory infections and asthma are discussed here. Women with pulmonary hypertension (see Chapter 9) and cystic fibrosis (see Chapter 1) are also at increased risk for complications during pregnancy. Outcomes depend on their status at the onset of pregnancy.28
Adult respiratory distress syndrome (ARDS) is an acute lung injury involving diffuse interstitial infiltrates, decreased lung compliance, and hypoxia.33 ARDS is usually triggered by sepsis but may also be triggered by other factors, including disseminated intravascular coagulation (see Chapter 8), preeclampsia, amniotic fluid embolism, abruptio placenta, or fetal demise.147,247 Physiologic changes during pregnancy, especially changes in colloid osmotic pressure, may increase the risk of ARDS following lung injury.33
Respiratory infection
Changes in the immune (see Chapter 13) and pulmonary systems in the pregnant woman, along with upper airway hyperemia and edema, not only make having an upper respiratory infection more uncomfortable but can also potentiate movement of the infection into the lungs.197 Infections associated with lung involvement can potentially increase airway resistance, thereby increasing the work of breathing, and lead to decreased VT and RV. This may lead to decreased maternal and subsequently fetal PaO2 levels. The increased oxygen requirements of pregnancy, elevated diaphragm, decreased FRC, increased lung water, and changes in closing volume may increase the severity of respiratory problems.27,43 Avoidance of those situations in which infections might be contracted and avoidance of individuals carrying infections when possible is a good practice. Most upper respiratory infections are only annoyances and do not lead to significant consequences for the mother or fetus.
Pneumonia, although rare, is a common cause of nonobstetric maternal death in North America.27,84 The prevalence of bacterial pneumonia is similar during pregnancy in nonpregnant, healthy women.57,84,247 However, pregnant women are more susceptible to viral pneumonia and the pneumonia may progress more rapidly during pregnancy.33,84,247 The risk of pneumonia increases with each trimester and by the third trimester, the risk of hospitalization from pneumonia is five times higher in pregnant than nonpregnant women.84,247 The risk of influenza related morbidity is 10.5/10,000 during pregnancy versus 1.91/10,000 in nonpregnant women.84 As a result, influenza vaccinations are recommended for all pregnant women during flu season, regardless of trimester.34 Pregnancy may also increase the risk of varicella pneumonia.84,247 Pneumonia increases the risk of preterm labor, altered fetal growth, and maternal and perinatal mortality.33,34,84,247 Although chronic infections such as tuberculosis (TB) have often been reported to reactivate and worsen with pregnancy, recent evidence suggests that in women with drug-sensitive TB who have received adequate therapy, fetal and maternal outcomes are generally good.147,247 Respiratory infections are discussed further in Chapter 13 (see section on Risk of Maternal Infection).
Asthma
Asthma is an obstructive disease characterized by increased airway resistance, decreased expiratory flow rates, hyperinflation with premature airway closure, and some loss of lung compliance. These factors lead to an increase in the work of breathing. Along with this, hyperinflation and exaggerated negative pleural pressures can lead to increased demands on the right ventricle. This can be seen as a rise in pulmonary arterial pressure. These factors decrease left stroke volume, arterial systolic pressure, and pulse pressure.147
The restrictive processes in asthma are usually reversible and are due to an increased responsiveness of the airways to a variety of stimuli. When stimulated, the characteristic responses include contraction of the bronchial smooth muscle, mucous hypersecretion, and mucosal edema. The mechanism for this responsiveness is unclear and may be different from patient to patient.147
Several mechanisms have been implicated in the etiology of asthma. These include an immunologic response, blocked β-adrenergic function, β-adrenergic amine deficiency, cholinergic dominance, intrinsic smooth muscle defect, or some combination of these. Other possibilities include an imbalance in cyclic nucleotides. Cyclic adenosine monophosphate (cAMP) and cyclic guanosine monophosphate (cGMP) are involved in the modulation of airway tone, with the former contributing to bronchodilation and the latter to bronchoconstriction.41 A major pathogenic characteristic is airway inflammation with release of inflammatory mediators (e.g., leukotrienes, histamine, eosinophil chemotactic factor of anaphylaxis, platelet-activating factor), and disruption of the mucosal epithelial barrier.147
The prevalence of asthma has increased in the general population, leading to an increase in pregnant women with asthma.171 As a result, asthma is the most common pulmonary problem seen during pregnancy, occurring in approximately 8% of pregnant women.173 Asthma may improve (about 23%), worsen (about 30%), or remain unchanged (about 47%) during pregnancy.57 Trying to predict an individual’s course during pregnancy is extremely difficult. Women with more severe asthma before pregnancy are more likely to have severe asthma, and require hospitalization, during pregnancy, although this is not always the case.18,57,147,247 No change in perinatal mortality has been reported in women with mild to moderate asthma when the asthma was medically managed; women with severe asthma are at high risk for exacerbation and pregnancy complications, including preterm delivery, low birth weight, fetal growth restriction, increased perinatal mortality and morbidity, and preeclampsia.14,57,147,171,203 Worsening of symptoms during the intrapartum period occurs in 10% to 40%; most return to their prepregnancy status by 3 months postpartum.80,171
Asthma may lead to maternal hypoxia or hyperventilation with resultant hypocapnia and alkalosis, potentially affecting fetal well-being.247 Transient hypoxia is not generally as great a concern as chronic hypoxia, which increases the risk of preterm birth, altered fetal growth, and mortality.247 Acute hypocapnia and alkalosis, however, can contribute to fetal depression by reducing umbilical and uterine blood flow secondary to vasoconstriction. Alkalosis also increases maternal hemoglobin affinity for oxygen, thereby reducing availability to the fetus.
The normal alterations of the respiratory system during pregnancy may influence asthma in both positive and negative ways (Table 10-2). The hyperventilation of pregnancy may be more distressful for the asthmatic woman.80 The increase in circulating cortisol levels may augment cAMP functioning as well as reduce inflammation through steroid action. Progesterone levels decrease bronchomotor tone—relaxing smooth muscle tissue—and thereby decrease airway resistance. Elevated serum cAMP levels may also promote bronchodilation. Thus improvement of asthma during pregnancy may be secondary to increased free cortisol, decreased plasma histamine, decreased bronchial smooth muscle tone due to progesterone, and decreased airway resistance due to decreased tone.147,171,247 Conversely, worsening may be due to increased progesterone and mineral steroids that compete for glucocorticoid receptors, increased β2-adrenoreceptor responsiveness, increased viral respiratory infections and thus bronchial inflammation, increased PGF2α, and hyperventilation.147 The increased tendency for gastroesophageal reflux (see Chapter 12) during pregnancy may also exacerbate the asthma.171 Therefore, the effect of pregnancy on the course of asthma in an individual woman depends on the balance of these factors in her system.
Table 10-2
Factors Affecting Asthma in Pregnancy
IMPROVEMENT
Increased progesterone-mediated bronchodilation
β-Adrenergic-stimulated bronchodilation
Decreased plasma histamine levels
Increased free cortisol levels
Increased glucocorticoid-mediated β-adrenergic responsiveness
Increased PGE-mediated bronchodilation
PGI2-mediated bronchial stabilization
Increased half-life of bronchodilators
Decreased protein-binding of bronchodilators
WORSENING
Pulmonary refractoriness to cortisol effects
Increased PGF2α-mediated bronchoconstriction
Decreased functional residual capacity, causing airway closure and altered ventilation-perfusion ratios
Increased major basic protein in lung
Increased incidence of viral or bacterial respiratory infection
Increased gastroesophageal reflux
Increased stress
Data from Schatz, M. & Hoffman, C. (1987). Interrelationships between asthma and pregnancy: Clinical and mechanistic considerations. Clin Rev Allergy, 5, 301.
