CHAPTER 77 Radiotherapy for Head and Neck Cancer
Radiation Physics, Radiobiology, and Clinical Principles
Clinical Principles of Radiotherapy
Toward the closing years of the nineteenth century, many investigations into electricity characteristics were conducted, one of them demonstrating that electric potential placed across two separated platinum electrodes produces a spark.1 British physicist William Crookes demonstrated that if the two electrodes were placed within an evacuated glass tube, the vacuum would eventually cause the walls of the vessel to fluoresce.2 On November 8, 1895, Wilhelm Roentgen, while performing an experiment with the Crookes tube, accidentally left a piece of paper painted with barium platinocyanide nearby; he noted that the paper fluoresced and realized that this fluorescence of the paper could have been caused by a new, invisible type of ray that the tube was now emitting. Thus, the x-ray was discovered.
Radioactivity was discovered shortly after by Henri Becquerel, who investigated the capability of different substances to produce x-rays. He observed the darkening of photographic plates by uranium salts and concluded that the same x-rays were emitted spontaneously and continuously from the uranium.3 Pierre and Marie Curie, who read his results, coined the term radioactivity to describe this phenomenon. In 1898 they isolated a material with radioactivity 60 times higher than uranium and called it radium.4
These discoveries led to radiation biology experiments. The first documented experiment was performed unintentionally at about the same time, when Antoine Becquerel developed a “burn” on his chest from carrying a vial of radium salt in his vest pocket. It soon became apparent that radiation had the ability to produce profound biologic changes. In the beginning it was believed to be a magical cure for almost every known illness. The first documented success was reported in 1899 in Stockholm5 by Thor Stenbeck, who treated a 49-year-old woman’s nasal basal-cell carcinoma. He delivered 100 treatments in the course of 9 months, and the patient was alive and well 30 years after the treatment. In 1901, Dr. Frand Williams in Boston reported on the successful treatment of a lip cancer.5 The early treatments often involved very large single exposures that resulted in extensive skin toxicities and other complications. Therefore, only superficial sites were originally treated by the direct application of radium.6 Eventually, physicians started to insert radium directly into deep-seated tumors, effectively beginning the field of brachytherapy.7
The use of external beam treatments made its leap in 1922 when Coutard and Hautant reported a new concept of fractionated treatments: advanced laryngeal cancer could be cured without severe toxicities using fractionated treatments.8 Advances in measurements were also achieved when the skin erythema dose (the dose of x-rays required to give a light skin reaction) was replaced by the roentgen in 1928,9 which was later replaced by the rad. The rad is the unit of absorbed dose and is a measure of the energy deposition per unit mass by all types of ionizing radiation. The next step was achieved with the development of higher-energy machines capable of depositing dose at depth.
Basic Physics
Characteristics of Radiation
Radiation refers to the propagation of energy through space or a medium (Fig. 77-1). Radiation can be broadly classified as either particulate or electromagnetic. If the radiant energy is carried off by a particle that has rest mass, the radiation is called “corpuscular” or “particulate” radiation. Examples of particulate radiations are electrons, beta particles, protons, neutrons, and heavy charged particles.
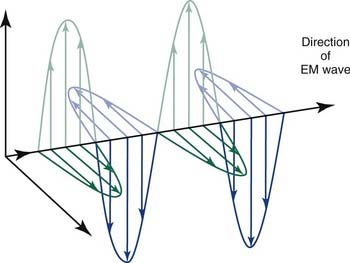
Figure 77-1. Propagation of electromagnetic (EM) radiation with oscillating electric and magnetic fields.
(From Saw CB. Foundation of Radiological Physics. Omaha, NE: C. B. Saw Publishing; 2004:10, with permission.)
where h is Planck’s constant, having a value of 6.626 × 10−34 joule-second (J.s). The energy of a photon is often expressed in electron volts (eV). One eV represents the amount of energy required to accelerate an electron through a potential of one volt. The frequency (ν) of a photon is related to its wavelength (λ) as follows:
where c = 3.0 × 108 m/sec, the speed of light in a vacuum. The waves of electromagnetic radiation are composed of oscillating electric and magnetic fields that are orthogonal to each other and to the direction of propagation, as shown in Figure 77-1. The electromagnetic spectrum spans a broad and continuous range from radiowaves to x-rays with wavelengths from 106 to 10−13 m. Radiation with wavelengths shorter than visible light is classified as ultraviolet rays, x-rays, and gamma rays. The boundaries between these regions are not sharply defined. For example, x-rays and gamma rays are indistinguishable except for their origins, one from the orbital electrons and the other from the nucleus, respectively. Different types of electromagnetic radiation interact differently with the same material (see Fig. 77-1).
Radiation Production from Linear Accelerators
Another type of radiation is termed bremsstrahlung11 (German for “braking radiation”), electromagnetic radiation produced by a sudden slowing down or deflection of charged particles (especially electrons) passing through matter near the strong electric fields of atomic nuclei.
Interaction of X-Rays with Matter
The most important of these interactions in RT are represented in Figure 77-2.
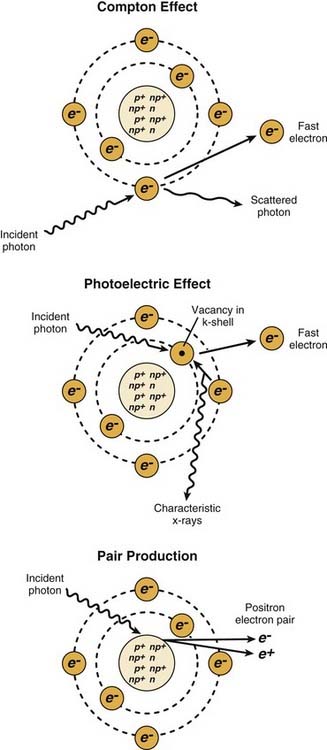
Figure 77-2. The major radiation interactions. e, electron; n, neutron; p, proton.
(Redrawn from Cox JD, Ang KK, eds. Radiation Oncology: Rationale, Technique, Results. 8th ed. St. Louis: Mosby; 2003:5.)
Radiation in Conventional Medicine
Linear accelerators (linacs) produce megavoltage x-rays. Commercially available medical linear accelerators produce x-rays and electrons with an energy range from 4 MeV up to around 25 MeV.11 The x-rays themselves are produced by the rapid deceleration of electrons in a target material, typically a tungsten alloy, which produces an x-ray spectrum via bremsstrahlung radiation. The shape and intensity of the beam produced by a linear accelerator may be modified or collimated by a variety of means. Thus, conventional, conformal, intensity-modulated, tomographic, and stereotactic radiotherapy are all produced by specially modified linear accelerators (Fig. 77-3).
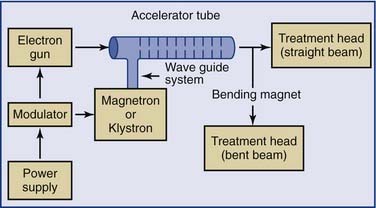
Figure 77-3. Block diagram of a typical medical linear accelerator.
(From Leibel SA, Phillips TL, eds. Textbook of Radiation Oncology. Philadelphia: WB Saunders; 1998:110.)
Additional magnetic or electrostatic lens elements may be included to ensure that the beam remains in the center of the tube and its electrodes (see Fig. 77-3).
Linear Accelerators
As an energy source, electrons differ from photons in that electrons travel only a certain (short) distance within tissue. They are very light particles compared with the nuclei of the target tissue with which they interact. Hence, the electrons lose a large fraction of their energy in a single process. This leads to much less skin sparing and the deposition of the majority of the dose in superficial tissues. Consequently, however, they are very useful for treatments in which the target of the radiation lies close to the surface of the patient, such as skin tumors (Fig. 77-4).
Particle Beams
One particle for which there has been a great amount of clinical work is the fast neutron.12,13 Fast neutrons are of clinical interest because of their radiobiologic properties, which occur because of the much greater amount of energy they deposit when they go through tissue. Neutrons are neutral particles and interact with the atomic nuclei, producing “heavy” charged particles such as protons, α-particles, or nuclear fragments that in turn create a dense chain of ionization events as they go through tissue. The distribution of these secondary particles depends on the energy spectrum of the neutron beam, so the biologic properties of the beam depend strongly on its energy spectrum. Neutrons used in therapy generally are produced by accelerating charged particles, such as protons or deuterons, and impacting them on a beryllium target.
There is considerable interest in using charged particle beams directly for therapeutic purposes, which generally requires beams of much higher energy than those used to produce neutrons. The lighter particles, such as protons14 and α-particles, are of interest because of their extremely favorable depth-dose characteristics. The radiobiologic properties of these beams are similar to those of conventional photon or electron beams. Heavy charged particles combine the favorable depth-dose properties of the proton and α-particle beams with the favorable biologic properties of the neutron beams. Energies are on the order of several hundred MeV per nucleon, rather than the few MeV per nucleon for the recoil fragments produced by neutrons. These highly energetic particles do not deposit much energy in tissue until they reach the end of their path, where they are moving slowly. Hence, they do not produce much radiation damage in the intervening tissues. Because of their extremely high cost and general unavailability, however, these beams are not widely in use. Interest in proton beams has grown, and the number of radiotherapy facilities using protons in the United States is expected to rise.
Treatment Aspects of External Beam Radiation
Typically, the radiation dose is delivered to the patient using a linear accelerator (Fig. 77-5). The radiation beam as produced is a forward peak; that is, it has extremely high intensity along the beam axis. It must pass through a conical metallic flattening filter to create a uniform field beam for clinical use. As the clinical beam exits the linear accelerator, it is collimated by a pair of jaws or a multileaf collimation (MLC) system (see Fig. 77-5). The multileaf collimation system is used to shield and protect normal structures (replacing the antiquated and cumbersome lead blocks). In addition, the system is used to modulate beam intensity in intensity-modulated RT. The linear accelerator has a gantry that allows the rotation of the treatment head around the patient. Hence, the radiation beam can be directed at the target from multiple positions to reduce dose to the normal tissues. Because of the high voltage, moving parts, and high dose, the linear accelerator must be properly calibrated and properly maintained for its safe use (see Fig. 77-5).
