Chapter 65 Respiratory Distress and Failure
The term respiratory distress is often used to indicate signs and symptoms of abnormal respiratory pattern. A child with nasal flaring, tachypnea, chest wall retractions, stridor, grunting, dyspnea, and wheezing is often judged as having respiratory distress. The magnitude of these findings is used to judge the clinical severity of respiratory distress. Although nasal flaring is a nonspecific sign, the other signs may be useful in localizing the site of pathology (Chapter 365). Respiratory failure is defined as inability of the lungs to provide sufficient oxygen (hypoxic respiratory failure) or remove carbon dioxide (ventilatory failure) to meet metabolic demands. Whereas respiratory distress is a clinical impression, the diagnosis of respiratory failure indicates inadequacy of oxygenation or ventilation or both. Respiratory distress can occur in patients without respiratory disease, and respiratory failure can occur in patients without respiratory distress.
Respiratory Distress
Respiratory Disease Manifesting as Respiratory Distress
Clinical examination is important in localizing the site of pathology (Chapter 365). Extrathoracic airway obstruction occurs anywhere above the thoracic inlet. Inspiratory stridor, suprasternal, chest wall, and subcostal retractions, and prolongation of inspiration are hallmarks of extrathoracic airway obstruction. By comparison, features of intrathoracic airway obstruction are prolongation of expiration and expiratory wheezing. Typical manifestations of alveolar interstitial pathology are rapid, shallow respirations, chest wall retractions, and grunting. The site of pathology can be localized and the differential diagnosis established on the basis of the clinical signs and symptoms (Tables 65-1 and 65-2).
Table 65-2 EXAMPLES OF ANATOMIC SITES OF LESIONS CAUSING RESPIRATORY FAILURE
LUNG | RESPIRATORY PUMP |
---|---|
CENTRAL AIRWAY OBSTRUCTION | THORACIC CAGE |
ARDS, acute respiratory distress syndrome; CNS, central nervous system.
Respiratory Distress without Respiratory Disease
Although respiratory distress most commonly results from diseases of lungs, airways, and chest wall, pathology in other organ systems can manifest as “respiratory distress” and lead to misdiagnosis and inappropriate management (Table 65-3). Respiratory distress resulting from heart failure or diabetic ketoacidosis may be misdiagnosed as asthma and improperly treated with albuterol, resulting in worsened hemodynamic state or ketoacidosis.
EXAMPLE(S) | MECHANISM(S) | |
---|---|---|
Cardiovascular |
Cardiovascular Disease Manifesting as Respiratory Distress
A child with cardiovascular pathology may present with respiratory distress caused by 2 mechanisms: (1) decreased lung compliance and (2) cardiogenic shock (Table 65-4). Diseases that result in an increased pulmonary arterial blood flow (e.g., left-to-right shunts) or increased pulmonary venous pressure (e.g., left ventricular dysfunction from hypertension or myocarditis, obstructed total anomalous pulmonary venous return) cause an increase in pulmonary capillary pressure and transudation of fluid into the pulmonary interstitium and alveoli. The increased pulmonary blood and water content leads to decreased lung compliance and results in rapid shallow respirations.
Neurologic Disease Manifesting as Respiratory Distress
CNS dysfunction can lead to alterations in respiratory patterns. Increased intracranial pressure (ICP) may manifest as respiratory distress. Early rise in ICP results in stimulation of respiratory centers, leading to increases in the rate (tachypnea) and depth (hyperpnea) of respiration. The resultant decrease in PaCO2 and elevation of cerebrospinal fluid pH lead to cerebral vasoconstriction and amelioration of intracranial hypertension. Cerebral hemispheric and midbrain lesions often result in hyperpnea as well as tachypnea. In such situations, blood gas measurements typically show respiratory alkalosis without hypoxemia. Pathology affecting the pons and medulla manifests as irregular breathing patterns such as apneustic breathing (prolonged inspiration with brief expiratory periods), Cheyne-Stokes breathing (alternate periods of rapid and slow breathing), and irregular, ineffective breathing or apnea. Level of consciousness is most often impaired when abnormal breathing pattern from a brainstem disorder is present. Along with respiratory changes, other manifestations of CNS dysfunction and increased ICP may be present, such as focal neurologic signs, pupillary changes, hypertension, and bradycardia (Chapter 63). Occasionally, severe CNS dysfunction can result in neurogenic pulmonary edema (NPE) and respiratory distress, which may be due to excessive sympathetic discharge resulting in increased pulmonary venous hydrostatic pressure as well as increased pulmonary capillary permeability. Central neurogenic hyperventilation is characteristically observed in CNS involvement by illnesses such as Reye syndrome and encephalitis. Bradycardia and apnea may be due to CNS-depressant medications, poisoning, prolonged hypoxia, trauma, or infection (see Table 65-2).