Pharmacologic treatment and the selection of an appropriate agent are based on the risk-to-benefit ratio of bronchodilator effect and hypoxia avoidance versus possible adverse consequences.173 Oral corticosteroids in the first trimester have been linked to an increased risk of cleft lip with or without cleft palate, and increase in preeclampsia, preterm delivery, and low birth weight.57,183 Thus inhaled corticosteroids are generally advocated as a first line therapy.57,173,247 Maternal physiologic changes may alter the pharmacokinetics, although most agents seem to be as effective during pregnancy as in nonpregnant women.247 Women with exacerbations may need stepwise increase in therapies; women who improve may also need changes in treatment.173 Decreased compliance (up to ⅓) with use of inhaled corticosteroids during pregnancy is associated with an increased frequency of exacerbations.57 The National Asthma Education and Prevention Program has published guidelines for management of the pregnant asthmatic woman and notes that “it is safer for pregnant women with asthma to be treated with asthma medication than it is for them to have asthma symptoms and exacerbations.”173 General management includes careful history taking and evaluation, patient education, avoidance of known triggers, and medical therapy. Education about the medical regimen and recognition of early symptoms so that treatment may be initiated early and hypoxia prevented are essential. Women need to be counseled to use only those medications prescribed and to avoid over-the-counter medications. Prescribed medications should be taken only as directed; maternal and fetal side effects need to be explained clearly and concisely.147
Smoking
“Smoking during pregnancy is among the leading preventable causes of adverse maternal and fetal outcomes.”171 Maternal smoking increases perinatal morbidity and mortality. Smoking may also interfere with a woman’s ability to conceive.170 Risks for the pregnant woman include increased rates of spontaneous abortion, abruptio placenta, placenta previa, early or late bleeding, premature rupture of membranes, and preterm labor.22,63,147,170,171
Fetal and neonatal risks of maternal smoking include low birth weight (two- to threefold increase), preterm delivery, and fetal growth restriction.22,147,170 Infants of women who smoke on average weigh 200 g less at birth than infants of women who do not smoke.170,233 There is a dose-related effect of the number of cigarettes per day and the decrease in birth weight, especially in women who continue smoking past 32 weeks’ gestation.147,170,190 The degree of risk for low birth weight in women who smoke may be influenced by maternal genotype, especially genetic polymorphisms that affect the activity of specific enzymes needed to metabolize the various chemicals in cigarettes.238 Paternal smoking is also associated with decreased birth weight although not to the degree seen with maternal smoking.170
The exact mechanism for these complications is not completely understood, but include indirect effects on uterine blood flow and direct effects due to transfer of toxins such as nicotine, which appears to be the toxin causing the most maternal and fetal harm, across the placenta (see Chapter 7).22,170,209 Nicotine and its main metabolite, cotinine, are lipid soluble and readily crosses the placenta.170,209 Fetal levels are generally 90% of maternal levels and may be higher.171 Nicotine may also compete with nutrients for placental nutrient carriers, reducing nutrient transfer and thus fetal growth. Many of the risks seen with smokers are related to the placenta or consequences of altered placental function. This supports an etiology mediated by changes in placental structure and alterations in uteroplacental blood flow and oxygenation.147
Placentas of smokers are proportionately greater in weight (as related to fetal weight) than placentas of nonsmokers and demonstrate histologic changes, suggesting hypoxia with a compensatory hypertrophy. Women who experience chronic anemia or live at high altitudes have similar compensatory mechanisms because of the chronic intrauterine hypoxia. The placentas of smokers also have more areas of calcification, an increased incidence of fibrin deposits, an increased frequency of necrosis and inflammation in the margin, and evidence of deoxyribonucleic acid (DNA) changes.147 In addition, an increased risk of placental lesions has also been identified. These findings suggest that smoking causes some direct damage to the blood vessels of the placenta that may lead to placental underperfusion.
The risk of hypoxia is further increased by carbon monoxide, a by-product of smoking, which equilibrates between maternal and fetal blood.147 Because carbon monoxide has a higher affinity for hemoglobin than oxygen, the oxygen-carrying capacity of the blood in smokers is reduced. These effects have been demonstrated by highly elevated levels of carboxyhemoglobin in the fetus at birth. Along with this, carbon monoxide greatly increases the affinity of oxygen for hemoglobin. With increased affinity, oxygen is less readily unloaded to the fetal tissues. This, in turn, reduces fetal oxygenation further.
Children of smokers are at risk for later problems as well, including behavioral difficulties; long term learning memory; and mood, conduct, and attention-deficit disorders.22,56,170,209 Changes in the central nervous system may be due to alterations in expression of neurotransmitters in the fetus, fetal adrenergic activation leading to sympathetic nervous system dysfunction, or alterations in the serotonin system.111 Alterations in lung function have been reported with in utero exposure to smoking and often persist to late childhood and possibly longer.56 Sudden infant death syndrome and the incidence of childhood respiratory disorders, including asthma, pneumonia, and bronchitis, are also higher.56,147,170,171 Offspring of smokers also are reported to have a higher incidence of non-Hodgkin lymphoma, acute lymphoblastic leukemia, and Wilms tumor, with a dose-response relationship.56,147
Inhalation anesthesia
The use of inhalation anesthesia in pregnant women usually occurs only during emergencies. Intubation may be more difficult in the pregnant women (see “Upper Respiratory Tract Capillary Engorgement”). The effect on the maternal respiratory system is related to maternal cardiorespiratory status before induction and the type and adequacy of ventilation following induction. Light to moderate anesthesia with adequate oxygen mixing should provide no difficulties to the well-hydrated, stable, pregnant woman.21 Because of the reduced functional residual capacity and increased closing volumes during pregnancy, as well as the higher metabolic requirements, the pregnant woman is less tolerant of apnea and is at higher risk for a difficult or failed intubation.135,136 Oxygen partial pressure levels drop rapidly in these situations, leading to hypoxia, hypoxemia, and acidosis. These events not only place the mother at risk, but also jeopardize the status of the fetus.
Inhalation agents are dose and time-dependent compounds affecting the fetus directly through transplacental movement of drugs or indirectly by altering maternal homeostasis or changing uteroplacental blood flow.21,193 If fetal depression does occur, it is an indication of impaired placental blood flow, possibly due to decreased maternal cardiac output.21,43,147 Most of the time, no serious depression occurs. However, uteroplacental blood flow may be altered through several mechanisms: (1) change in perfusion pressure, (2) modification of vascular resistance, (3) alterations in uterine contractions and basal tone, and (4) interference in fetal cardiovascular function (umbilical circulation). Uterine blood flow varies directly with perfusion pressure across the uterine vascular bed (uterine arterial pressure minus uterine venous pressure) and inversely with uterine vascular resistance. The balance between perfusion pressure and vascular resistance is the primary basis for acute changes in uterine blood flow.21,141
Adverse responses or heavy anesthesia can precipitate a hazardous sequence of events. Maternal cardiac output may fall, precipitating a fall in blood pressure and an increased likelihood of maternal acidosis and decreased uterine blood flow. The result is a decreased uteroplacental blood flow with decreased nutrient supply to the fetus. Fetal heart rate and blood pressure may fall due to direct fetal cardiovascular depression (drug response) or the indirect effect of decreased uteroplacental perfusion. The decreased cardiac output and low blood pressure culminate in fetal hypoxia and acidosis, as reflected in low oxygen saturations, elevated PCO2 levels, and falling base excess. Fetal status before induction affects the severity of the response.21,141 This same sequence of events may occur with severe maternal hyperventilation. Marked reductions in maternal PCO2 reduce uteroplacental blood flow and maternal cardiac output and can lead to fetal hypoxemia and acidosis.
Summary
The maternal respiratory alterations that occur during pregnancy ensure an adequate supply of oxygen to the developing fetus and its supporting structures. These demands are increased with activity and labor and are usually compensated for without difficulty. However, subjective interpretation of labor and the pain experienced can trigger maternal hyperventilation and alter fetal homeostasis. Adequate education of the mother about the normal physiologic changes and the labor experience is essential to maternal-fetal well-being. Psychoprophylaxis, analgesia, and anesthesia can moderate the experience and can be used safely during the intrapartum period. Careful monitoring with all these methods is important to safeguard the fetus. Clinical implications for the pregnant woman and her fetus are summarized in Table 10-3.
Table 10-3
Clinical Implications for the Respiratory System in the Pregnant Woman and Fetus
Understand the normal respiratory changes that occur during pregnancy (pp. 297-301, 303-304).