Radiobiology
Radiation cell killing occurs when critical targets within the cell are damaged by radiation.15 A number of biologic molecules or structures are potential targets for radiation damage. According to most studies DNA is the most critical target for the biologic effects of radiation. On a molecular level, this effect requires the production of ionizations, which is why we refer to the process as ionizing radiation. The damage can occur directly when the radiation is absorbed by the DNA itself, because the atoms of the DNA become ionized and damaged. More commonly, however, it occurs indirectly through the following three modes of action15,16:
All of these species are highly reactive free radicals that, in turn, interact with the DNA and cause damage. Both ways eventually cause broken bonds in the DNA backbone, which can cause double-strand breaks, ultimately resulting in mitotic death (Fig. 77-6).
These broken bonds can result in the loss of a base or of the entire nucleotide, or in complete breaking of one or both of the strands of DNA. Single-strand breaks are easily repaired with use of the opposite strand as a template. Therefore, single-strand breaks show little relation to cell killing, although they might result in mutation if the repair is incorrect. Double-strand breaks, on the other hand, are thought to be the most important lesion in DNA produced by radiation.18 Double-strand breaks, as the name implies, results in snapping of the chromatin into two pieces. These double-strand breaks can result in mutations or, most important, in cell killing.
A growing body of experimental data suggests that radiation damage to DNA is not the only mechanism by which ionizing radiation damages cells. Other mechanisms are apoptosis, cell cycle arrest, and mitotic death. One study has suggested that apoptosis can be triggered by radiation energy deposition in cell membranes.19 It has also been reported that direct radiation damage to mitochondria can trigger apoptosis.20
Cell Cycle Arrest
Radiation triggers signaling cascades leading to arrest, usually at the G1 and G2 checkpoints in the cell cycle.21 Cell cycle perturbations (Fig. 77-7) are seen characteristically after radiation exposure and were among the earliest observed biologic effects of radiation. Cells can show checkpoints or arrest in any phase of the cell cycle, although the best-described checkpoints with respect to radiation damage are the G1 and G2 checkpoints. Normal cells and those cancer cells that retain p53 function are blocked in the G1 phase of the cell cycle. This is a p53-mediated event.
Radiosensitivity
Our main interest in radiobiology is in figuring out ways to improve the treatment toxicity ratio. One of the main concepts is radiation sensitivity, which refers to the relative susceptibility of cells, tissues, tumors, or organisms to radiation. However, tumor regression is not solely a function of tumor cell death, but is influenced by many factors, including the amount of extracellular stroma, the propensity of the tumor cells to undergo rapid rather than delayed death, and the resorption of radiation-inactivated cells (depopulation). A frequent misconception is that tumors should be more radiosensitive than normal tissues because they proliferate more rapidly. This misconception may go back 100 years. In 1906, only 11 years after the discovery of x-rays, Bergonie and Tribondeau formulated a “law” for the relationship between cellular radiosensitivity and reproductive capacity.22 They postulated that cells that have a higher proliferation rate are more radiosensitive than slowly proliferating cells. On a purely cellular basis, it is correct that cells in mitosis at the time of irradiation are more radiosensitive than cells in other phases of the cell cycle. However, many other factors, such as tissue- and host-specific factors, have important influence. In 1906, there was no appreciation of late-occurring normal tissue complications. Nowadays, we know that many slowly proliferating or nonproliferating normal tissues, such as the kidney, are highly radiosensitive; they just express radiation injury much later than rapidly proliferating tissues. Similarly, the proliferation rate of tumors does not predict their radio-curability. For example, rapidly proliferating tumors such as glioblastoma multiforme can be highly radioresistant.
Cell Survival
Loss of reproductive integrity in long-term survival assays is important to our understanding of the response of either a tumor or a normal tissue to radiation. When cells are exposed to lethal doses of radiation, they may not die immediately or within a few hours of treatment, or even sometimes within a single division of radiation. When cells have been observed by time-lapse cinematography after irradiation, some cells survive and go on to form colonies, and some die quickly. Others go through up to several rounds of abortive cell division before finally ceasing to divide. Radiation biologists have demonstrated that it is the proportion of cells capable of forming a colony by sustained cell division that most fully predicts the effects of a dose of radiation.23 In order to eradicate or control a tumor; one must inactivate all clonogenic tumor cells. In other words, the treatment may fail if only one clonogen survives, because that cell can give rise to a regrowing tumor. In order to understand tumor control better, plots of the surviving fraction of cells as a function of the radiation dose have been performed. An example is shown in Figure 77-8.
By convention, the surviving cell fraction is plotted on a logarithmic scale, and the radiation dose is plotted on a linear scale. This curve is representative of most mammalian cells. Consider the solid curve in Figure 77-8, which represents the survival data. Note that there are two distinct regions to the curve. There is an initial region for low radiation doses, where the slope of the curve is shallow. In this region, small incremental changes in the amount of radiation are not very effective at increasing the number of cells killed. This is called the shoulder region, and its width is characterized by the parameter Dq. It is the distance along the dose axis at a surviving fraction of unity between the abscissa and the point where the extrapolated linear portion of the curve is intersected. It is a measure of the ability of the cells to repair small amounts of radiation damage. At higher doses of radiation, the curve becomes a straight line on a semilog plot. Its slope is characterized by Do, which is the incremental dose change required to reduce the surviving cell fraction to 1/e of its value. The steeper the slope in this region, the smaller is the value of Do and the more radiosensitive is the cell line. When extrapolated back to a zero radiation dose, it intersects the abscissa at a value N. A curve of this type can be modeled using the following equation:
where S is the surviving fraction, D is the radiation dose, and N and Do are as indicated in Figure 77-8. In target theory, N can be thought of as the number of distinct targets in the cell that should receive one radiation “hit” before the cell is inactivated.
A number of mathematical models have been devised to attempt to describe the shape of the cell survival curves that are observed experimentally, with an initial shallower slope, an eventual bending (the “shoulder”), and a final, steeper slope. These include target models, lethal and potentially lethal damage models, and repair saturation models. Some of these have been based on simple mathematical modeling without any real attempt to model known molecular events involved in cell killing, whereas others have been based on attempts to model some of the known molecular events (e.g., chromosome breaks or DNA repair) that are involved in cell killing. All of the models can describe the shape of the survival curve to a first approximation. None does so perfectly, and none takes into account all the events and all of the possible mechanisms involved in cell death. A model that has been most influential on clinical practice is the linear quadratic model,24 because it is one of the models that best fits the behavior of cells after exposure to radiation doses within the range used in the clinic. The linear-quadratic model was devised by Keller and Rossi,25 who proposed that radiation-induced cell killing resulted from two potential events, one with a linear relation to dose (exp[−αD]), the other having a quadratic relation to dose (exp[−βD2]). This was expressed mathematically by the “alpha-beta” equation, as follows:
where S represents survival of a cell population after a dose, D (Fig. 77-9). It has been hypothesized that this equation actually represents a molecular reality.26 Double-strand breaks in the DNA are lethal lesions that are produced by either one energy deposition, an event termed (αD), or by two separate events, each involving a single strand of DNA (βD2), which then interact. This hypothesis is now thought to be unlikely because of the low probability of two tracks interacting within a single double helix.
The α/β ratio, which is derived from Equation 77-4, represents a point on the survival curve at which the components of cell killing are equal to each other—that is, αD = βD2, or D = α/β. In other words, for each cell population there is a dose of radiation in which the linear (α) and quadratic (β) contributions to cell killing are equal. The α/β ratio is specific to each cellular population and reflects the sensitivity of the cell to the two types of damage. Tissues that have an early response to radiation (skin, mucosa, and tumor cells) have a high α/β ratio. In other words, their survival curves stay straight for a longer period before the bend occurs, in which there is a higher contribution of single-event or α killing. Late-responding tissues, such as spinal cord, kidney, and muscle, have survival curves that bend earlier, with resultant lower α/β ratios. These late-responding tissues have shoulders on their survival curves within the range of doses commonly used in RT. This understanding led to the concept that altered fractionation schedules could be used to treat tumor populations more effectively with respect to damage to late-responding tissues.
Tissue-Radiation Characterizations
The main intent in clinical radiotherapy is to irradiate tumoral tissues while minimizing the damage to the surrounding healthy tissues. The four main concepts of clinical radiotherapy are known as “the four Rs of radiotherapy”: repair (of sublethal damage), redistribution (across the cell cycle), repopulation, and reoxygenation. Radiotherapy basically works not because tumors are more radiosensitive than normal tissue but because normal tissues are better at repair and repopulation. These four Rs also account for different response rates of tumors.27–29
Reoxygenation of the tumor cells causes the cells to be more radiosensitive. The response of cells to ionizing radiation is strongly oxygen dependent, with well-oxygenated cells showing up to threefold greater sensitivity to the killing effects of ionizing radiation than the same cells under hypoxic conditions (Fig. 77-10).30 Tumors tend to have a much higher proportion of hypoxic cells than normal tissues do. Therefore it is important to allow tumor cells to reoxygenate. However, if too much time occurs between fractions, repopulation or proliferation of the tumor cells could occur. Therefore the main issue is creating a balance between reoxygenation of the tumor and its repopulation and repair, and at the same time minimizing the damage to the surrounding tissue.
In order to evaluate the impact of a radiation treatment, time-dose considerations are important in estimating the effect of a given total radiation dose.31 If the dose were given in a single fraction, then the healthy tissues would experience more cell killing than if it were given in a fractionated manner. This difference occurs because single fractions allow no opportunity for repair of sublethal damage. In general, smaller total radiation doses given over shorter total treatment times can produce the same normal tissue effects as larger total radiation doses given over longer intervals.