Respiratory Failure
Respiratory failure occurs when oxygenation and ventilation are insufficient to meet the metabolic demands of the body. Respiratory failure may result from an abnormality in (1) lung and airways, (2) chest wall and muscles of respiration, or (3) central and peripheral chemoreceptors (Fig. 65-1). Clinical manifestations depend largely on the site of pathology. Although respiratory failure is traditionally defined as respiratory dysfunction resulting in PaO2 < 60 torr with breathing of room air and PaCO2 > 50 torr resulting in acidosis, the patient’s general state, respiratory effort, and potential for impending exhaustion are more important indicators than blood gas values.
Acute lung injury due to pneumonia, sepsis, aspiration, drowning, embolism, trauma, smoke inhalation, or drug overdose often leads to the acute respiratory distress syndrome (Table 65-5; Fig. 65-2).
Table 65-5 SIMPLIFIED CONSENSUS DEFINITION OF ACUTE LUNG INJURY
From Wheeler AP, Bernard GR: Acute lung injury and the acute respiratory distress syndrome: a clinical review, Lancet 369:1553–1564, 2007.
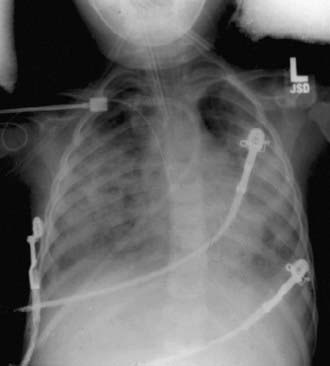
Figure 65-2 Frontal portal chest radiograph showing diffuse bilateral infiltrates consistent with acute lung injury.
(From Wheeler AP, Bernard GR: Acute lung injury and the acute respiratory distress syndrome: a clinical review, Lancet 369:1553–1564, 2007.)
Pathophysiology of Respiratory Failure
Ventilation-Perfusion Mismatch, Venous Admixture, Intrapulmonary Shunt
Normal VD/VT is around 0.33. VD/VT increases in states that result in decreased pulmonary perfusion, such as pulmonary hypertension, hypovolemia, and decreased cardiac output. Venous admixture and intrapulmonary shunting predominantly affect oxygenation, resulting in a PAO2-PaO2 (A-aO2) gradient without elevation in PaCO2. The reason is the greater ventilation of perfused areas, which is sufficient to normalize PaCO2 but not PaO2 because of their respective dissociation curves (Chapter 365). The relative straight-line relationship of hemoglobin-CO2 dissociation allows for averaging of PCO2 from hyperventilated and hypoventilated areas. Because the association between oxygen tension and hemoglobin saturation plateaus with increasing PaO2, the decreased hemoglobin-O2 saturation in poorly ventilated areas cannot be compensated for by well-ventilated areas where hemoglobin-O2 saturation has already reached near-maximum. This results in decreased SaO2 and PaO2. Elevation of PaCO2 in such situations is indicative of attendant alveolar hypoventilation. Examples of diseases leading to venous admixture include asthma and aspiration pneumonia, and those of intrapulmonary shunt include lobar pneumonia and acute respiratory distress syndrome.
Monitoring a Child in Respiratory Distress and Respiratory Failure
Clinical Examination
Clinical observation is the most important component of monitoring. The presence and magnitude of abnormal clinical findings, their progression with time, and their temporal relation to therapeutic interventions serve as guides to diagnosis and management (Chapter 365). The child with respiratory distress or failure should be observed in the position of greatest comfort and in the least threatening environment.