Explain to the pregnant woman the changes that can occur in the respiratory system early in pregnancy and how they can affect daily activities and exercise tolerance (pp. 298-300, 303-305).
Encourage prelabor preparation to reduce discomfort, hyperventilation, and anxiety during labor (pp. 301-303).
Reduce hyperventilation during labor by counting respirations slowly, discouraging breath holding, and encouraging deep breathing between contractions (pp. 301-303).
Discuss upper airway changes that may lead to nasal congestion and other symptoms (pp. 304-305).
Counsel women regarding respiratory infections during pregnancy (pp. 305-306).
Counsel pregnant women to get an influenza vaccine if they are pregnant during flu season (p. 306).
Discuss usual exercise routines and changes that may be necessary (p. 305 and Chapter 9).
Counsel pregnant women regarding air travel (p. 305).
Counsel asthmatic women who are pregnant to follow their medical regimen as directed, to avoid known precipitating factors, and seek medical intervention when symptoms persist (pp. 305-307).
Encourage and support pregnant women in reducing or eliminating cigarette consumption both during and after pregnancy (pp. 207-308, Chapter 7).
Development of the respiratory system in the fetus
Embryonic development of the lung and the role of lung fluid and fetal breathing movements in development, as well as surfactant synthesis and secretion, set the stage for understanding the changes that occur with transition to extrauterine life. Respiratory system development is stimulated by multiple genes and a complex interplay of regulatory molecules, including growth factors, transcription factors (DNA binding proteins), extracellular matrix molecules, integrins, intracellular adhesion molecules, morphogens, and exogenous factors such as retinoic acid and antioxidants. 40,83,123,163,198,240 Lung development is also stimulated by mechanical forces especially stretch of lung tissue by fetal breathing movements and accumulation of lung fluid.38 Mechanical forces increase the rate of cell proliferation and differentiation, especially of the alveolar epithelium.90
Anatomic development
Lung growth occurs in five stages: embryonic (3 to 6 weeks’ gestation), pseudoglandular (6 to 16 weeks’ gestation), canalicular (16 to 26 weeks’ gestation), saccular (26 to 36 weeks’ gestation), and alveolar (36 weeks’ gestation to 2 to 3 years postbirth).114,129 These stages are summarized in Figure 10-5.
The embryonic stage of lung development lasts from 3 to 6 weeks’ gestation. Around day 24, a ventral diverticulum (outpouching) can be seen developing from the foregut. This groove extends downward and is gradually separated from the future esophagus by a septum (see Chapter 12). Two to 4 days later, the first dichotomous branches can be seen (Figure 10-6). By the end of this stage, 3 main divisions are evident on the right and 2 on the left, with 10 rudimentary bronchopulmonary segments on the right and 8 or 9 on the left.164,230,240 Lung bud branching is mediated by fibroblast growth factors (FGF)-1 and -2 secreted by the heart, transforming growth factor-β (TGF-β), which is controlled by retinoic acid (RA) via RAR-α and RAR-β (deletions of these receptors can lead to pulmonary agenesis, tracheal esophageal fistula, and lobar agenesis) and many other endodermal and mesenchymal factors.40,163
Between 6 and 16 weeks’ gestation, a tree of narrow tubules forms. New airway branches arise through a combination of cell multiplication and necrosis. These tubules have thick epithelial walls made of columnar or cuboidal cells. This morphologic structure, along with the loose mesenchymal tissue surrounding the tree, gives the lungs a glandular appearance (hence the term pseudoglandular stage).114,164,240 The principal pulmonary arteries are in place by 14 weeks.114 By 16 weeks, branching of the conducting portion of the tracheobronchial tree is established. These preacinar airways can from this point forward increase only in length and diameter, not in number. The most peripheral structures at this time are the terminal brochioles.240 Fifteen to 20 generations of airways develop (all branches to the level of the alveolar ducts).114,120
Epithelial-mesenchymal interaction is critical for early lung and pulmonary vasculature development and branching morphogenesis mediated by FGF-10, FGF-7, endothelial GF, and TGF-α.90,120,198,199 The mesenchymal tissue surrounding the airways has an inductive influence via expression of multiple growth and transcription factors and other signaling molecules. Removal of this tissue interrupts epithelial branching until regeneration occurs. Mesenchyme that surrounds the endodermal tree contributes to the nonepithelial elements of the bronchial tree. Another type of mesenchyme develops into the pleura, subpleural connective tissue, intralobular septa, and cartilage of the bronchi. Toward the end of the pseudoglandular period, rudimentary forms of cartilage, connective tissue, muscle, blood vessels, and lymphatic vessels can be identified.104 Ciliated cells appear in the upper airway by 7 weeks and in the bronchi by 13 weeks. Mucus-producing glands appear in the bronchi by 13 weeks.114,240 Mucus production begins by 14 weeks.114 Primitive arteries appear by 14 weeks.114
Around 16 weeks, the epithelial cells of the distal air spaces (future alveolar lining) begin to flatten (becoming more cuboidal) and increase in glycogen (which serves as a substrate for surfactant synthesis), signaling the beginning of the canalicular stage.192 This stage is called canalicular because of the vascular capillaries that begin to multiply in the interstitial space.240 A rich vascular supply begins to proliferate in the interstitial space, and with the changes in mesenchymal tissue, the capillaries are brought closer to the airway epithelium to form alveolar-capillary membranes (future gas exchange areas) by 19 weeks.114,240 Primitive respiratory bronchioles begin to form during this stage, delineating the acinus (gas-exchanging section of the lung) from the conducting portion of the lung (Figure 10-7, A). Type II and then type I alveolar epithelial cells begin to differentiate.240 Between 20 and 24 weeks, the cuboidal type II cells lining the terminal portions of the airway begin to develop lamellar bodies, indicating the beginning of surfactant production.114,120,192
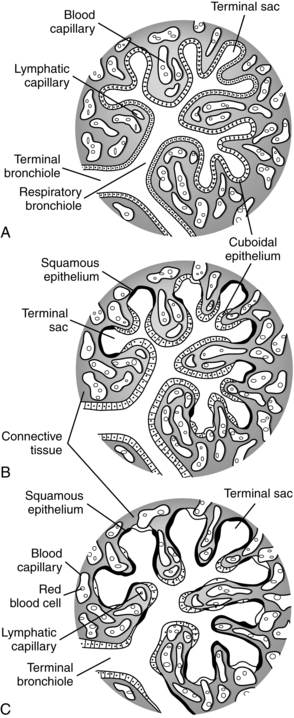
The canalicular stage continues until 26 weeks’ gestation. By 24 to 26 weeks, terminal air sacs begin to appear as outpouchings of the terminal bronchioles, marking the beginning of the saccular stage (see Figure 10-7, B). During the saccular stage the number of terminal sacs increases, forming multiple pouches off a common chamber (the future alveolar duct). Glucocorticoid receptors on these cells increase with increasing gestation.90 Around 30 weeks’ gestation, lung surface area and volume increase sharply.156,240 As blood vessels develop, they stretch and thin the epithelium that covers them, bringing the double capillary network (see Pulmonary Vasculature) into closer proximity with the developing airways (see Figure 10-7, B and C).164,240 The number of air spaces (initially saccules, then later alveoli) increase from 65,000 at 18 weeks to 240,000 by 24 weeks, 4 million by 32 to 36 weeks and 50 to 150 million by term (versus 500 million in adults).114,115,120
The alveolar stage begins around 36 weeks’ gestation (see Figure 10-7, C). Shallow cup-shaped indentations in the saccule walls can be detected. These primitive alveoli consist of smooth-walled transitional ducts and saccules with primitive septa with double capillary loops.38 These saccules will deepen and multiply via septation postnatally to form true alveoli and markedly increase the gas exchange surface area.38,240 Only about 20% of the alveoli are formed by term. Alveolarization also involves thinning of the distal airways and alveolar walls and growth of lung capillary network.6,255 These processes are stimulated by growth and can be disrupted by hypoxia or hyperoxia.120,255 The alveolar stage is also a time of microvascular maturation with further thinning of the gas exchange membrane and intraalveolar walls and formation of a single capillary network (see Pulmonary Vasculature).90,198 Disruption of lung development between 32 weeks and term interferes with alveolarization and can have both short- and long-term effects on lung function.114
The respiratory portion of the lung has a continuous epithelial lining composed mainly of two cell types: type I and type II cells (pneumocytes). These cell types begin to differentiate in the late canalicular stage.198,240 Type II cells appear first, followed by type I cells. Type I cells attenuate or flatten with maturation. These cells with their long cytoplasmic extensions cover approximately 93% of the alveolar surface by term (compared to about one third of the surface in adults).240 The thinnest area of the alveolus is composed of these extensions, and it is here that gas exchange occurs most rapidly.