The classic measurements that illustrate this point are the isoeffect measurements on skin that were made by Strandquist.32 He showed that the isoeffect lines for various degrees of skin damage and for curing skin cancer were straight when plotted on a log-log scale of total dose versus time. Moreover, the lines appeared to have the same slope (i.e., were parallel). The required dose to produce a given effect was proportional to time to the 0.33 power. Ellis33 extended this concept to clinical radiotherapy by allocating a portion of the exponent 0.33 to the overall treatment time, T, and a portion to the number of fractions, N. He defined the nominal standard dose (NSD) with the following equation:
where Dt is the total radiation dose. The exponents in this expression are for skin and no doubt vary for other tissues.
Clinical Applications
Radiation Fractionation
Altered Fractionation Schedules
Four prospective randomized trials comparing hyperfractionation with standard irradiation alone (without chemotherapy) have been performed.34–37 In all studies locoregional control was significantly better for those patients treated with hyperfractionation and accelerated fractionation with a concomitant boost than for those treated with standard fractionation. The advantage ranged from an 8% to a 20% 2-year locoregional control rate. There was also a trend toward better disease-free survival with hyperfractionation. In one of the studies, which dealt specifically with oropharynx tumors, there was an improvement in overall survival: Overall survival rate at 42 months was 27% for the hyperfractionation arm and 8% for the conventional treatment arm (P = .03).36 Survival rates were 40% for the hyperfractionated arm and 18% for the conventional RT arm (P = .06). All groups treated with altered fractionation RT had significantly greater late side effects than those undergoing conventional fractionation. The acute side effects of hyperfractionation were slightly more intense and common than with standard fractionation, but the long-term effects appear to be very similar.
Accelerated fractionation is the administration of multiple daily radiation doses of larger size to reduce the overall treatment time. The goal of accelerated fractionation is to prevent tumor repopulation during a course of radiation therapy. Clinical support for tumor cell proliferation during therapy is found in numerous studies that have shown a loss in tumor control with prolongation of overall treatment time in HNC. In general, accelerated treatments that employ continuous (rather than split-course) RT schedules without compromise in the total dose result in better local control. However, whether the added mucosal toxicity from this approach is justified by meaningful gains in survival remains an open question. The studies present a trend toward better primary tumor control in the hyperfractionation arm but no significant difference in overall survival. Comparison of accelerated RT with conventional RT for locoregionally advanced HNC has been tested in a considerable number of randomized trials.34–41 All of these trials used somewhat different dose fractionation schedules, and the results (in terms of both locoregional control and survival) are inconsistent. The only trial suggesting a survival benefit for this approach was the smallest.39
The reasons for these inconsistent findings may be the differences in treatment regimens used. In some studies overall treatment time was decreased by more than 50%, necessitating a decrease in total dose by approximately 10% to 20%.38,40,41 This approach resulted in significantly better locoregional control in only one trial,38 and none showed gains in overall survival. Another approach involved moderate reduction in the overall treatment time without a relevant compromise in total dose, with the use of split-course RT regimens.34,35,38 With this strategy, no benefit in local control or survival has been observed, except for a significant improvement in local control in one of the trials.35 This improvement did not translate into an improvement in overall survival, however. This lack of survival benefit has been attributed, at least in part, to the significantly higher rate of severe late toxicities in the accelerated treatment arm, which resulted in an increase in the non–cancer-related death rate.
Moderate reduction of the overall treatment time without a relevant compromise in total dose has also been achieved in two studies, with the use of six or seven fractions per week (compared with the usual five fractions weekly) without a split course.39,42 Both of these studies showed a significantly improved local control rate in the accelerated treatment arm, and one demonstrated a trend for an improvement in overall survival.39 Both studies demonstrated a significant increase in acute side effects. Whether late toxicity is also worse with the accelerated treatment approach is unclear. The available data are conflicting, with some trials suggesting no increase in late toxicity,38 and others suggesting the opposite.35
The benefit of altered fractionation RT for squamous cell HNC was addressed in a meta-analysis of 6515 patients enrolled in 15 selected trials comparing hyperfractionated or accelerated fractionation RT with conventional fractionation RT.43 The majority were treated for stage III or IV disease. Compared with conventional fractionation RT, altered fractionation RT resulted in a statistically significant 18% reduction in rate of local failure at the primary site, corresponding to a 6.4% absolute reduction in rate of locoregional failure at 5 years. The improvement in locoregional control was more pronounced in patients undergoing hyperfractionated RT and in those treated with accelerated RT without dose reduction. A significant benefit in overall survival was also found; altered fractionation regimens reduced the risk of dying by 8%, which translated into an absolute survival benefit of 3.3% and 3.4% at 2 and 5 years, respectively. The magnitude of benefit was statistically significant in patients treated with hyperfractionated RT, but not for those undergoing accelerated fractionation RT, with or without a dose reduction. Although this meta-analysis demonstrates the advantage of hyperfractionation versus conventionally fractionated radiation, the standard of care today is chemoradiation, and these studies do not demonstrate superiority of any RT regimens over chemoradiation.
The benefit of combining radiosensitizing chemotherapy with hyperfractionated RT (CRT) schedules was shown in one study in which 116 patients with HNCs (approximately 53% of which were unresectable) were randomly allocated to undergo hyperfractionated RT either alone or with 5-fluorouracil (5-FU) and cisplatin.44 At 3 years, CRT was associated with significantly higher rates of locoregional control (70% vs. 44%), a trend toward better relapse-free survival (61% vs. 41%, P = .08), and better overall survival (55% vs. 34%, P = .07). Toxicity, although high, was equivalent in the two groups. However, no trial has compared a regimen using hyperfractionated RT with or without chemotherapy directly with a regimen using concomitant CRT and conventional fractionation RT, the widely accepted standard of care, at least in the United States, for locoregionally advanced laryngeal and oropharyngeal cancers. These twice-daily treatments place greater time demands on the patient as well as on the treatment facility and may create problems with compliance. As a result, hyperfractionated regimens have not been widely adopted, at least in the United States.
Radiosensitizers
Reducing Hypoxia
Within the tumor cell microenvironment, radiation therapy effectiveness is closely dependent on oxygen availability at the time of treatment. Hypoxic cells are 2.5 to 3 times less radiosensitive than well-oxygenated cells.45,46 The hypoxic cell fraction in solid tumors ranges from less than 1% to more than 50%.47 Hypoxia in the tumor microenvironment may be a result of increased interstitial pressure, which leads to vascular collapse with resulting areas of transient or persistent hypoperfusion, local hypoxia, and acidosis.48 Many patients with cancer are anemic, a factor that may also contribute to localized hypoxia and that has an adverse effect on curability of a tumor by radiation therapy.49 In order to overcome the hypoxia, specific trials addressing this issue were performed. These explored the concept of increasing the delivery of oxygen, also known as hyperbaric oxygen therapy (HBO), which increases the amount of oxygen in the blood. The use of carbogen, also raises the local oxygen level. Although the use of HBO was associated with significant increases in response rate (clinical complete response rate 84% with HBO vs. 52% without it),50 there were no significant differences in 5-year rates of local control or survival. Inhalation of carbogen (95% oxygen–5% carbon dioxide) during radiation therapy, often combined with accelerated radiation and oral nicotinamide (ARCON treatment)6,7 has been suggested in several trials, and its benefit has not been confirmed.
A second approach to decreasing the impact of hypoxia is the use of hypoxic cell sensitizers, some of which have been used in concomitant chemotherapy regimens, including nimorazole and tirapazamine. Although results of initial clinical trials have been promising, so far there is no proven evidence for survival benefit.51–53
Several trials have evaluated the hypothesis that outcomes might be improved through correction of preexisting anemia using recombinant human erythropoietin (epoetin or darbepoetin); this hypothesis was supported by the results of uncontrolled studies.52 However, subsequent randomized trials directly testing this hypothesis did not confirm benefit and, in fact, suggested significantly worse locoregional control and overall survival outcomes, or both, in patients who received epoetin during therapy for HNC.54,55
Concomitant Chemotherapy
The additive effect may occur in two different ways, as follows:
Synergistic or additive effects are thought to be due to the following mechanisms56–58:
UNRESECTABLE TUMORS
Several meta-analyses have been performed to analyze the use of chemoradiotherapy in unresectable tumors. One meta-analysis of trials evaluating locoregional treatment with or without chemotherapy suggested that the magnitude of the absolute survival benefit with chemotherapy (given in any fashion with RT) was 4% at five years.61 The hazard ratio (HR) for death was 0.81 (95% confidence interval [CI] 0.76 to 0.88) with concomitant chemoradiotherapy compared with locoregional therapy alone, and the benefit was more pronounced with multiple-agent chemotherapy regimens (hazard ratio 0.69).
A second meta-analysis of randomized trials testing curative intent RT alone or with concurrent or alternating chemotherapy concluded that the addition of concurrent (but not induction) chemotherapy to conventional or altered fractionation RT prolonged survival by an average of 12 months.62 Benefit was most pronounced in those trials that used 5-FU or cisplatin-based chemotherapy (average survival prolongation 24 months and 16 months, respectively).
INDUCTION CHEMOTHERAPY
The majority of studies suggest that there is no obvious role for induction chemotherapy. Tumor cells that remain after chemotherapy are likely to be chemoresistant and to show cross-resistance to radiation therapy as well.63 Delaying radiation treatment can lead to accelerated repopulation of surviving tumor clonogens, which occurs during an extended total treatment time. There are also practical issues. Induction chemotherapy is associated with increases in toxicity (compared with local therapy without chemotherapy), cost, and overall duration of treatment. Some patients receiving induction chemotherapy do not go on to receive definitive therapy because of the toxicities.