Pulse oximetry is the most commonly utilized technique to monitor oxygenation. Noninvasive and safe, it is the standard of care in bedside monitoring of children during transport, procedural sedation, surgery, and critical illness. It indirectly measures arterial hemoglobin-O2 saturation by differentiating oxyhemoglobin from deoxygenated hemoglobin using their respective light absorption at wavelengths of 660 nm (red) and 940 nm (infrared). A pulsatile circulation is required to enable detection of oxygenated blood entering the capillary bed. Percentage of oxyhemoglobin is reported as arterial oxyhemoglobin saturation (SaO2); however, the correct description is oxyhemoglobin saturation as measured by pulse oximetry (SpO2). This is because SpO2 may not reflect SaO2 in certain situations. It is important to be familiar with the hemoglobin-O2 dissociation curve (Chapter 365) in order to estimate PaO2 at a given oxyhemoglobin saturation. Because of the shape of the hemoglobin-O2 dissociation curve, changes in PaO2 above 70 torr are not readily identified by pulse oximetry. Also, at the same PaO2 level, there may be a significant change in SpO2 at a different blood pH value. In most situations, an SpO2 value greater than 95% is a reasonable goal, especially in emergency situations. There are exceptions, such as in patients with single ventricle cardiac lesions, in whom the pulmonary and systemic circulations are receiving blood flow from the same ventricle (e.g., after Norwood procedure for hypoplastic left heart syndrome), or with large left-to-right shunts (e.g., ventriculoseptal defect [VSD] and patent ductus arteriosus). In these types of pathophysiologic situations, a lower SpO2 is desired to avoid excessive blood flow to the lungs and pulmonary edema from the pulmonary vasodilatory effects of oxygen, and, in the patient with a single ventricle, diverting blood flow away from the systemic circulation. Because pulse oximetry recognizes all types of hemoglobin as either oxyhemoglobin or deoxygenated hemoglobin, it provides inaccurate information in the presence of carboxyhemoglobin and methemoglobin. Percentage of oxyhemoglobin is overestimated in carbon monoxide poisoning and methemoglobinemia. It should be recognized that dangerous levels of hypercarbia may exist in patients with ventilatory failure, who have satisfactory SpO2 if they are receiving supplemental oxygen. Pulse oximetry should not be the only monitoring method in patients with primary ventilatory failure, such as neuromuscular weakness and CNS depression. It is also unreliable in patients with poor perfusion and poor pulsatile flow to the extremities. Despite these limitations, pulse oximetry is a noninvasive, easily applicable, and effective means of evaluating the percentage of oxyhemoglobin in most patients.
Blood Gas Abnormalities in Respiratory Distress and Respiratory Failure
(See Chapters 52.7 and 365.)
Assessment of Oxygenation and Ventilation Deficits


Management
Endotracheal Intubation and Mechanical Ventilation
Average values for age, size, and depth of insertion for tracheal tubes are given in Table 65-6. Preoxygenation of the patient with high fractions of inspired oxygen is essential and will allow maximum procedure time prior to the onset of hypoxemia.
Although intubation can be accomplished without sedation and pharmacologic paralysis in selected patients, the physiologic benefits of these measures to the patient as well as to the facilitation of the intubation usually far outweigh the risks; sedation and paralysis should be considered standard unless contraindicated. Administration of a sedative and analgesic followed by a paralytic agent is a common pharmacologic regimen for facilitating intubation. The particular type and dose of each agent often depends on the underlying disease and clinician preference. Commonly used agents are listed in Table 65-7. An alternative to this pharmacologic approach, especially when intubation is urgent or the patient is suspected of having a full stomach, increasing the risk of aspiration, is rapid sequence intubation (Chapter 62).