The type II cells, although more numerous than type I, occupy less than 5% of the alveolar surface (versus about two thirds in adults).192,240 These cells retain their cuboidal shape and contain more organelles than do type I cells. Mitochondria are larger, and the Golgi apparatus, rough endoplasmic reticulum, ribosomes, and multivesicular bodies are more extensive. Lamellar bodies and glycogen lakes appear within the cytoplasm.240 Surfactant is produced and secreted by the lamellar bodies. With development, numbers and size of lamellar bodies increase with storage of surfactant lipids. As the density of lamellar bodies increases, cytoplasmic glycogen decreases.240
The first type II cells are seen between 20 and 24 weeks’ gestation.114 Once they appear, the number of cells increases, with a concomitant increase in the number of lamellar bodies within the cells. The organelles migrate toward the luminal plasma membrane (alveolar duct surface), which forms prominent microvilli, extending into the alveolar duct toward the end of gestation. Surfactant secretion occurs along this border.
Surfactant secretion from type II cells is detectable between 24 and 25 weeks’ gestation.99 Surfactant proteins (SPs) also begin forming. SP-A expression begins at 75% to 80% of gestation; SP-B and SP-C begin by mid-gestation.155,240 Along with surfactant production and secretion, type II pneumocytes appear to be the chief cells involved in the repair of the alveolar epithelium. This suggests that type I cells are more susceptible to injury and differentiate from type II pneumocytes.
Pulmonary vasculature
Vascular development involves two stages: vasculogenesis (development of blood vessels) and angiogenesis (“sprouting” of new blood vessels from existing ones).6 Development of the pulmonary vasculature and lymphatic system requires continuous coordinated interaction between epithelial and mesenchymal tissue.74,158,220 The relatively hypoxic uterine environment is essential for development of the lung and pulmonary vasculature. This hypoxic vasoconstriction is mediated by hypoxia inducible factor-1 (HIF-1), which regulated vascular endothelial growth factor (VEGF) and allows the pulmonary circulation to increase 60% by term without a dramatic redistribution of blood to the lungs prior to birth.74 The lung vasculature development is regulated by multiple transcription factors and other mediators. VEGF is critical both prior to and after birth. Alterations in VEGF also decrease alveolarization.158 Other factors mediating pulmonary vasculature development include angioproteins, platelet derived GF, bone morphogenetic proteins, WNT, and NOTCH signaling pathways (see Chapter 3).
Pulmonary vessel development occurs in conjunction with the branching of the bronchial tree. The arteries have more branches than the airways; the veins develop more tributaries. The preacinar region has an arterial branch (referred to as a conventional artery) that runs along each conducting airway; supernumerary arteries feed the adjacent alveoli. All the preacinar bronchi along with the pulmonary arteries are present by 16 to 17 weeks’ gestation (end of the pseudoglandular period).6,74,220 If for any reason there is a decrease in the number of airways, there is a concomitant decrease in conventional and supernumerary arteries. From 16 weeks on, the preacinar vessels increase in length and wall structure only.74,104,192,240
With movement into the canalicular and saccular stages, intraacinar arteries appear and continue their development into the postnatal period. The conventional arteries continue their development for the first 18 months of life, and the supernumerary arteries continue to be laid down for the first 8 years. These latter vessels are smaller and more numerous, servicing the alveoli directly.104 If blood flow through the conventional arteries is reduced or blocked, the supernumerary arteries may serve as collateral circulation, thereby maintaining lung function during periods of ischemia or increased pulmonary vascular resistance. Postnatally, the intraacinar vessels multiply rapidly as alveoli appear.
The pulmonary veins develop more slowly. By 20 weeks, however, preacinar veins are present. The development of the veins parallels that of the arteries and conducting airways, although supernumerary veins outnumber supernumerary arteries. Both types of veins (supernumerary and conventional) appear simultaneously.104 Formation of additional veins as well as lengthening of existing veins continues postnatally.
Further development of the pulmonary circulation is related to the changes in muscle wall thickness and extension of muscle into arterial walls. Because of the low intrauterine oxygen tension, the pulmonary artery wall is very thick. The wall thins as oxygen tension rises after birth. With thinning, the medial layer elastic fibrils become less organized. The pulmonary vein, in contrast, is found to be deficient in elastic fibers at birth and progressively incorporates muscle and elastic tissue over the first 2 years of life.99
The intrapulmonary arteries have thick walls as well. The smaller arteries have increased muscularity and dilate actively with the postnatal increase in oxygen tension. There is a concomitant fall in pulmonary vascular resistance.104 Between 3 and 28 days postnatally, these vessels achieve their adult wall thickness–to–external diameter ratio; the larger arteries take longer to achieve adult levels (4 to 18 months).99
The arteries of the fetus are more muscular than those of the adult or child. Muscle thickness–to–external diameter ratio decreases postnatally based on postnatal age and the size of the vessel.104 After delivery, muscle distribution changes, and this process continues over the first 19 to 20 years of life. Antenatally, muscle development can be seen in the arteries of the terminal bronchioles; by 4 months’ postnatal age, the arteries of the respiratory bronchioles have incorporated muscle tissue.