Several trials have compared different regimens of induction chemotherapy.64,65 The triple regimen of docetaxel or paclitaxel plus cisplatin and 5-FU has been compared with a regimen using cisplatin plus 5-FU in the setting of induction chemotherapy. The studies demonstrated that the taxane-containing regimen (with docetaxel or paclitaxel) provided superior outcomes, reporting a 14% improvement of overall survival at 3 years and improvement in median survival from 30 to 71 months.65 However, neither of these studies compared induction chemotherapy with direct chemoradiation. In the study by Hitt and associates,64 13% of the patients assigned for the better-tolerated PCF induction regimen dropped out and did not receive the definitive treatment, a situation that might lead to worse survival than that with standard treatment. In order to answer this question, three large randomized trials have been initiated to test the benefit of adding induction chemotherapy to chemoradiotherapy. Until these trials are completed, this approach should be considered investigational.
ORGAN PRESERVATION
The U.S. Department of Veterans Affairs (VA) Laryngeal Cancer Study was the first published randomized trial using neoadjuvant chemotherapy with the major endpoint of organ preservation.66 In this study, 332 patients with advanced laryngeal cancer, either stage III (53%) or stage IV (47%), were randomly assigned in equal numbers to one of the following two regimens:
A second publication from the same study reported long-term survival in the chemoradiation group only.67 At the time of that report, 53% survival was noted at 3 years. Patients who had a histologic CR had better survival than patients with persistence of tumor after chemotherapy.
Subsequently, Forastiere and colleagues68 compared RT alone with either induction chemotherapy followed by RT or concomitant CRT, with larynx preservation as the primary end point. In their initial report, 2-year laryngectomy-free survival rates significantly favored the concomitant chemoradiotherapy group (88% vs. 75% for neoadjuvant chemotherapy and 70% for RT alone), and the rates of local control were also significantly better (80%, 64%, and 58%, respectively).68 There were no significant differences when induction chemotherapy followed by RT was compared with RT alone, and overall survival was nearly identical in all three groups (2-year and 5-year survival rates 74% to 76%, and 54% to 56%, respectively).
In a later report that this group presented at the 2006 meeting of the American Society of Clinical Oncology, there were no longer any differences in laryngectomy-free survival between the groups receiving concomitant chemoradiotherapy and induction chemotherapy followed by RT (47% versus 45% at 5 years, respectively, compared with 35% for RT alone).69 However, the overall laryngeal preservation rate still favored concomitant therapy (84% versus 71% respectively, compared with 66% with RT alone), as did locoregional control rates. This study has established concomitant CRT as a standard of therapy in this setting.
POSTOPERATIVE TREATMENT
Two large trials have reported a survival benefit for combined chemotherapy and conventional fractionation over radiation alone after surgical resection among patients with locally advanced but resectable disease.70,71 These benefits came at the cost of enhanced acute mucosal toxicity, although late toxicities were not more common.
In a multi-institutional trial sponsored by the European Organization Research and Treatment of Cancer (EORTC), 334 patients with high-risk resected squamous cell cancer (SCC) of the oral cavity, oropharynx, larynx, or hypopharynx were randomly assigned to RT alone or the same dose of RT with concomitant cisplatin.62 High-risk disease was defined as a T3 or T4 primary with any nodal stage (excepting T3N0 laryngeal cancer), involved surgical margins, extracapsular extension, perineural invasion, vascular invasion, or oral cavity/oropharyngeal primary sites with involvement of level IV or V lymph nodes.
At a median follow-up of 60 months, concomitant chemoradiotherapy was associated with significantly better 5-year rates of progression-free survival (47% vs. 36%) and overall survival (53% vs. 40%), fewer locoregional relapses (18% vs. 31%), and a significantly longer time to progression (55 versus 23 months) than those for PORT alone. In a similarly designed Radiation Therapy Oncology Group (RTOG) trial, 459 patients with resectable high-risk SCC of the oral cavity, oropharynx, larynx, or hypopharynx were randomly assigned to RT alone or the same doses of RT with concomitant cisplatin on days 1, 22, and 43.70 The definition of “high-risk” differed somewhat from that used in the EORTC trial, consisting of positive resection margins, involvement of two or more lymph nodes, or extracapsular nodal extension. At a median follow-up of 46 months, chemoradiotherapy was associated with a significantly better 4-year disease-free survival (40% vs. 30%) and fewer locoregional relapses (19% vs. 30%) than those for PORT alone. The difference in overall survival was not statistically significant (hazard ratio 0.84, P =.19). As in the EORTC trial, the incidence of acute severe mucosal toxicity was significantly greater in the chemoradiotherapy group (77% vs. 34%). Long-term toxicity rates were comparable in the two groups, although data derived from larger numbers of patients undergoing definitive treatment suggest that the rate of long-term toxicity is higher in patients receiving chemoradiotherapy than in those undergoing RT alone.
In order to suggest treatment guidelines, risk stratification was addressed in a pooled analysis comparing the selection criteria, clinical and pathologic risk factors, and treatment outcomes of the EORTC and RTOG studies.71 The findings can be summarized as follows: Extracapsular extension and/or microscopically involved surgical margins were the only risk factors for which the effect on survival of adding chemotherapy to RT was statistically significant in both trials. There was a trend toward better survival in favor of chemoradiotherapy in the group of patients who had stage III or IV disease, perineural infiltration, vascular embolisms, and/or clinically enlarged level IV or V lymph nodes secondary to tumors arising in the oral cavity or oropharynx, but the differences were not statistically significant. Patients in whom two or more histopathologically involved lymph nodes without extracapsular extension was the only risk factor that did not seem to benefit from the addition of chemotherapy.
Targeted Therapy
In order to reduce side effects and optimize treatment, several studies have substituted chemotherapy with molecular targeting antibodies. The most prominent molecule thus far investigated has been cetuximab (Erbitux, IMC225), which is a monoclonal antibody that is directed against the epidermal growth factor receptor and often over-expressed in the majority of HNCs. Cetuximab inhibits receptor activity by blocking the ligand binding site. The benefit of cetuximab as a radiation sensitizer was tested in a multicenter trial72 in which 424 patients with locoregionally advanced SCC of the oropharynx, hypopharynx, or larynx were randomly assigned to RT (once daily, twice daily, or with a concomitant boost, with the specific approach selected by each participating institution) with or without weekly concurrent cetuximab. The majority of patients underwent concomitant boost RT (56%), whereas once daily and twice daily fractionation schedules were used in 26% and 18% of patients, respectively. Cetuximab was administered at a dose of 400 mg/m2 over 2 hours 1 week prior to RT, then at 250 mg/m2 over 1 hour for subsequent weekly doses during the RT weeks. At a median follow-up of 54 months, the cetuximab-treated group had significantly better median (49 vs. 29 months) and 3-year survival rates (55% vs. 45%) than the group receiving RT alone. Locoregional control rates were also significantly better (50% vs. 41%, respectively), and there was also a suggestion of a higher laryngeal preservation rate in the cetuximab group (88% versus 80% at 3 years, respectively).72 The cumulative rates of distant metastases at 2 years were similar (16% versus 17%, respectively).72 Although cetuximab did not appear to exacerbate the toxic effects commonly associated with RT (i.e., mucositis, xerostomia, dysphagia, and weight loss), patients receiving combined therapy had a greater incidence of grade 3 or 4 skin toxicity (17% vs. 1% rate of acneiform rash, respectively).
The next generation of clinical trials is focusing on combinations of RT, cetuximab, and conventional cytotoxic chemotherapy agents. Although early reports using this approach are promising, it may result in greater toxicity, and incorporation of this strategy into clinical practice would be premature.73
Advanced Radiation Technologies
In traditional irradiation of HNC, the placement of the radiation fields and their shapes were based on the bony anatomy acquired by the simulator diagnostic-quality films. During the late 1980s, advancements in computer technology and imaging introduced methods to identify the targets on CT scans and display the radiation beams in three dimensions relative to the anatomy. In addition, calculation and display of the radiation dose distributions and methods to evaluate and compare rival plans with dose-volume histograms (DVHs) became available. The introduction of multileaf collimators facilitated an increase in the number of beams that could be delivered without a large extension of treatment time. The result was the emergence of three-dimensional conformal radiotherapy (3DCRT), which allowed better precision of irradiation delivery to image-based targets and some improvements in the sparing of noninvolved critical tissue. Early studies of the utility of 3DCRT in HNC examined larynx,73 nasopharynx,74 hypopharynx,75 and paranasal sinuses cancers.76 These studies demonstrated a significant benefit for 3DCRT—better coverage of the tumors and reduced doses to critical tissue—over standard techniques.
Intensity-Modulated Radiotherapy
Intensity-Modulated Radiotherapy in Head and Neck Cancer
The anatomy of the neck is complex, with many critical and radiation-sensitive organs in close proximity to the targets. Tight dose gradients around the targets that limit the doses to the noninvolved tissue, features characteristic of IMRT, are desirable and offer the potential for therapeutic gains (Fig. 77-11). Noninvolved tissues, the sparing of which may offer tangible gains, include the major salivary glands, minor salivary glands dispersed within the oral cavity, the mandible, and the pharyngeal musculature. In the cases of nasopharyngeal and paranasal sinus cancers, critical normal tissues that may be partly spared using IMRT are the inner and middle ears, the temporomandibular joints, temporal brain lobes, and the optic pathways.
Defining the Target: Imaging
The simulation contrast-enhanced CT is in most cases the only imaging modality required for the delineation of the targets. Magnetic resonance imaging (MRI) is a necessary adjunct to CT for tumors close to the base of skull, such as nasopharyngeal and paranasal sinus cancer, where it provides better details of tumor extension and of the parapharyngeal and retropharyngeal spaces than CT.77,78 Another potential imaging modality for this purpose is fluorodeoxyglucose-positron emission tomography (FDG-PET). However, in a series of HNCs in which CT, MRI, and FDG-PET were obtained, and surgery was then performed to validate the primary tumor extent and lymph node involvement, a rather limited benefit of FDG-PET over CT and MRI was found.79 PET-derived gross tumor volumes were smaller than those derived from CT and MRI, and surgical specimens were even smaller. However, when examined in detail, despite overestimation in most dimensions, all three imaging modalities actually underestimated the mucosal extent of disease.79 Therefore, physical examination and laryngoscopy findings should be part of the definition in addition to image modalities such as CT and PET.