Once adequate sedation and/or paralysis has been achieved, ventilation should be assisted with a bag-mask device. After optimal preoxygenation, intubation can be performed. The clinician uses his/her dominant hand to open the patient’s mouth and inserts the laryngoscope blade gently along the tongue to its base. The airway opening can be visualized by applying lift up and away from the clinician, along the axis of the laryngoscope handle. If a straight (Miller) laryngoscope blade is used, the epiglottis is lifted anteriorly by the tip of the blade to visualize the glottis. If a curved (Macintosh) blade is used, the tip should be advanced into the vallecula and then lifted to visualize the glottis. Secretions often obscure visualizations at this step and should be suctioned clear. Once clear visualization of the vocal cords is accomplished, the tube can be placed through the cords. Rapid confirmation of tube placement is essential and should be assessed by as many of the flowing steps as possible: Auscultation of both lung fields as well as the epigastrium for equal breath sounds and good air movement and evaluation of the abdomen for increasing distention should be performed. Adequate bilateral chest expansion and misting inside the tracheal tube with each breath are suggestive of proper tube placement. An increasing heart rate, if heart rate has decreased during the attempt, and a rising or normal pulse oximetry reading are suggestive of successful tube placement. Preoxygenation may significantly delay a drop in SpO2 with improper tube placement, leading to a significant delay in its recognition. Confirmation of exhaled CO2 is mandatory. It can be accomplished with use of a disposable colorimetric CO2 detector or with capnography. In situations of very low pulmonary perfusion, such as cardiac arrest, exhaled CO2 may not be detected. A chest radiograph should also be obtained to confirm proper placement of the tracheal tube, which should lie roughly halfway between the glottis and the carina (Chapter 62).
Transient Manual Ventilation in the Immediate Preintubation and Postintubation Periods
Establishment of ventilation via bag and mask or bag and tracheal tube is required prior to transport of the patient to a setting of continued critical care. The technique of manual ventilation should take into account the underlying pathology. Ventilation of patients with diseases characterized by low FRC (pneumonia, pulmonary edema, ARDS, etc.) should include the application of positive end-expiratory pressure (PEEP) to prevent alveolar derecruitment. This can be accomplished with use of a PEEP valve on a self-inflating ventilation bag or by careful manipulation of exhaust gas using an anesthesia bag. Such diseases are also characterized by a short time constant (Chapter 70) and therefore are best managed with relatively small tidal volumes and high ventilation rates. Diseases characterized by airway obstruction have prolonged time constants and are therefore best managed with relatively slow rates and high tidal volumes.
Chernick V, West J. The functional basis of respiratory disease. In: Chernick V, Boat TF, Wilmott RW, et al, editors. Kendig’s disorders of the respiratory tract in children. ed 7. Philadelphia: Saunders; 2006:29-64.
de Caen A, et al. Airway management. In: Nichols DG, Ackerman AD, Carcillo JA, et al, editors. Rogers’ textbook of pediatric intensive care. ed 4. Philadelphia: Lippincott Williams & Wilkins; 2008:303-322.
Kilpatrick FA, Wilson W. Arterial and capillary blood gas analysis. In: Barnhart S, Czervinske M, editors. Perinatal and pediatric respiratory care. Philadelphia: Saunders; 1995:114-129.
Leaver SK, Evans TW. Acute respiratory distress syndrome. BMJ. 2007;335:389-394.
Lumb AM. Pulmonary ventilation. In Nunn’s applied respiratory physiology, ed 6, Philadelphia: Elsevier/Butterworth Heinemann; 2005:76-91.
Vender J, Clemency M. Oxygen delivery systems, inhalational therapy and respiratory therapy. In: Benumof J, editor. Airway management: principles and practice. Philadelphia: Mosby; 1996:205-227.
Wheeler AP, Bernard GR. Acute lung injury and the acute respiratory distress syndrome: a clinical review. Lancet. 2007;369:1553-1564.
Wratney A, Hamel D, Cheifetz I. Inhaled gases. In: Nichols DG, Ackerman AD, Carcillo JA, et al, editors. Rogers’ textbook of pediatric intensive care. ed 4. Philadelphia: Lippincott Williams & Wilkins; 2008:532-543.
65.1 Mechanical Ventilation
Mechanical ventilation neither is intended to normalize gas exchange nor is a form of cure. The goals are to maintain sufficient oxygenation and ventilation to ensure tissue viability until the disease process has resolved and to minimize the inevitable complications of the therapeutic intervention itself. PaO2, PaCO2, and pH levels are maintained in ranges that provide a safe environment for the patient while protecting the lungs from damage due to oxygen toxicity, pressure (barotrauma), tidal volume overdistention (volutrauma), atelectotrauma, and cytokine release (biotrauma) (Figs. 65-3 and 65-4).