The pulmonary capillary network develops along with the terminal air sacs and alveoli. During the canalicular stage there is a marked increase in lung capillaries around the developing air spaces. In areas where the capillaries come into close approximation with the cuboidal epithelium, the epithelium begins to thin as initial air-blood barriers develop. 38,74,99,220 During the saccular stage, capillaries form a double layer between thick intrasaccular septa, with a capillary network on either side of a central connective tissue core.99,114,220,240 During the early alveolar stage, the intraalveolar septa attenuates and the capillary bilayer fuses into a single layer accompanied by further microvascular growth and development. This is mediated by many stimuli including VEGF, transcription factors, crosstalk between the epithelium and mesenchyme, and the low intrauterine oxygen tensions.220,237 VEGF is important in maintaining alveolar structure and development of the alveolar-epithelial capillary interface. Platelet derived GF, FGF family, collagenases, and proteoglycans are also important in alveolar development at this stage.40
Congenital anomalies of the lungs
Most lung abnormalities arise during the embryonic and pseudoglandular stages.240 Pulmonary agenesis in the embryonic period probably occurs secondary to failure of initial (lung agenesis) or later (lobar-bronchial agenesis) branching. Primary pulmonary hypoplasia is related to abnormalities in the transcription factors or GF critical for early lung development.120 Agenesis is rare, but when it occurs is often associated with tracheal stenosis or esophageal atresia.240 Lack of adequate amniotic fluid during the canalicular or saccular periods can lead to pulmonary hypoplasia.40 Pulmonary hypoplasia may arise due to intrinsic abnormalities in lung development, and is most damaging during the canalicular stage when the conducting airways are developing.114 Hypoplasia may occur secondary to external compression, as with congenital diaphragmatic hernia (CDH), or from oligohydramnios, as occurs with renal agenesis (and thus inadequate amniotic fluid production in Potter syndrome.74,120 The mechanisms for pulmonary hypoplasia with oligohydramnios (see Chapter 3) is not well understood but may be due to mechanical restriction of the chest wall (lack of space to grow due to lack of amniotic fluid in the amniotic sac), interference with fetal breathing movements, or failure to produce adequate fetal lung fluid.240 Pulmonary hypoplasia with decreased alveoli and a reduced pulmonary vascular bed can result in abnormal muscularization of preacinar and intracinar arterioles and lead to persistent pulmonary hypertension.74
CDH occurs in 1/2200 to 1/4000 live births and is due to malformations in the diaphragm with alterations in lung development.3,74 The cause of the malformation is unknown and the defect in the diaphragm may be secondary to altered lung development.3,74 Hypotheses include that the diaphragmatic defect is due to (1) abnormal development of the adjacent lung, (2) abnormal muscle innervation by the phrenic nerve, (3) improper myotubule formation, (4) failure of closure of the pleuroperitoneal canal, or (5) defective pleuroperitoneal fold development.83 CDH results in lung compression by the herniated portions of the gastrointestinal system. The lung on the side of the herniation is most severely affected, but the lung on the contralateral side is also affected. The lung hypoplasia in CDH involves a developmental as well as a compression defect, possibly due to down regulation of signaling molecules, with delayed lung maturation, altered pulmonary vasculature development and defects in surfactant maturation.6,83,90,114,138,232
Bronchiogenic cysts—usually single lesions ranging in size from small cysts to those covering an entire lobe—develop early in gestation. These cysts arise from abnormal budding of the foregut diverticulum with cystic duplication of the tracheobronchial tree.40 Congenital cystic adenomatoid malformations arise from alterations in the development of the terminal respiratory bronchioles.40,229 These defects are characterized lung tissue with a discordant spacial arrangement due to abnormal airway patterning and abnormal branching of immature bronchioles accompanied by overgrowth of terminal bronchioles and a decreased number of alveoli.40 Other events that can alter fetal and postnatal lung development include fetal growth restriction, altered fetal breathing movements, decreased nutrient supply, nicotine exposure, and preterm birth.150
Functional development
The functional development of the lung includes development of the surfactant system, defenses against inflammation and oxidative stress, lung fluid, and fetal breathing movements. The lung secretes various substances and has its own macrophage function. Macrophages are found in groups of three or four cells lying free within the alveolar space. Ingested foreign bodies are seen as osmiophilic inclusions within the cell. These cells are spherical in shape and are derived from hematopoietic tissue.157 Larger particles (e.g., bacteria) not swept away by ciliary action are removed and destroyed by pulmonary macrophages. Foreign material, once identified, is engulfed and destroyed by the macrophage. These cells are critical for protection of the lung environment and removing surfactant from the alveolar surface.
Surfactant
Surfactant is of major importance to the adequate functioning of the lung and has many roles (Table 10-4), including reduction of surface tension at the air-liquid interface within alveoli (Figure 10-8), altering lung mechanics, and is lung innate host defenses.244 Pulmonary surfactant is a lipoprotein, composed of 70% to 80% phospholipids (Figure 10-9).120,246 The majority (80%) of the lipid is saturated phosphatidylcholine (PC), of which dipalmitoyl phosphatidylcholine (DPPC) is the most abundant. The latter is the component responsible for decreasing the surface tension to almost zero when compressed at the surface during inspiration. Phosphatidylglycerol (PG) accounts for another 5% to 10% of the phospholipids present in surfactant. PG is unique to lung cells, bronchoalveolar fluid, and amniotic fluid. This makes PG a good marker for surfactant. Immature lungs contain large amounts of phosphatidylinositol PI. Levels of PI decrease as PG increases with lung maturity.114,120 Neutral lipids include primarily cholesterol along with esters and acylglycerol fatty acids.246 The other components are involved in intracellular transport, storage, exocytosis, adsorption, spreading of the monolayer, clearance at the alveolar lining, and immunoprotection (see Box 10-2 on page 315).246
Table 10-4
Classic and “Nonsurfactant” Functions of Pulmonary Surfactant
CLASSIC FUNCTIONS | “NONSURFACTANT” FUNCTIONS |
Maintaining the gas exchange area of the lung
Reduction of pulmonary shunt flow
SP-A and SP-D are “collectins” with a pathogen-recognizing function
SP-A and SP-D serve as opsonins, modulating chemotaxis, and phagocytosis
SP-A interacts with alveolar macrophages through a specific receptor
Alteration of cytokine/inflammatory mediator release
From Frerking, I., et al. (2001). Pulmonary surfactant: Functions, abnormalities and therapeutic options. Intensive Care Med, 27, 1700.
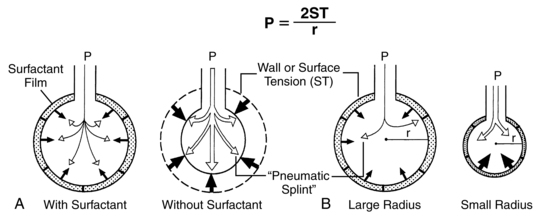
The four surfactant proteins (SPs) are SP-A, SP-B, SP-C, and SP-D. These make up 10% of the surfactant.114 SPs are involved in surfactant function, modulate alveolar macrophages, and influence innate immunity and surfactant catabolism.244 SP-A, SP-B, and SP-C are produced by Clara cells (conducting airway epithelial cells). SP-A and SP-D are hydrophilic collectins (innate host defense proteins). SP-B and SP-C are hydrophobic.143 SP-A is the most abundant SP and is needed for surfactant turnover, formation of tubular myelin (Figure 10-10 and Box 10-2 on p. 315), and nonimmune host defenses within the lungs.
SP-A maintains the surfactant monolayer at the air-liquid interface.44 The major function of SP-A is in innate host defenses in the alveoli and airways. SP-A acts as an opsonin in modifying inflammatory responses and promotes phagocytosis and clearance of pathogens by alveolar marcophages.114,120 Deficiency of SP-A increases the risk of pulmonary infection. SP-B acts with SP-A to form tubular myelin (see Figure 10-10).24,46,156,243,246 SP-A may also act to make surfactant less vulnerable to inactivation by edema and inflammatory byproducts after lung injury, in binding exotoxins and in gram negative and gram positive organism opsonization.114 SP-A levels are low in preterm infants with respiratory distress syndrome (RDS).120 Decreased SP-A to phospholipid ratios increase the risk of RDS, bronchopulmonary dysplasia (BPD), and death.114
SP-B maintains the surface tension–lowering effects of surfactant, enhances spreading of the monolayer, and stimulates lipid adhesion and surface film formation.157 SP-B is essential for production of lamellar bodies and reprocessing of surfactant.44,114,120 SP-C working with SP-B facilitates spreading, surface absorption, and lipid uptake.24,114,156,243 SP-D has a similar function to SP-A in innate host defenses and is increased with acute lung injury.24,114,156,243 SP-D is also important in surfactant structure and regulating the alveolar surfactant pool and reuptake. SP-D binds bacteria, fungi, viruses, and promotes macrophage opsonization and Phagocytosis.245
Mutations in genes for SP-A, SP-B, and SP-C and transporter proteins such as ABCA3 can lead to inborn errors in surfactant production and acute or chronic respiratory problems after birth.243,245 Mutations in the SP-B gene on the short arm of chromosome 2 result in a recessive disorder that has a neonatal onset with surfactant deficiency, respiratory failure, and increased risk of air leak and pulmonary hypertension. Complete lack of SP-B results in severe RDS that does not respond to surfactant replacement therapy. These infants need a lung transplant to survive. These infants are usually term and die within 3 to 6 months. Infants with partial or transient SP-B defects have a better outcome.41,156 SP-C mutations had a variable onset of symptoms such as tachypnea, cyanosis, and failure to thrive. ABCA3 (transport protein located in the lining of lamellar bodies) deficiency can also result in severe surfactant deficiency and a lethal form of RDS in term infants.114,245 Alterations in SP-C can lead to interstitial lung disease.114,120
Surfactant biosynthesis.
The biosynthesis of surfactant entails a series of events including glycerophospholipid production, apoprotein synthesis, glycosylation and surfactant apoprotein processing, integration of surfactant component parts, transport of components from synthesis sites to integration sites (lamellar bodies), and finally breakdown and recycling of components. This process is summarized in Box 10-2 on page 315 and in Figure 10-10.