Selection and Outlining of the Targets
Selection and Delineation of the Lymphatic Clinical Target Volumes
Our knowledge of the pattern and risk of lymphatic drainage from different head and neck sites is based on the classic anatomical work of Rouviere,80 which has been reviewed,81 the assessment of the location and prevalence of clinical neck metastasis by Lindberg82; and the large experience with elective neck dissections providing information about microscopic metastases, which was reported by Byers and colleagues83 and Shah.84 A division of the neck into six levels has been developed by surgeons from Memorial Hospital and revised by Robbins and associates.85,86 The retropharyngeal nodes, which are not routinely dissected surgically, are not considered in the surgical neck levels classification but are important targets in the irradiation of nasopharyngeal and other advanced HNCs.87
The Planning Target Volumes
Once the GTVs and CTVs are outlined on the axial CT scans, a uniform expansion of these targets is performed to obtain the PTVs that accommodate setup uncertainties. Typically the magnitude of the margin is 3 to 5 mm, which means that an extra 5-mm ring of normal tissue around the target receives full dose. In a region with targets and organs at risk in close proximity, reducing this margin can potentially reduce treatment-related toxicity. Indeed, it has been estimated that each 1 mm of margin adds 1.3 Gy of dose to the parotid gland, which is particularly sensitive to low doses of radiation.88 In order to reduce setup uncertainty, many groups have advocated daily imaging, with a position correction if the displacement from the original plan exceeds a threshold value. An example of the delineation of the targets and noninvolved structures in a case of oropharyngeal cancer is provided in Figure 77-12. The delivery of a single IMRT plan throughout the course of treatment provides better dose conformality than the use of several consecutive plans common in conventional radiotherapy, which consist of initial fields encompassing all targets followed with a boost to the gross tumor.89 When a single plan is prescribed, the gross tumor PTV receives both a higher total dose and a higher dose per fraction than the PTVs of the subclinical disease. Owing to the differences in the daily fraction doses, a correction of the total dose to yield the normalized total dose for a 2-Gy fraction regimen is required. Therefore a standard IMRT plan would include 70 Gy over 35 fractions to the GTV, and lower fraction doses to the subclinical disease PTVs: 63 Gy to the high-risk and 56 to 59 Gy to lesser-risk elective targets, over 35 fractions (1.8 Gy and 1.6-1.7 Gy).
The Optimization Process: The Number and Directions of the Beams
Although tomotherapy uses arcs around the patient, IMRT using multileaf collimators requires decisions regarding the number and orientation of the beams. It was suggested early on that if the number of segments (or beamlets) is large enough, the direction of the beams is not important, and coplanar beams arranged at equal distances around the patient’s head and neck would achieve satisfactory results. Most investigations of IMRT of the head and neck with multileaf collimators use this approach. The beam number should be odd, to prevent opposed beams, which would increase hot spots near their entrances to the neck. Nine beams arranged equidistantly (40 degrees apart) were found to be optimal: They provided better dose distributions than five or seven beams, whereas fifteen beams did not seem to improve the plans.90
Clinical Results
Nasopharynx
The use of IMRT for the nasopharynx represents opportunities to spare many critical noninvolved structures and to improve tumor coverage, as detailed previously. These improvements have been demonstrated in several treatment planning exercises in nasopharyngeal and oropharyngeal tumors, in which IMRT plans were compared with “standard 3D” plans in the same patients.91,92
Two randomized prospective studies compared IMRT treatment with conventional treatment in early stage nasopharyngeal cancer.93,94 Both showed similar high rates of tumor control and a benefit for IMRT for sparing of salivary flow. One of these studies demonstrated a benefit for IMRT in patient-related xerostomia.93
A large clinical series of IMRT of nasopharyngeal cancer has been reported by investigators at the University of California in San Francisco.95 They studied 67 patients treated in the years 1995 to 2000. The GTV dose was 1.12 to 2.25 Gy/fraction to a total of 65 to 70 Gy, and the CTV dose was 1.8 to 2.0 Gy/fraction to a total of 50 to 60 Gy. In order to prevent under-dosing of the targets, the prescribed doses were typically the minimal doses encompassing the targets. The resulting delivered mean GTV dose was 74.5 Gy, at mean GTV fraction doses of 2.24 to 2.4 Gy. The resulting normalized total dose (calculated for late-responding tissue) was 80 Gy. This regimen yielded excellent locoregional tumor control: The rate was 97% at median follow-up of 31 months, with reasonable rates of acute toxicity. For re-irradiation of nasopharyngeal cancer, IMRT offers dose distribution advantages because it significantly reduces the extent of irradiation of the previously irradiated tissue volume. Lu and coworkers96 described their experience with re-irradiation using IMRT for recurrent nasopharyngeal cancer. The rate of acute toxicity was acceptable, and at a median follow-up of 9 months, the locoregional control rate was 100%. Although longer follow-up is necessary, the preliminary toxicity and local control data for these recurrent cases are promising.96
Paranasal Sinuses
The main obstacle to adequate irradiation of tumors of the paranasal sinuses is the proximity of the optic pathways to the target in advanced cases. In these cases, IMRT can provide adequate target coverage while sparing the optic pathways (Fig. 77-13).
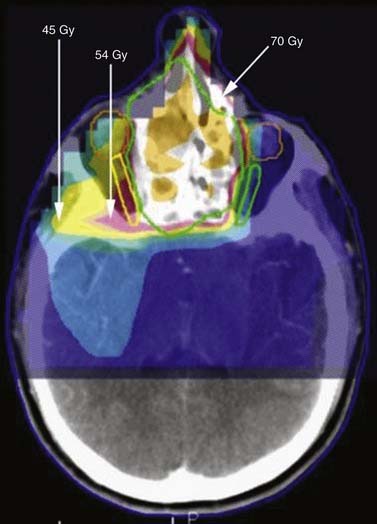
Figure 77-13. Radiation of sinuses with sparing of the optic nerves. Green represents GTV, to Gy dose; yellow is US Gy; and pink is SU Gy.
The group from the Royal Marsden Hospital found that compared with 3DCRT using the same beam arrangement, IMRT significantly reduced the dose to the optic nerves and improved PTV coverage.97 Tsien and colleagues98 at the University of Michigan found that IMRT planning in patients with nonresectable maxillary sinus cancer revealed no substantial difference between plans using an anterior field and two lateral fields and plans using nine equidistant beams arranged around the patient. Optimization using normal tissue complication probability (NTCP) of the optic nerves as an objective function was found to facilitate planning and to provide a basis for the assessment of the clinical trade-off between PTV coverage and ipsilateral optic nerve sparing.98
A large clinical series of IMRT for paranasal sinuses was reported from Ghent University in Belgium.99 39 patients were treated postoperatively, and the 4-year actuarial local control after surgery and IMRT was more than 80% for patients with T1-4aN0M0 disease. In the group undergoing IMRT, there was significantly lower incidence of severe dry-eye syndrome than in the historical cohort.
Oropharynx
In general, all series investigating IMRT for oropharyngeal cancer have reported outstanding locoregional control rates.92,100 These series reported 2-year locoregional tumor control rates of 90% to 98% for patient populations who mainly had stage III or IV tumors. However, these results should be evaluated in the context of a likely selection bias and, importantly, with consideration of the emergence of human papillomavirus–related oropharyngeal cancer, which is associated with better prognosis.101
Some of the most reliable information gained from clinical series of IMRT for HNC in general, and oropharyngeal cancer in particular, relates to the pattern of tumor recurrences relative to the targets and the locally delivered doses. These data allow an assessment of the adequacy of target selection and delineation. In all reported cases, it seems that careful selection and delineation of the targets resulted in very few or no marginal or out-of-field recurrences. De Arruda and colleagues100 reported that all recurrences in their series were in-field. In the series by Chao and coworkers,102 most marginal recurrences were in the lower neck, which was treated with an anterior field that was matched to the IMRT-treated upper neck. In the series reported by Eisbruch and associates,87 in which the majority of patients had oropharyngeal cancer, almost all recurrences were in-field, in high-risk volumes that had received the full prescribed doses.
Larynx and Hypopharynx
IMRT can improve target dose homogeneity for laryngeal and hypopharyngeal SCC while reducing the dose to the normal tissues at risk.103–105 Clinical data on IMRT for laryngeal and hypopharyngeal SCC are scarce, however, and include limited numbers of patients within large heterogeneous series of multiple HN tumor sites.87,95,105 In general, these series showed lower tumor control rates than those reported for oropharyngeal sites. Potential reasons could be patient selection factors. For example, at the University of Michigan, only patients with laryngeal cancer and advanced nodal disease receive IMRT. The reason is that for patients with nodal stage 0 to 1, the uppermost nodes at level II are the subdigastric nodes, which can be treated with conventional techniques while sparing the majority of the parotid glands and avoiding the oral cavity; the retropharyngeal nodes or level II near the base of skull are not at risk in these patients. Another small study has suggested a benefit in xerostomia without a compromise in local control (94% at 2 years) in advanced node-negative laryngeal cancer.106
In general the main intent in IMRT planning is preservation of function. One of the main issues is reducing xerostomia via the sparing of the parotid salivary glands and submandibular glands. According to one study, the mean dose of 26 Gy is the threshold for parotid gland sparing (see Fig. 77-12),107 and patients in whom at least one functioning parotid gland was spared enjoyed significant preservation of salivary flow and reduced xerostomia.