Basic Concepts of Ventilator Management
Equation of Motion
A pressure gradient is required for air to move from one place to another (Fig. 65-5). During natural spontaneous ventilation, inspiration results from generation of negative intrapleural pressure from contraction of the diaphragm and intercostal muscles, drawing air from the atmosphere across the airways into the alveoli. During mechanical ventilation, inspiration results from positive pressure created by compressed gases through the ventilator, which pushes air across the airways into alveoli. In both spontaneous and mechanical ventilation, exhalation results from alveolar pressure generated by the elastic recoil of the lung and the chest wall. Pressure necessary to move a given amount of air into the lung is determined by two factors: lung and chest wall elastance, and airway resistance. The relationship among pressure gradient, compliance, and resistance is described in Figure 65-5. Elastance—defined as the change in pressure (ΔP) divided by the change in volume (ΔV)—refers to the property of a substance to oppose deformation. It is opposite of compliance (ΔV ÷ ΔP), the property of a substance to allow distention or lengthening when subjected to pressure. Compliance (C) is therefore expressed as 1/elastance.
Functional Residual Capacity
During inspiration, oxygen-enriched gas enters alveoli. During exhalation, oxygen continues to be removed by the pulmonary capillary circulation. Functional residual capacity is the volume of gas left in the alveoli at the end of expiration. It is the only source of gas available for gas exchange during exhalation. In diseases with decreased FRC (e.g., ARDS, pulmonary edema), alveolar oxygen concentration declines sharply throughout expiration, resulting in hypoxemia. Two ventilator strategies commonly employed to improve oxygenation in such situations are the application of PEEP and increasing the inspiratory time (TI) (Fig. 65-6). PEEP increases FRC, whereas a longer TI allows longer exposure of pulmonary capillary blood to a higher concentration of O2 during inspiration.
Time Constant
At the beginning of inspiration, the atmospheric pressure is higher than the pressure in the alveoli, resulting in movement of air into the alveoli. During mechanical ventilation, the ventilator circuit serves as the patient’s atmosphere. As alveoli expand with air, the alveolar pressure rises throughout inspiration until it equilibrates with the ventilator pressure, at which time airflow ceases. Expiration starts when the ventilator pressure falls below the alveolar pressure. Alveolar pressure decreases throughout expiration until it reaches the ventilator pressure, at which time no further egress of air from the alveoli occurs. If inspiration or expiration is terminated before pressure equilibration between alveoli and the ventilator is allowed to occur, alveolar expansion during inspiration or alveolar emptying during expiration is incomplete. Incomplete inspiration results in delivery of decreased tidal volume, whereas incomplete expiration is associated with air trapping and the presence of residual PEEP in the alveoli that is greater than the ventilator pressure, referred to as auto-PEEP. Some time is required for pressure equilibration to occur between alveoli and the atmosphere, which is reflected in the time constant (TC). It takes 3 TCs for 95%, and 5 TCs for 99%, of pressure equilibration to occur. The time constant depends on compliance and resistance, and their relationship is depicted in Figure 65-7. Time constant is calculated as compliance multiplied by resistance (C × R) and is measured in seconds.
Diseases with decreased compliance (increased elastance) are characterized by high elastic recoil pressure, which results in more rapid equilibration of alveolar and ventilator pressures, thereby decreasing TC. Diseases with increased airway resistance are associated with slower flow rates, require longer time for movement of air from one place to another, and therefore have increased TC. Airways expand during inspiration and narrow during expiration (Chapter 365). Therefore, expiratory time constant (TCE) is longer than inspiratory time constant (TCI). In intrathoracic airway obstruction (asthma, bronchiolitis, aspiration syndromes), airway narrowing is much more pronounced during expiration. Therefore, although both TCE and TCI are prolonged in such diseases, TCE is much more prolonged than TCI. Patients with such diseases therefore are best ventilated with slower rates, higher tidal volume, and longer expiratory time than inspiratory time. In diseases characterized by decreased compliance, both TCE and TCI are short; however, the TCE is closer to TCI than in normal lungs because of the stiffer alveoli recoil with greater force. Patients with these diseases are best ventilated with small VT to prevent ventilator-induced lung injury and with a relatively longer inspiratory time in each breath to improve oxygenation.