PC and PG are composed of a three-carbon glycerol backbone with fatty acids esterified to the hydroxyl groups. The asymmetric arrangement of these molecules results in a hydrocarbon-rich fatty acid tail, which is nonpolar, and a phosphodiester region, which is polar. This allows for a monolayer film (and sometimes multilayers) to be established at the air-liquid interface within the alveoli.114,191,196,246
The increasing biosynthesis of phosphatidylcholine (PC), phosphatidylinositol (PI), and phosphatidylglycerol (PG) in late gestation depends on the biosynthesis of phosphatidic acid. PI appears by 28 weeks and peaks at 32 to 35 weeks; PG appears at 36 weeks and increases to term; PC gradually increases from 28 weeks to term, peaking after 36 weeks.88 The majority of phospholipid produced is PC. The choline pathway and the diglyceride synthesis mechanisms that yield increased PC synthesis in late gestation interact. Although this interaction yields increased quantities of PC, it is not the highly saturated version identified in the final surfactant compound. The remodeling of PC that occurs in the phosphatidylcholine-lysophosphatidylcholine cycle provides the DPPC required for surfactant.156,196
As gestation advances, phospholipid content and saturation increase. This is accompanied by an increase in lamellar bodies within the type II cells. Choline incorporation, which is low in early gestation, increases abruptly in late gestation. This suggests that pathway regulatory mechanisms are enhanced in order to meet postnatal needs. Increases in surfactant before birth are due to increased synthesis; increases during and immediately after birth are due to increased secretion.196 In late gestation in term infants, the surfactant pool increases to levels similar to those in adults. Table 10-5 summarizes differences in surfactant components in mature versus immature lungs. Markers of lung maturation include loss of glycogen lakes in type II cells, increased fatty acid synthesis, increased β-receptors, and increased production of PC via the choline incorporation pathway.156
Table 10-5
Changes in Surfactant with Development
VARIABLES | IMMATURE LUNG | MATURE LUNG |
TYPE II CELLS | ||
Glycogen lakes | High | None |
Lamellar bodies | Few | Many |
Microvilli | Few | Many |
SURFACTANT COMPOSITION | ||
Sat PC/Total PC | 0.6 | 0.7 |
Phosphatidylglycerol (%) | <1 | 10 |
Phosphatidylinositol (%) | 10 | 2 |
SP-A (%) | Low | 5 |
SURFACTANT FUNCTION | ||
Decreased | Normal |
Sat PC, Saturated phosphatidylcholine; SP-A, surfactant protein A.
From Jobe, A.H. (2006). Lung development and maturation. In R.J. Martin, A.A. Fanaroff, & M.C. Walsh (Eds.). Neonatal-perinatal medicine: Diseases of the fetus and infant (8th ed.). Philadelphia: Mosby, p. 1076.
Enzymatic changes in the phospholipid synthesis pathway correlate to the surge in saturated PC and increase in PG with concomitant decrease in PI.156,196 Whether this surge is due to a change in enzyme or substrate concentration, an adjustment in catalytic efficiency, a change in substrate affinity, or the activation of latent enzymes is not clear.
Influences on fetal lung maturation
A complex interaction of hormones, growth factors, and other substances controls surfactant synthesis. Normal lung function is dependent upon the presence of surfactant, which permits a decrease in surface tension at end-expiration (to prevent atelectasis) and an increase in surface tension during lung expansion (to facilitate elastic recoil on inspiration). Surfactant provides the lung with the stability required for maintenance of homeostatic blood gas pressures while decreasing the work of breathing.82 Surfactant may play a role in the cascade of events that leads to the initiation of labor and parturition. Secretion of surfactant phospholipids into amniotic fluid with fetal breathing during the third trimester may stimulate PGE synthesis in the amnion by serving as a source of arachadonic acid. SP-A, which is secreted after 80% of gestation and reaches maximal levels right before term, may serve as a stimulus for activation of inflammatory signals that eventually lead to labor onset.116,120
Hormones that stimulate or accelerate lung maturity include glucocorticoids, thyroid hormones, estradiol, vascular epithelial growth factor, fibroblast growth factor, prolactin, thyrotropin-releasing hormone, catecholamines, TGF-α, and epidermal growth factor.143,196 Estradiol stimulates phospholipid synthesis and helps prepare the pulmonary circuit for the decreased pulmonary vascular resistance after birth.213 Thyroxine (T4) and triiodothyronine (T3) have also been shown to increase the rate of phospholipid synthesis.196 Thyroid hormones, like glucocorticoids, enhance production of PC through choline incorporation. They do not, however, increase PG synthesis or stimulate the production of surfactant-specific proteins. Whereas glucocorticoids increase fatty acid synthetase activity, thyroid hormone seems to decrease its activity. These differences suggest different sites of action for these hormones as well as the need for action in conjunction with other hormones. However, trials of thyrotropin-releasing hormone either alone or in combination with glucocorticoids have demonstrated a lack of efficacy and an increase in complications.41
Glucocorticoids and thyroid hormones play a role in enhancing the synthesis of phospholipids; catecholamines stimulate the secretion of surfactant into the alveolar space. This appears to be a direct action of adrenergic compounds on type II cells. The increase in surfactant and saturated phosphatidylcholine in lung fluid improve lung stability. An added benefit is the inhibition of fetal lung fluid secretion and possible reabsorption of the fluid within the alveoli at the time of delivery. These two effects (the increase in surfactant and decrease in lung fluid) work together in preparing for respiratory conversion at birth.71 Other factors influencing type II cell maturation and surfactant biosynthesis are summarized in Table 10-6.
Table 10-6
Regulators of Alveolar Type II Cell Differentiation
AGENT | PROPOSED PHYSIOLOGIC ROLE |
INDUCERS | |
Glucocorticoid (cortisol) | Major endogenous modulator of alveolar development and surfactant production |
β-Adrenergic agonists (especially epinephrine, cyclic AMP) | Increase surfactant production and secretion, especially during labor and delivery |
Thyroid hormones (T3, T4) | Enhance glucocorticoid effects on lipid synthesis |
Retinoic acid | May interact with glucocorticoids to regulate lipid and SP-B production |
Bombesin-related peptides | May contribute to surfactant lipid synthesis |
Parathyroid hormone-related protein | ? |
INHIBITORS | |
Protein kinase C activators (proinflammatory cytokines) | Inhibit surfactant protein gene transcription during infections, inflammation |
Transforming growth factor-β family | Inhibit type II maturation during early gestation and with inflammation |
Tumor necrosis factor-α | Inhibit SP-B and SP-C gene transcription during infection |
Insulin | Inhibit surfactant protein gene transcription in infants of diabetic mothers with poorly controlled disease |
Dihydrotestosterone | Delayed type II cell maturation in males |
From Guttentag, S. & Ballard, P.L. (2004). Lung development: Embryo growth, maturation and developmental biology. In H.W. Tausch, R.A. Ballard, & C.A. Gleason (Eds.). Avery’s diseases of the newborn (8th ed.). Philadelphia: Saunders, p. 610.
Glucocorticoids.
Glucocorticoids are critical for the normal pattern of surfactant synthesis and fetal lung maturation.91,196 Glucocorticoids bind to gene promoter regions and induce lipogenic enzymes needed for phospholipid production and desaturation of PC, an induce enzymes for Na and K flux.156 Glucocorticoids increase the rate of glycogen depletion and glycerophospholipid biosynthesis. The depletion in glycogen leads to direct anatomic changes in alveolar structures by thinning the interalveolar septa and increasing the size of the alveoli. Morphologic changes include an increase in the number of type II cells and an increase in the number of lamellar bodies within those cells. These changes occur in conjunction with functional maturation, leading to an accelerated synthesis of surfactant phospholipid.24,91,156 Glucocorticoids may also play a role in early lung development.24 Glucocorticoids act directly on lung tissue, increasing the number of β-adrenergic receptors and enhancing elastin and collagen production, thus improving lung compliance.156,196 Glucocorticoids also increase antioxidant enzymes to assist in protecting cells during the transition from the low oxygen intrauterine to high oxygen extrauterine environment, enhance lung fluid clearance and lung parenchyma maturation.89,156 Levels of glucocorticoids increase near term enhancing lung maturation by increasing fatty acids needed for phospholipid synthesis, and increasing expression of SP-B, SP-C, and SP-D (primarily by increasing transcription of genes that code for these proteins).89,143 The actions of glucocorticoids may be mediated by the expression of 11β-hydroxysteroid dehydrogenase (see Chapter 19).75
Antenatal corticosteroids.