Other potential functional gains from IMRT compared with conventional RT include reduced long-term dysphagia. Sparing of pharyngeal constrictors and the glottic and supraglottic larynx may be beneficial in this regard.108
Proton Therapy
Currently, all centers utilize photons, or high-energy x-rays, for their IMRT treatments. However, because of their inherent physical characteristics, protons are better able to concentrate dose inside targets and minimize dose to surrounding normal tissues. Most of the energy of a proton beam is deposited near the end of the beam path (Bragg peak), the location of which is determined by the energy of the beam. Thus, the target can be included in the tissue volume receiving the high dose while little is delivered before or after the beam passes through the target, and the integral dose delivered outside the targets is lower than the dose delivered using IMRT. Use of proton beams would be expected to reduce the risk of secondary malignancies, which is especially important in pediatric cancer patients.109 With the use of inverse planning, intensity-modulated proton therapy (IMPT) can further improve the therapeutic index of radiotherapy. Several groups have published treatment planning comparisons of (photon) IMRT with IMPT.110–112 Using IMPT, mean doses to the organs at risk—the parotid glands, in particular—have been reduced by as much as 50%. However, because IMPT has been used for only a few years, long-term clinical treatment results are not yet available. The areas in the head and neck that could benefit from the added conformality achievable with protons are the paranasal sinuses and nasopharynx, which are often in close proximity to the optic nerves and chiasm, as well as the brain. Retrospective reviews of 3D conformal proton therapy suggest high local control rates with minimal toxicity, including preservation of vision in patients with advanced sphenoid sinus cancers.113
Neutron and Carbon Ion Therapy
In addition to protons, other particles have been used to treat HNCs. Neutrons have found a niche in treatment of salivary gland tumors, after a small trial randomly assigning patients to photon or neutron radiotherapy demonstrated a survival benefit for neutron therapy.114 Other ions, including helium, neon, and carbon, have been investigated over the past 50 years. Currently, carbon ion therapy shows the most promise. Compared with photons, carbon ions have the conformality advantage of protons, with the additional advantage of a greater relative biologic efficacy (RBE): A smaller dose of carbon ions is required to achieve the same biologic effect as a reference dose of photons. This difference is due to a higher linear energy transfer with carbon ions, which theoretically causes more irreparable DNA damage, is more effective in hypoxic cells, and is less subject to variation in radiosensitivity with cell cycle. Currently, few centers worldwide are able to treat with this modality. Initial results in adenoid cystic carcinoma and locally advanced SCC of the head and neck, which demonstrate excellent local control rates with low toxicities, are promising.115 However, long-term results are not yet available.
Imaging-Guided Radiotherapy
Tracking Anatomic Changes during Therapy
During the 6- to 7-week course of radiotherapy, many anatomic changes can occur. Because dose distributions achieved using IMRT have steep gradients, and PTV “safety” margins are becoming smaller, it is especially important to understand the consequences of anatomic changes during therapy for the dose to both targets and normal tissues. In definitive cases, the primary tumor, nodes, or both can shrink, whereas in postoperative cases, inflammation and edema can resolve. Additionally, weight loss, a common consequence of acute treatment toxicity, can lead to muscle wasting and shifting of both normal tissue and tumor positions. Barker and associates116 conducted a detailed study of such anatomic changes during radiotherapy for HNC.116 The primary tumors and nodes were noted to shrink at a median rate of 1.8% of the initial volume per treatment day, so that on the last day of treatment, the median overall volume loss was close to 70%. The parotid glands moved medially during treatment, the median displacement at the end of therapy being 3.1 mm. These changes were highly correlated with patient weight loss. After publication of these findings, several investigators suggested replanning during treatment to minimize parotid gland dosage while maximizing tumor dosage. This issue is subject to ongoing studies.
Brachytherapy
As described previously, brachytherapy is localized radiotherapy that uses many radioactive isotopes, such as radium Ra-226 and cesium Cs-137 needles for implants in certain cases or afterloading techniques using iridium Ir-192 sources.117 These sources produce a lower-energy gamma ray, thus simplifying the radiation protection requirements associated with routine patient care. The sources are left in place for a specified time and then are removed. Alternatively, permanent implants using gold Au-198 and iodine I-125 can be used. These implants deliver their total radiation dose over the effective lifetime of the radioactive material. The obvious advantages of implants are better dose localization, which results in less radiation damage to the healthy tissue surrounding the tumor, and the relatively prolonged time over which the radiation is delivered. The disadvantages include lack of addressing subclinical disease and the requirement for general anesthesia in many cases.
External-beam radiation is given at the rate of 1.5 to 2.0 Gy per minute.118 A typical Ir-192 implant delivers its dose at the rate of 0.4 to 0.8 Gy per hour. High-dose-rate (HDR) remote afterloading devices have also been developed. These devices push a single, high-activity Ir-192 source through a set of interstitial catheters, and a computer program controls the source dwell time at various points throughout the implant. Typically, about 3.0 to 3.5 Gy is given to a distance of about 1 cm from the periphery of the catheters each treatment, and up to two daily treatments are given about 6 hours apart. Each treatment takes about 15 to 30 minutes, depending on the strength of the radioactive source and the complexity of the implant.
Radiation Side Effects
Acute Side Effects
Acute complications can interfere with and delay treatment. Even short delays can be deleterious, interfering with local tumor control.119,120 In one study, for example, a 5-day delay in completing RT was associated with a 3.5% to 8% reduction in local control for laryngeal cancers.120 The effect was greater in tumors with a higher probability of local failure. In addition to treatment-related factors, the nature, frequency, and severity of complications are heavily influenced by tumor-related factors (e.g., location, invasion of vital structures) and patient-related factors (e.g., oral hygiene, nutritional status, continued tobacco use, and history of diabetes, collagen vascular disease or human immunodeficiency virus).
The following sections discuss the most common acute side effects:
Xerostomia—
Prevention of this side effect can be performed by reducing salivary gland dosage in formal planning. Some suggested that the use of amifostine may reduce xerostomia. The benefit of amifostine was shown in a landmark trial of 315 patients receiving RT alone (60 to 70 Gy in 1.8- to 2.0-Gy daily fractions), who were randomly assigned to receive either amifostine or no amifostine.121 Amifostine reduced the incidence of significant acute xerostomia from 78% to 51% and the incidence of significant chronic xerostomia at 12 months from 57% to 34%. This agent had no impact on rates of mucositis or on tumor control at 2 years.121 However, data on the effect of amifostine in the chemoradiation setup are conflicting,122,123 other than that it has severe side effects and is expensive and therefore has not been widely used.
Mucositis
Radiation-induced loss of stem cells in the basal layer interferes with the replacement of cells in the superficial mucosal layers when they are lost through normal physiologic sloughing. The subsequent denuding of the epithelium results in mucositis, which can be painful and can interfere with food intake and nutrition. Mucositis usually develops 2 to 3 weeks after the start of RT. The incidence of mucositis is variable, depending on the field, the total dose and duration of RT, and the concomitant administration of chemotherapy.124 In one series, clinically significant mucositis occurred in 64% of patients receiving RT to the head or neck for any malignancy.125 Both incidence and severity are higher in patients receiving chemoradiotherapy than in those undergoing RT alone. Chemotherapy can have a similar effect on the mucosa, and the combination of chemotherapy with RT increases the rate of mucositis especially when the modalities are delivered concurrently. Mucositis is managed symptomatically with scrupulous oral hygiene, dietary modification, and topical anesthetics. A variety of agents have been evaluated for use either prophylactically or after the onset of symptoms; their role in the treatment of radiation-induced mucositis is uncertain (Fig. 77-14).
Late Toxicities
Xerostomia
Xerostomia is a frustrating side effect that may lead to many other effects. It often improves with time,126 but it can be long-lasting or even permanent. Regarding treatment, several strategies may minimize the incidence of xerostomia. When possible, sparing one parotid gland and, if possible, the submandibular glands can greatly diminish the incidence of xerostomia.127 Commercially available salivary substitutes or artificial saliva (oral rinses containing hyetellose, hyprolose, or carmellose) relieve the discomfort of xerostomia by temporarily wetting the oral mucosa. Although they can provide temporary relief, especially prior to eating, many patients must take frequent sips of water to remain comfortable. In addition to being inconvenient, this can lead to secondary problems such as nocturia from late night fluid intake in men with prostatic hypertrophy and in men and in women with small bladder capacity. In patients in whom untreated or unaffected residual salivary tissues are intact, sialagogues that stimulate salivary production may be used. Sialagogues can be characterized as gustatory, tactile, or pharmacologic. Gustatory stimuli such as acidic or bitter substances are most effective at stimulating salivary flow.128 Sweet substances such as sugar-free hard candy also stimulate the flow of saliva, but to a lesser extent. Pharmacologic sialagogues, which are typically agonists of the muscarinic (M3) receptor, include pilocarpine and cevimeline. Of these, pilocarpine has been the most extensively investigated. Pilocarpine may improve or prevent xerostomia when given after completing radiotherapy. Improvement in symptoms may occur only after many weeks of treatment, and cholinergic side effects, in particular sweating, may stop a significant number of patients from continuing to use the drug.128
Osteoradionecrosis
Mandibular ORN occurs in 5% to 10% of patients treated with conventional RT or high-dose-rate brachytherapy, and is severe in about 2%.129,130 The incidence of this complication appears to be higher with hyperfractionated regimens, especially with a short inter-fraction interval. In one study of 168 patients with oral cancer treated with hyperfractionated or conventional RT, the incidence of ORN was higher with hyperfractionated therapy (23% vs. 9%, respectively).130 Tooth extraction and dental disease in irradiated regions are major factors in the development of both mandibular and maxillary ORN.129,130 Although opinion differs as to whether extraction of diseased and nonrestorable teeth should be performed before or after irradiation, many writers report that postirradiation extraction of such teeth produces a higher rate of mandibular ORN.131 Furthermore, at least some data suggest that mandibular ORN associated with postirradiation tooth extraction more often requires radical resection than does ORN that develops after pre-irradiation extraction (45% vs. 12%, respectively).132 Repair of nonrestorable and diseased teeth prior to RT may reduce the risk of this complication. However, most authorities do not recommend the pre-irradiation extraction of healthy or restorable teeth.