Critical Opening Pressure
Collapsed or atelectatic alveoli require a considerable amount of pressure to open. Once open, the alveoli require relatively less pressure for continued expansion. The process of opening atelectatic alveoli is called recruitment. In a normal lung, alveoli remain open at the end of expiration, and therefore the lung requires relatively less pressure to receive its tidal volume. In a disease process in which the alveoli collapse at the end of expiration (e.g., ARDS), a substantial amount of pressure is required to open the alveoli during inspiration. This pressure causes ventilator-induced lung injury via two mechanisms: (1) barotrauma at the terminal airway–alveolar junction and (2) volutrauma due to overdistention of alveoli that are already open (see Figs. 65-3 and 65-4). Although a pulmonary parenchymal disease process is rarely uniform, and each of the millions of alveoli may have its own mechanical characteristics, a composite volume-pressure relationship could be conceptualized for the whole lung (Fig. 65-8).
Phases of Mechanical Ventilation
Control Modes
Intermittent Mandatory Ventilation Mode
In intermittent mandatory ventilation (IMV), the inspiration is initiated at a set frequency with a timing mechanism independent of patient effort. In between machine-delivered breaths, the patient can breathe spontaneously from a fresh source of gas. IMV allows for adjustment of ventilator support according to the patient’s needs and is therefore useful in the weaning process. Lack of synchrony between machine-delivered breaths and patient efforts may result in ineffective ventilation and patient discomfort, especially when IMV is delivered at a high rate. In such cases, the patient may require sedation and pharmacologic paralysis for efficient delivery of tidal volume. To obviate this problem, synchronized IMV (SIMV) is used, whereby the machine-delivered breaths are triggered by the patient’s inspiratory efforts (Fig. 65-9). In between the machine-delivered breaths, a fresh source of gas is available for spontaneous patient breaths. In the absence of patient effort, the patient receives a backup rate much like in IMV mode. Even with SIMV, ventilator-patient asynchrony can occur, because tidal volume, inflation pressure, and inspiratory time are determined by the ventilator alone.
Control Variable
Once initiated, either the tidal volume or the pressure delivered by the machine can be controlled. The machine-delivered breath is thus referred to as either volume-controlled or pressure-controlled (Table 65-8).
Table 65-8 CHARACTERISTICS OF PRESSURE-CONTROLLED AND VOLUME-CONTROLLED METHODS OF VENTILATION
PRESSURE-CONTROLLED VENTILATION | VOLUME-CONTROLLED VENTILATION | |
---|---|---|
Control setting(s) |
VT, tidal volume.
With volume-controlled ventilation (VCV), machine-delivered volume is the primary control, and the inflation pressure generated depends on the respiratory system’s compliance and resistance. Changes in respiratory system compliance and resistance are therefore easily detected from changes observed in inflation pressure. In pressure-controlled ventilation (PCV), the pressure change above the baseline is the primary control, and the tidal volume delivered to the lungs depends on the respiratory system’s compliance and resistance. Changes in respiratory system compliance and resistance do not affect inflation pressure and may therefore go undetected unless the exhaled VT is monitored. VCV and PCV have their own advantages and disadvantages (see Table 65-8). Generally speaking, PCV is more efficient than VCV in terms of amount of tidal volume delivered for a given inflation pressure during ventilation of a lung that has nonuniform time constants, such as asthma. In VCV, relatively less-obstructed airways are likely to receive more of the machine-delivered volume throughout inspiration than relatively more-obstructed airways with longer time constants (Fig. 65-10A). This situation would result in uneven ventilation, higher PIP, and a decrease in dynamic compliance. In PCV, because of a constant inflation pressure that is held throughout inspiration, relatively less-obstructed lung units with shorter time constants would achieve pressure equilibration earlier during inspiration than the relatively more-obstructed areas. Thus, units with shorter time constants would attain their final volume earlier in inspiration, and those with longer time constants would continue to receive additional volume later in inspiration (Fig. 65-10B). This situation would result in more even distribution of inspired gas, delivery of more tidal volume for the same inflation pressure, and improved dynamic compliance in comparison with VCV.