Administration of antenatal corticosteroids (ACS) can accelerate normal lung maturation both morphologically and functionally as described above, inducing structural maturation, and increasing lung volume, compliance, and responses to exogenous surfactant therapy.94 Current ACOG recommendations are: “A single course of corticosteroids is recommended for pregnant women between 24 weeks’ and 34 weeks’ gestation who are at risk of preterm delivery within 7 days. A single course of antenatal corticosteroids should be administered to women with premature rupture of membranes before 32 weeks’ gestation to reduce the risks of respiratory distress syndrome, perinatal mortality, and other morbidities. The efficacy of corticosteroid use at 32 to 33 completed weeks’ gestation for preterm premature rupture of membranes is unclear, but treatment may be beneficial, particularly if pulmonary immaturity is documented.”5,p.422 Either betamethasone or dexamethasone may be used, with no clear proof of efficacy of one drug over the other. Some studies suggest that betamethasone may be safer and more protective, but this has not been found in others.174,242 ACS treatment reduces the incidence of RDS by about 50% in infants younger than 31 weeks’ gestational age, decreases neonatal mortality by 30%, and decreases the incidence of intraventricular hemorrhage, necrotizing enterocolitis and the severity of disease in infants with RDS.20,91,93,120,143,186 Concerns have been raised about the effects of corticosteroids on the developing brain and fetal growth with repeated courses of ACS.5,13,20,127,175,186,242 Multiple courses may decrease the risk of RDS, but they also increase the risk of fetal growth alterations.13,20,42,93,178,186,235 However, a recent study reported that a single repeat dose (>7 days) was not associated with differences in physical growth or neurodevelopment at 2 years.185 Another recent study found that multiple courses (2 to 9) were associated with a dose-dependent decline in fetal growth.178 ACOG recommends that a “single rescue course of antenatal corticosteroids may be considered if the antecedent treatment was given more than 2 weeks prior, the gestational age is less than 32 6/7 weeks, and the women are judged by the clinician to be likely to give birth within the next week. However, regularly scheduled repeat courses or multiple courses (more than two) are not recommended. Further research regarding the risks and benefits, optimal dose, and timing of a single rescue course of steroid treatment is needed.”5,p.422
Factors enhancing and delaying lung maturation.
In addition to the use of antenatal steroids, other factors may also influence fetal lung maturation. Maternal-fetal events that lead to stress, and increased fetal catecholamine and corticosteroid levels have been associated with accelerated lung maturity and a decreased risk of RDS.156 However, stressed infants do not consistently have a lower incidence of RDS. Maturation of lung function is variable. Although lung maturation usually occurs after 35 to 36 weeks, lung maturation may occur earlier or later. Some infants seem to have spontaneous early lung maturation. Lung maturation can be induced as early as 24 weeks by antenatal corticosteroids or exposure to inflammation.116,120 About 20% of infants born <28 weeks have low oxygen needs with no or minimal RDS.140 Some infants born at 24-25 weeks can be transitioned to air breathing with continuous positive airway pressure (CPAP) or maintained on CPAP from birth.10,165
Lung maturation is delayed in infants of diabetic mothers (IDMs) and infants with RH isoimmunization with hydrops. The major causative factor influencing pulmonary maturation in IDMs seems to be adequacy of blood glucose control.156 Hyperglycemia and hyperinsulinemia decrease choline incorporation into PC; hyperinsulinemia delays PC production by the type II cell and delays morphologic maturation of the lung.156 Maturation of surfactant synthesis occurs at the same time that glycogen is depleted from the lungs and liver. Insulin inhibits glycogen breakdown, thereby decreasing the substrate available for PC synthesis as well as altering the natural anatomic changes that occur with glycogen depletion. The effect of cortisol on choline incorporation may be altered by insulin, although some have found either no effect or a synergistic effect. Insulin may inhibit surfactant protein gene transcription in women with poorly controlled diabetes.69 However, in vivo studies suggest that hyperglycemia rather than hyperinsulinemia may be a more important cause of altered lung maturation in an IDM.196
Androgens also delay type II cell maturation.69 At any stage of gestation, the female fetal lung is about 1 week more mature. This may be why male preterm infants are more prone to RDS than female infants of similar gestations.196
Assessment of fetal lung maturity
Accurate assessment of fetal lung maturity is important in the management of preterm labor and birth. This is especially true when the date of the last menstrual period is not known or if preeclampsia, placenta previa, multiple pregnancy, Rh isoimmunization, diabetes, or fetal growth restriction is present. The presence of surfactant phospholipids in the amniotic fluid allows determination of lung maturity because their concentrations change as the surfactant system matures. Gluck and colleagues initially demonstrated that the ratio of lecithin (L) to sphingomyelin (S) (the L/S ratio) is a reliable index and a good predictor of whether RDS will develop if the infant is born within the 48 hours following amniocentesis.156 Sphingomyelin is a membrane lipid not related to lung maturation that remains relatively stable. Lecithin levels rise sharply at around 34 to 35 weeks’ gestation, thereby providing the basis for achieving a ratio between the two. An L/S ratio greater than 2:1 indicates lung maturity and a negligible risk for RDS. Values less than 1.5:1 to 2:1 indicate lung immaturity, with a high incidence of RDS if the ratio is <1:1.120 A higher incidence of inaccurate readings is found in complicated pregnancies.
Factors that influence phospholipid concentrations in the amniotic fluid include volume of amniotic fluid (varies inversely) and contamination of the sample with blood, meconium, or antiseptics. PC levels are falsely high with blood contamination (fetal or maternal). PG is only slightly affected, however, because blood does not contain PG in significant amounts. The presence of PG is an indication that the major synthesis pathway for surfactant is present and functioning. Therefore, when PG is present in the amniotic fluid, there is little risk for RDS regardless of the L/S ratio value.156 Other techniques for assessing fetal lung maturity include the complete lung profile, a modified profile (L/S ratio and PG only), and techniques such as the fluorescent polarization (FP) assay, lamellar body count, surfactant to albumin ratio, and foam stability.88,156
Antioxidant defenses
The placenta contains all major antioxidant systems.29,49,172 Oxidative stress is a part of the normal process of early development of the fetus and placenta (see Chapter 3). For example, low levels of oxidative stress are needed for placental angiogenesis and to establish blood flow in the intervillous space. Initial embryologic development occurs in a low oxygen environment. The onset of full intervillous space blood flow (and thus increased exposure to reactive oxygen species) occurs at the same time that maternal, placental, and fetal AO systems increase. Production of reactive oxygen species may be important in signaling during pregnancy, stimulating labor onset and enhancing myometrial contractility. Excess oxidative stress, however, can increase the risk of miscarriage. In addition, oxidative stress is increased with preeclampsia, diabetes, and fetal growth restriction.29,172
The transition to the extrauterine environment is a move from a relatively hypoxic state to a more hyperoxic one. In utero, the fetal lung is exposed to low PaO2 levels, with even lower oxygen tensions existing in the fetal lung fluid that fills the alveolar spaces and bronchiole tubes. At delivery the alveolar and airway cells are abruptly exposed to hyperoxic tensions. Therefore it is important to have an AOS in place that can reduce damage by oxygen radicals. Concerns about oxidative stress is one of the bases for studies of the use of room air or low oxygen levels rather than 100% oxygen for delivery room resuscitation.39,124,184,187,226,235
The development of the antioxidant enzymes occurs in the second half of gestation. For example, activity of superoxide dismutase, the major antioxidant enzyme, appears around the time that surfactant synthesis begins in the type II cells and continues to mature to term.132 Thus the preterm infant is particularly vulnerable to lung injury from oxidative stress.255 Antioxidant levels appear to be strongly correlated to the degree of protection that can be expected from oxygen radical–induced lung injury.11 When enzyme levels are increased (as with exogenous treatment), there is increased tolerance to hyperoxia. Although there are other protective mechanisms in place, it does not appear that increased amounts of these will result in an increased level of protection. However, deficiencies within these other pathways will result in an increased susceptibility to cellular damage. This suggests that the other antioxidants play a secondary role in providing protection. Antioxidant enzymes provide primary protection by direct detoxification of reactive oxygen radicals. This prevents damage to cell proteins and DNA structure. The other systems terminate reactions already initiated by oxygen radical attack.