Ben-David and colleagues133 demonstrated that rates of ORN could be significantly lower if the following rules were observed: reducing the mandibular volumes receiving high doses, improved salivary flow rates and associated improved oral health, and uniform prophylactic dental care. For mild ORN, treatment with conservative débridement, antibiotics, and occasionally ultrasound is usually successful.134 However, when bone and soft tissue necrosis are extensive, radical resection of the mandible with immediate microvascular reconstruction may provide better results.135 Persistence of ORN despite aggressive treatment should raise the suspicion of recurrent cancer.135
Hyperbaric oxygen therapy (HBO) has been suggested as a beneficial therapeutic maneuver in patients in whom ORN of the jaw develops after RT. ORN may be triggered by a predominantly fibro-atrophic mechanism.136 At least in theory; HBO simulates the function of monocytes and fibroblasts, increasing collagen synthesis and vascular density. Despite this theoretical rationale, the available data are conflicting, as to clinical benefit, of HBO for both prevention and therapy of ORN. The benefit from HBO was called into question by results of a randomized multicenter trial in which 68 patients with overt mandibular ORN were randomly assigned to receive either 30 to 40 exposures to HBO or a placebo at 2.4 absolute atmospheres for 90 minutes.137 Healing rates were equivalent in the two groups and in fact trended toward worse in the HBO group (19%, compared with 32% for placebo; P = .23). Although this was only a single study and did not include patients receiving HBO prior to dental extraction, its results raise doubt about the efficacy of HBO for ORN.
Thyroid Dysfunction
Irradiation of the low neck is associated with hypothyroidism. The incidence varies widely and is dose-dependent as well as time dependent, increasing with time elapsed since treatment. RT-induced hypothyroidism develops at a median of 1.4 to 1.8 years (range 0.3 to 7.2 years) after RT.138 It is more common in patients undergoing both neck surgery and RT but is not higher in patients who undergo chemotherapy in addition to RT than in those who have RT alone.
Vascular Complications
Carotid artery rupture (also called carotid blowout syndrome) and oropharyngocutaneous fistula are major complications associated with RT to the neck. These sequelae occur almost exclusively in patients who have received combined surgery and RT. As an example, in one series of 46 patients with carotid artery rupture, 43 had undergone extensive primary and salvage radical surgery with intraoperative brachytherapy, external beam irradiation, or both.119 Both retrospective and prospective studies show that both carotid artery rupture and oropharyngocutaneous fistula occur more often with preoperative than postoperative irradiation.
Carotid rupture and fistula are rarely observed after definitive RT with or without concomitant chemotherapy but may be more common when tumor is invading the carotid sheath or extending into and occupying a large volume of the soft tissue of the neck.139 Patients with ORN of the temporal bone after definitive treatment for nasopharyngeal cancer are also at risk for carotid artery rupture.
The most common angiographic abnormality seen in one study was one or more pseudoaneurysms.140 Although this devastating complication can sometimes be managed with endovascular therapy, it recurs in up to 25% of patients, often as a manifestation of recurrent tumor or of multifocal iatrogenic arteriopathy and occasionally wound complications.
Neurologic Complications
Radiation myelopathy may begin acutely, but more often the onset is insidious. Paresis, numbness, and sphincter dysfunction developing 6 to 12 months after irradiation are the typical manifestations of progressive radiation-induced myelitis. However, the initial signs can be subtle, such as decreased temperature sensation and decreased proprioception. These signs may stabilize or slowly progress inexorably to lower extremity weakness, footdrop, the Brown-Séquard syndrome, incontinence, hyperreflexia, loss of bowel and bladder function, and complete paresis below the irradiated section of the spinal cord. The risk of chronic radiation myelopathy rises progressively with the dose of radiation.141 Evidence derived from several large series suggests that the incidence of myelopathy is less than 0.5% after 50 Gy with conventional fractionation.141 The best estimate of the dose expected to cause a 5% risk of myelopathy within 5 years (sometimes called the TD 5/5) is approximately 60 Gy if the radiation is given in 1.8- to 2.0-Gy daily fractions. This dose value appears to be independent of the region or length of spinal cord treated. The risk of myelopathy increases rapidly with doses above 60 Gy, with one series reporting an incidence of 50% in patients receiving between 68 and 73 Gy to the spinal cord.141
Bernier J, Cooper JS, Pajak TF, et al. Defining risk levels in locally advanced head and neck cancers: a comparative analysis of concurrent postoperative radiation plus chemotherapy trials of the EORTC (#22931) and RTOG (#9501). Head Neck. 2005;27:843.
Bernier J, Denekamp J, Rojas A, et al. ARCON: accelerated radiotherapy with carbogen and nicotinamide in head and neck squamous cell carcinomas; the experience of the Co-operative Group of Radiotherapy of the European Organization for Research and Treatment of Cancer (EORTC). Radiother Oncol. 2000;55:111.
Bernier J, Domenge C, Ozsahin M, et al. Postoperative irradiation with or without concomitant chemotherapy for locally advanced head and neck cancer. N Engl J Med. 2004;350:1945.
Bonner JA, Harari PM, Giralt J, et al. Radiotherapy plus cetuximab for squamous-cell carcinoma of the head and neck. N Engl J Med. 2006;354:567.
Bourhis J, Lapeyre M, Tortochaux J, et al. Phase III randomized trial of very accelerated radiation therapy compared with conventional radiation therapy in squamous cell head and neck cancer: a GORTEC trial. J Clin Oncol. 2006;24(18):2873-2878.
Bourhis J, Overgaard J, Audry H, et al. Hyperfractionated or accelerated radiotherapy in head and neck cancer: a meta-analysis. Lancet. 2006;368:843.
Brizel DM, Albers ME, Fisher SR, et al. Hyperfractionated irradiation with or without concurrent chemotherapy for locally advanced head and neck cancer. N Engl J Med. 1998;338:1798.
Coleman CN. Hypoxia in tumors: a paradigm for the approach to biochemical and physiologic heterogeneity. J Natl Cancer Inst. 1988;80:310.
Cooper JS, Pajak TF, Forastiere AA, et al. Postoperative concurrent radiotherapy and chemotherapy for high-risk squamous-cell carcinoma of the head and neck. N Engl J Med. 2004;350:1937.
Eisbruch A, Marsh LH, Dawson LA, et al. Recurrences near the base of the skull following IMRT of head and neck cancer: implications for target delineation in the high neck, and for parotid sparing. Int J Radiat Oncol Biol Phys. 2004;59:28-42.
Eisbruch A, Schwartz M, Rasch C, et al. Dysphagia and aspiration after chemoradiotherapy for head-and-neck cancer: which anatomic structures are affected and can they be spared by IMRT? Int J Radiat Oncol Biol Phys. 2004;60:1425-1433.
Eisbruch A, Ship JA, Martel MK, et al. Parotid gland sparing in patients undergoing bilateral head and neck irradiation: techniques and early results. Int J Radiat Oncol Biol Phys. 1996;36:469-480.
Eisbruch A, Ten Haken R, Kim HM, et al. Dose, volume and function relationships in parotid glands following conformal and intensity modulated irradiation of head and neck cancer. Int J Radiat Oncol Biol Phys. 1999;45:577-587.
Forastiere AA, Goepfert H, Maor M, et al. Concurrent chemotherapy and radiotherapy for organ preservation in advanced laryngeal cancer. N Engl J Med. 2003;349:2091.
Fu KK, Pajak TF, Trotti A, et al. Radiation Therapy Oncology Group (RTOG): A phase III randomized study to compare hyperfractionation and two variants of accelerated fractionation to standard fractionation radiotherapy for head and neck squamous cell carcinomas: first report of RTOG 9003. Int J Radiat Oncol Biol Phys. 2000;48:7-16.
Hall EJ. Radiobiology for the Radiologist. Philadelphia: JB Lippincott; 1994.
Hitt R, Lopez-Pousa A, Martinez J, et al. Phase III study comparing cisplatin plus fluorouracil to paclitaxel, cisplatin and fluorouracil induction chemotherapy followed by chemoradiotherapy in locally advanced head and neck cancer. J Clin Oncol. 2005;23:8636-8645.
Kam MK, Leung SF, Zee B, et al. Prospective randomized study of intensity-modulated radiotherapy on salivary gland function in early-stage nasopharyngeal carcinoma patients. J Clin Oncol. 2007;25:4873-4879.
Khan FM. The Physics of Radiation Therapy: Mechanisms, Diagnosis, and Management, 3rd ed. Philadelphia: Lippincott Williams & Wilkins; 2003.
Khan FM, Potish RA, editors. Treatment Planning in Radiation Oncology. Philadelphia: Lippincott, 1998.
Pignon JP, Bourhis J, Domenge C, et al. Chemotherapy added to locoregional treatment for head and neck squamous-cell carcinoma: three meta-analyses of updated individual data. Lancet. 2000;355:949.
Posner MR, Hershock DM, Blajman CR, et al. TAX 324 Study Group. Cisplatin and fluorouracil alone or with docetaxel in head and neck cancer. N Engl J Med. 2007;357(17):1705-1715.
Prise KM, Schettino G, Folkard M, et al. New insights on cell death from radiation exposure. Lancet Oncol. 2005;6(7):520-528.
Ritchie JM, Smith EM, Summersgill KF, et al. Human papillomavirus infection as a prognostic factor in carcinomas of the oral cavity and oropharynx. Int J Cancer. 2003;104:336.
Robbins KT, Medina JE, Wolfe GT, et al. Standardizing neck dissection terminology.: official report of the Academy’s Committee for Head and Neck Surgery and Oncology. Arch Otolaryngol Head Neck Surg. 1991;117:601-605.
Som PM. The present controversy over the imaging method of choice for evaluating the soft tissues of the neck. AJNR Am J Neuroradiol. 1997;18:1869-1872.