Conventional Ventilator Settings
Patient-Ventilator Asynchrony
Monitoring Respiratory Mechanics
Respiratory System Dynamic Compliance and Static Compliance
It takes into account both the flow-resistive and the elastic properties of the respiratory system. Changes in CDYN can be used to assess effects of different levels of PEEP as tidal ventilation is shifted along the slope of the volume-pressure curve (see Fig. 65-8). After an increase in PEEP in alveolar-interstitial diseases (increased elastance), an increase in CDYN suggests alveolar recruitment, whereas a decrease in CDYN may indicate overdistention. Similarly, in obstructive diseases (increased resistance), adjustment in PEEP levels to ameliorate airway collapse during exhalation can be guided by monitoring CDYN. To assess only the elastic recoil of the lung, measurement of CSTAT when there is no airflow is required. This measurement is performed by using an inspiratory hold maneuver with the patient under neuromuscular blockade and observing pressure-time and flow-time waveforms (Fig. 65-11). During this maneuver, inspiratory flow ceases while the expiratory valve continues to remain closed, thus allowing pressure to equilibrate throughout the ventilator circuit and the patient’s lungs. This pressure, referred to as the plateau pressure (Pplat), is reflective of alveolar pressure. CSTAT is calculated as follows:
Albuali WH, Singh RN, Frasier DD, et al. Have changes in ventilation practices improved outcome in children with acute lung injury? Pediatr Crit Care Med. 2007;8:324-330.
Brower RG, Lanken PN, MacIntyre N, et al. National Heart, Lung, and Blood Institute ARDS Clinical Trials Network: Higher versus lower positive end-expiratory pressures in patients with the acute respiratory distress syndrome. N Engl J Med. 2004;351:327-336.
Campbell RS, Davis BR. Pressure-controlled versus volume-controlled ventilation: does it matter? Respir Care. 2002;47:416-424.
Greenough A, Dimitriou G, Prendergast M, et al: Synchronized mechanical ventilation for respiratory support in newborn infants, Cochrane Database Syst Rev (1):CD000456, 2008.
Marraro GA. Innovative practices of ventilatory support with pediatric patients. Pediatr Crit Care Med. 2004;4:8-17.
Meade MO, Cook DJ, Guyatt GH, et al. Ventilation strategy using low tidal volumes, recruitment maneuvers, and high positive end-expiratory pressure for acute lung injury and acute respiratory distress syndrome. JAMA. 2008;299:637-645.
Mercat A, Richard JCM, Vielle B, et al. Positive end-expiratory pressure setting in adults with acute lung injury and acute respiratory distress syndrome. JAMA. 2008;299:646-654.
Newth CJ, Venkataraman S, Willson DF, et al. Weaning and extubation readiness in pediatric patients. Pediatr Crit Care Med. 2009;10:1-11.
Papazian L, Forel JM, Gacouin A, et al: Neuromuscular blockers in early acute respiratory distress syndrome, N Engl J Med 363(12):1107–1116.
Pearse RM, Young JD. Steroids to prevent postextubation laryngeal oedema. Lancet. 2007;369:1060-1061.
Pinhu L, Whitehead T, Evans T, et al. Ventilator-associated lung injury. Lancet. 2003;361:332-340.
Priestley MA, Helfaer MA. Approaches in the management of acute respiratory failure in children. Curr Opin Pediatr. 2004;16:293-298.
Randolph A. Management of acute lung injury and acute respiratory distress syndrome in children. Crit Care Med. 2009;37:2448-2454.
Sarnaik AP, Daphtary K, Meert KL, et al. Pressure controlled ventilation in children with status asthmaticus. Pediatr Crit Care Med. 2004;5:133-138.
Schweickert WD, Pohlman MC, Pohlman AS, et al. Early physical and occupational therapy in mechanically ventilated, critically ill patients: a randomized controlled trial. Lancet. 2009;373:1874-1882.
Srinivasan R, Asselin J, Gildengorin G, et al. A prospective study of ventilator-associated pneumonia in children. Pediatrics. 2009;123:1108-1115.