Lung fluid
Fluid is present in the lung lumen as early as 6 weeks’ gestation and is actively secreted beginning in the canalicular stage.74,181 This fluid is derived from alveolar epithelium secretions and is not an ultrafiltrate or mixture of plasma or amniotic fluid.30 Lung fluid is thought to be secreted primarily by type I and II alveolar cells.126Active transport is required to attain the final ion concentrations found in the fluid; this is achieved by the active transport of chloride, with sodium following passively.74,126 The water flux can be attributed to the osmotic force of sodium chloride (NaCl).30 Osmolarity and sodium and chloride levels are higher in lung fluid than in amniotic fluid, whereas pH, bicarbonate, potassium, and protein levels are lower.74,114,126
Estimated lung fluid production in term infants is 4 to 5 mL/kg/hr or about 300 to 400 mL per day for the average infant and is slightly greater than the functional residual capacity (FRC) after birth.74,114,120 Of the fluid produced, some is swallowed and a small amount moves into the amniotic fluid via periodic opening of the larynx. Most remains in the lungs due to the higher pressure within the fetal thoracic cavity compared to amniotic fluid.114 Movement of lung fluid into amniotic fluid increases with fetal breathing movements, but these movements do not alter lung fluid production rate.120,240 The amount of lung fluid contributed to the amniotic pool is relatively insignificant when compared to that contributed by micturition (see Chapter 3).
Lung fluid plays an important part in cell maturation and development as well as in determining formation, size, and shape of the developing air space. Alterations in fluid dynamics affect pulmonary cell proliferation and differentiation. If production of lung fluid is decreased or if significant chronic leakage of amniotic fluid is experienced (both of which lead to oligohydramnios), there is risk of lung hypoplasia.74,126,181 In comparison, excessive lung fluid or tracheal obstruction of a chronic nature leads to hyperplasia, with an increase in the number of alveoli, although these alveoli are functionally immature.126,240
Lung fluid production slows in late pregnancy, with a decrease in volume to 65% of previous values. Absorption of lung fluid begins in early labor so that after birth only about 35% of the original lung fluid volume needs to be cleared (see Transitional Events).114,156,192 Infants born by cesarean delivery prior to labor onset will have a greater volume of lung fluid to clear at birth, increasing the risk of transient tachypnea of the newborn. Preterm infants also have more lung fluid at birth (up to 25% greater than term infants).126
Fetal blood gases
Usual fetal blood gases are listed in Table 10-7. Factors that affect maternal-to-fetal diffusion of oxygen include maternal and fetal PO2 relationships, maternal and fetal oxygen-hemoglobin dissociation curves (see Box 10-1 on page 301), HbF concentrations, and the Bohr effect.159 Fetal PO2 is lower (20 to 30 mm Hg) than after birth, but the oxygen content is only slightly below that of adults due to the increased affinity of fetal hemoglobin for oxygen (see Chapter 8) and lower oxygen demands due to decreased metabolic needs.81 Carbon dioxide transfer is affected by hydrogen ion concentration and the Haldane effect. Fetal blood gases and evaluation of fetal acid-base status are described in Chapter 6.
Table 10-7
Normal Fetal pH and Blood Gas Data
UMBILICAL VEIN | DESCENDING AORTA | ASCENDING AORTA | |
pH | 7.40-7.43 | 7.36-7.39 | 7.37-7.40 |
PO2 (torr) [kPa] | 28-32 [3.72-4.25] | 20-23 [2.66-3.05] | 21-25 [2.79-3.32] |
PCO2 (torr) [kPa] | 38-42 [5.05-5.58] | 43-48 [5.71-6.38] | 41-45 [5.45-5.98] |
From Fineman, J.R., Clyman, R., & Heymann, M.A. (2004). Fetal cardiovascular physiology. In R.K. Creasy, R. Resnik, & J.D. Iams (Eds.). Maternal-fetal medicine: Principles and practice (5th ed.). Philadelphia: Saunders, p. 172.
Fetal breathing movements
Fetal breathing movement (FBM) are rhythmic diaphragmatic, laryngeal, and intercostal muscle contractions.47 FBM can be seen on ultrasound as early as 10 weeks’ gestation, and they occur 6% of the time by 19 weeks.38,90,194 FBM are rapid and irregular, occurring intermittently early in gestation. These early movements probably originate in the spinal cord.1 Later movements require differentiation of the respiratory motor neurons and functional innervation of these neurons.87 As gestation progresses, the strength and frequency of FBM increase, and they occur approximately 30% to 40% of the time in the last 10 weeks, with a rate of 30 to 70 breaths per minute.9,48,162,194 FBM are associated with fetal state and tend to occur in rapid eye movement-like sleep. Large movements (gasping) occur 5% of the total breathing time, one to four times per minute. FBM decrease in frequency immediately before term labor onset.194
The rapid and irregular respiratory activity contributes to lung fluid regulation and stretches the lung tissues, thereby influencing lung growth.1,74,90 The diaphragm seems to be the major structure involved, with minimal chest wall excursion encountered (4- to 8-mm change in transverse diameter). Movement of the diaphragm is necessary for chest wall muscle and diaphragm training and development, building adequate strength for the initial breath.48 The movement of the diaphragm also influences the course of lung cell differentiation and proliferation. Bilateral phrenectomy in animal models results in altered lung morphology with an increase in type II over type I cells. Presumably the innervated diaphragm increases the size of the thorax and thereby increases tissue stress, affecting morphology.
With increasing gestational age, FBM become more organized and vigorous.162 Even with these gestational changes, tracheal fluid shifts are negligible—the pressure generated being no more than 25 mmHg (3.32 kPaa). Fetal maturation leads to the appearance of FBM cycles, with an increase in breathing movements during daytime hours. Although the patterns of FBM vary according to rate, amplitude, and character, they seem to be correlated mostly with fetal behavioral states and occur most often during rapid eye movement sleep.1,9,48,194
The mechanism for the initiation of FBM is unknown, although they can be stimulated by maternal inhalation of carbon dioxide, adrenergic and cholinergic compounds, and PG synthesis inhibitors. Other factors affecting FBM, including maternal glycemia, nicotine use, alcohol ingestion, and labor, are summarized in Table 10-8.47,157,194 These Altered breathing patterns can be seen during periods of fetal hypoxia. Mild hypoxemia decreases the incidence of FBM, while severe hypoxemia may lead to cessation of FBM for several hours. The onset of asphyxia leads to gasping.9 The onset of mild hypoxemia (as with umbilical artery occlusion of short duration) may lead to quiet sleep, which decreases activity expenditure and oxygen consumption in the fetus. Although paradoxic in nature, this conservation mechanism may protect the fetus while cardiac output is redistributed toward the placenta.
Table 10-8
Factors Affecting Fetal Breathing Movements
FACTOR | EFFECT |
Glucose concentrations; glucose infusions | Increased FBM in patients who have fasted |
Cigarette smoking | Increased frequency of FBM, although incidence remains unchanged |
Caffeine | Increased FBM with chronic exposure; no effect with acute exposure |
Ethanol | Dramatic decrease in FBM |
Methadone | Decreased FBM with chronic exposure |
Tocolytics | Dramatic increase in FBM |
FBM, Fetal breathing movements.
Adapted from Richardson, B.S. & Gagnon, R. (2004). Fetal breathing and body movements. In R.K. Creasy, R. Resnik, & J.D. Iams (Eds.). Maternal-fetal medicine: Principles and practice (5th ed.). Philadelphia: Saunders, p. 184.