65.2 Long-Term Mechanical Ventilation
Patient Selection and Ethical Considerations
Indications
Optimal patient selection for home mechanical ventilation requires identification of the cause of chronic respiratory failure and awareness of the long-term outlook for the patient. Many of the causative conditions have the potential to improve with therapy, time, growth, or age. Some are amenable to surgical correction and have the potential for weaning of the patient from mechanical ventilation after an appropriate intervention is performed. Conditions requiring long-term respiratory support may involve a primary pulmonary parenchymal or airway pathology, neuromuscular or musculoskeletal abnormality, or inadequate CNS control of the respiratory system. In some cases, home mechanical ventilation is indicated to prevent the development of pulmonary hypertension and cor pulmonale from chronic hypoxic pulmonary vasoconstriction (bronchopulmonary dysplasia, obstructive sleep apnea). Other candidates for home ventilation include patients with cardiopulmonary or airway problems who are able to sustain oxygenation and ventilation but are not thriving because of excessive caloric expenditure from increased work of breathing. Tachypnea can also negatively affect oral feeding in infants, further compromising the positive caloric balance needed for growth. This scenario is very common in patients with bronchopulmonary dysplasia and congenital heart disease. Some conditions that cause chronic respiratory failure are slowly progressive and/or irreversible; some patients with these conditions are still excellent candidates for home ventilation. Many children with spinal cord injuries, muscular dystrophy, and type II spinal muscular atrophy enjoy excellent quality of life with long-term ventilation, and some have even graduated from college and become productive members of society. The type of respiratory support needs to be tailored to the individual patient’s needs and resources. Availability of resources may depend on local conditions. Also, treatment philosophy and outcome goals vary from patient to patient (Table 65-9).
Team Structure for Home Mechanical Ventilation Program
For optimal management of the child undergoing home mechanical ventilation, a comprehensive, multidisciplinary approach at a tertiary care center is required (Table 65-10). Indeed, in these cases, life-sustaining therapies are being delivered outside the ICU and often outside the hospital. Several disciplines of health care provision must be involved, and the family must be thoroughly trained to ensure the safety, growth, psychosocial development, and possible clinical improvement of the child.
Table 65-10 TEAM APPROACH TO INSTITUTIONAL HOME VENTILATION PROGRAM
TASK | PERSON(S) INVOLVED |
---|---|
Decision to institute long-term ventilation | Family, intensivist/neonatologist, pulmonologist |
In-hospital management | Intensivist/neonatologist |
Assuming overall leadership of long-term care | Pulmonologist or other qualified physician |
Medical/surgical issues | Otolaryngologist, general surgeon, neurologist, neurosurgeon, craniofacial surgeon |
Training: Suctioning, tracheostomy care, ventilator function and trouble shooting | Respiratory therapist, nurse, medical equipment company, family |
Arranging financial and nursing resources | Social worker |
Assessment of nutrition, feeding, speech | Dietician, occupational/speech therapist |
Primary care: Immunization, growth and development assessment | Primary care physician or pulmonologist |
Home medication instructions | Pharmacist, nurse, physician |
Follow-up regarding ventilator adjustments | Pulmonologist |
Assessment of home suitability | Medical equipment company |
Home care | Family, home health nursing company |
Methods of Long-Term Mechanical Ventilation
AARC, Respiratory Home Care Focus Group. AARC Clinical Practice Guideline: Long-term invasive mechanical ventilation in the home—2007 revision & update. Respir Care. 2007;52:1056-1062.
Eigen H, Zander J. Home mechanical ventilation of pediatric patients. American Thoracic Society. Am Rev Respir Dis. 1990;141:258-259.
Kacmarek RM, Steven D, Mack CW. Home respiratory care and mechanical ventilation: the essentials of respiratory care. St Louis: Elsevier/Mosby; 2005. pp 569–589
MacDuff A, Grant IS. Critical care management of neuromuscular disease including long-term ventilation. Curr Opin Crit Care. 2003;9:106-112.
Ottonello G, Ferrari I, Pirroddi IMG, et al. Home mechanical ventilation in children: retrospective survey of a pediatric population. Pediatr Int. 2007;49:801-805.
Robert D, Argaud L. Clinical review: long-term noninvasive ventilation. Crit Care. 2007;11:210-230.
Sarnaik AP, Daphtary K, Sarnaik AA. Ethical issues in pediatric intensive care in developing countries: combining western technology and eastern wisdom. Indian J Pediatr. 2005;72:339-342.
Simmonds AK. Home ventilation. Eur Respir J. 2003;22(Suppl 47):38S-46S.
Sritippayawan S, Kun SS, Keens TG, et al. Initiation of home ventilation in children with neuromuscular diseases. J Pediatr. 2003;142:481-485.