The Department of Veterans Affairs Laryngeal Cancer Study Group. Induction chemotherapy plus radiation compared with surgery plus radiation in patients with advanced laryngeal cancer. N Engl J Med. 1991;324:1685.
1. Rossi HH, Kellerer AM. Roentgen. Radiat Res. 1995;144:124-128.
2. Glasser O. Roentgen, 2nd ed. Springfield, IL: Charles C Thomas; 1958.
3. Myers WG. Becquerel’s discovery of radioactivity in 1896. J Nucl Med. 1976;17:579-582.
4. Curie P, Curie M, Bemont G. Sur une nouvelle substance fortement radioactive contenue dans la pechblende (note presented by M Becquerel). Compt Rend Acad Sci (Paris). 1898;127:1215.
5. A review of the history of radiation therapy. http://www.xray.hmc.psu.edu/rci/ss9/ss9_1.html.
6. Del Regato J. The American Radium Society: its diamond jubilee. Am J Clin Oncol. 1991;14:93-100.
7. Brenner DJ. Radiation biology in brachytherapy. J Surg Oncol. 1997;65:66-70.
8. Del Regato JA. Fractionation: a panoramic view. Int J Radiat Oncol Biol Phys. 1990;19:1329-1331.
9. Houlthusen H. The present status of dosage measurements. Radiology. 1928;10:292.
10. Khan FM. The Physics of Radiation Therapy: Mechanisms, Diagnosis, and Management, 3rd ed. Philadelphia: Lippincott Williams & Wilkins; 2003.
11. Khan FM, Potish RA, editors. Treatment Planning in Radiation Oncology. Philadelphia: Lippincott, 1998.
12. Duncan W, Arnott SJ, Battermann JJ, et al. Fast neutrons in the treatment of head and neck cancers: the results of a multi-centre randomly controlled trial. Radiother Oncol. 1984;2:293.
13. Griffin TW, Davis R, Hendrickson FR, et al. Fast neutron radiation therapy for unresectable squamous cell carcinomas of the head and neck: the results of a randomized RTOG study. Int J Radiat Oncol Biol Phys. 1984;10:2217.
14. Jereczek-Fossa BA, Krengli M, Orecchia R. Particle beam radiotherapy for head and neck tumors: radiobiological basis and clinical experience. Head Neck. 2006;28(8):750-760.
15. Hall EJ. Radiobiology for the Radiologist. Philadelphia: JB Lippincott; 1994.
16. Ward JF. DNA damage produced by ionizing radiation in mammalian cells: identities, mechanisms of formation, and reparability. Prog Nucleic Acid Res Mol Biol. 1988;35:95-125.
17. Brenner DJ, Ward JF. Constraints on energy deposition and target size of multiply damaged sites associated with DNA double-strand breaks. Int J Radiat Biol. 1992;61:737-748.
18. Powell S, McMillan TJ. DNA damage and repair following treatment with ionizing radiation. Radiother Oncol. 1990;19:95-108.
19. Prise KM, Schettino G, Folkard M, et al. New insights on cell death from radiation exposure. Lancet Oncol. 2005;6(7):520-528.
20. Aneja N, Tjalkens R, Philbert MA, et al. Irradiation of mitochondria initiates apoptosis in a cell free system. Oncogene. 2001;20(2):167-177.
21. Kastan MB, Onyekwere O, Sidransky D, et al. Participation of p53 protein in the cellular response to DNA damage. Cancer Res. 1991;51(23):6304-6311.
22. Beck-Bornholdt HP, Dubben HH, Willers H. [Proliferation rate and radiosensitivity: Bergonié’s and Tribondeau’s error]. Strahlenther Onkol. 1997;173(6):335-337.
23. Puck TT, Markus PI. The action of x-rays on mammalian cells. J Exp Med. 1956;103:653-666.
24. Steel G. Basic Clinical Radiobiology, 3rd ed. London: Arnold; 2002.
25. Keller AM, Rossi HH. The theory of dual radiation action. Curr Top Radiat Res Q. 1972;8:85.
26. Chadwick K, Leenhouts H. A molecular theory of cell survival. Phys Med Biol. 1973;18:78.
27. Thames H, Hendry J. Fractionation in Radiotherapy. London: Taylor & Francis; 1987.
28. Peracchia G, Salti C. Radiotherapy with thrice-a-day fractionation in a short overall time: clinical experiences. Int J Radiat Oncol Biol Phys. 1981;7:99-104.
29. van den Bogaert W, van der Schueren E, Horiot JC, et al. Early results of the EORTC randomized clinical trial on multiple fractions per day (MFD) and misonidazole in advanced head and neck cancer. Int J Radiat Oncol Biol Phys. 1986;12(4):587-591.
30. Gragg RL, Humphrey RM, Thames HDJr, Meyn RE. The response of Chinese hamster ovary cells to fast neutron radiotherapy beams. III. Variation in biological effectiveness with position in the cell cycle. Radiat Res. 1978;76:283-291.
31. Tomlinson R, Gray L. The historical structure of some human lung cancers and the possible implications for radiotherapy. Br J Cancer. 1955;9:539.
32. Strandquist M. Studien uber die Kumulative urkung der roentgenstrahlen bei fractionerung. Acta Radiol Suppl. 1944;55:1.
33. Ellis F. Fractionation in radiotherapy. In: Delly T, Wood C, editors. Modern Trends in Radiotherapy, Vol 1. London: Butterworth; 1967.
34. Fu KK, Pajak TF, Trotti A, et al. A Radiation Therapy Oncology Group (RTOG) phase III randomized study to compare hyperfractionation and two variants of accelerated fractionation to standard fractionation radiotherapy for head and neck squamous cell carcinomas: first report of RTOG 9003. Int J Radiat Oncol Biol Phys. 2000;48(1):7-16.
35. Horiot JC, Le Fur R, N’Guyen T, et al. Hyperfractionation versus conventional fractionation in oropharyngeal carcinoma: final analysis of a randomized trial of the EORTC cooperative group of radiotherapy. Radiother Oncol. 1992;25(4):231-241.
36. Pinto, LH, Canary PC, Araujo CM, et al. Prospective randomized trial comparing hyperfractionated versus conventional radiotherapy in stages III and IV oropharyngeal carcinoma. Int J Radiat Oncol Biol Phys. 1991;21(3):557-562.
37. Cummings B, O’Sullivan B, Keane T, et al. 5-year results of 4 week/twice daily radiation schedule-the Toronto trial (abstract). Radiother Oncol. 2000;56(Suppl):S8.
38. Bourhis J, Lapeyre M, Tortochaux J, et al. Phase III randomized trial of very accelerated radiation therapy compared with conventional radiation therapy in squamous cell head and neck cancer: a GORTEC trial. J Clin Oncol. 2006;24(18):2873-2878.
39. Skladowski, K, Maciejewski B, Golen M, et al. Continuous accelerated 7-days-a-week radiotherapy for head-and-neck cancer: long-term results of phase III clinical trial. Int J Radiat Oncol Biol Phys. 2006;66(3):706-713.
40. Dobrowsky W, Naude J. Continuous hyperfractionated accelerated radiotherapy with/without mitomycin C in head and neck cancers. Radiother Oncol. 2000;57(2):119-124.
41. Dische S, Saunders M, Barrett A, et al. A randomised multicentre trial of CHART versus conventional radiotherapy in head and neck cancer. Radiother Oncol. 1997;44(Aug (2)):123-136.
42. Overgaard J, Hansen HS, Specht L, et al. Five compared with six fractions per week of conventional radiotherapy of squamous-cell carcinoma of head and neck: DAHANCA 6 and 7 randomised controlled trial. Lancet. 2003;362(9388):933-940.
43. Bourhis J, Overgaard J, Audry H, et al. Hyperfractionated or accelerated radiotherapy in head and neck cancer: a meta-analysis. Lancet. 2006;368:843.
44. Brizel DM, Albers ME, Fisher SR, et al. Hyperfractionated irradiation with or without concurrent chemotherapy for locally advanced head and neck cancer. N Engl J Med. 1998;338:1798.
45. Coleman CN. Hypoxia in tumors: a paradigm for the approach to biochemical and physiologic heterogeneity. J Natl Cancer Inst. 1988;80:310.
46. Gray LH, Conger AD, Ebert M, et al. The concentration of oxygen dissolved in tissues at the time of irradiation as a factor in radiotherapy. Br J Radiol. 1953;26:638.
47. Moulder JE, Rockwell S. Hypoxic fractions of solid tumors: experimental techniques, methods of analysis, and a survey of existing data. Int J Radiat Oncol Biol Phys. 1984;10:695.
48. Roh HD, Boucher Y, Kalnicki S, et al. Interstitial hypertension in carcinoma of uterine cervix in patients: possible correlation with tumor oxygenation and radiation response. Cancer Res. 1991;51:6695.
49. Prosnitz RG, Yao B, Farrell CL, et al. Pretreatment anemia is correlated with the reduced effectiveness of radiation and concurrent chemotherapy in advanced head and neck cancer. Int J Radiat Oncol Biol Phys. 2005;61:1087.
50. Haffty BG, Hurley R, Peters LJ. Radiation therapy with hyperbaric oxygen at 4 atmospheres pressure in the management of squamous cell carcinoma of the head and neck: results of a randomized clinical trial. Cancer J Sci Am. 1999;5:341.
51. Bernier J, Denekamp J, Rojas A, et al. ARCON: accelerated radiotherapy with carbogen and nicotinamide in head and neck squamous cell carcinomas: the experience of the Co-operative Group of Radiotherapy of the European Organization for Research and Treatment of Cancer (EORTC). Radiother Oncol. 2000;55:111.
52. Rischin D, Peters L, Hicks R, et al. Phase I trial of concurrent tirapazamine, cisplatin, and radiotherapy in patients with advanced head and neck cancer. J Clin Oncol. 2001;19:535.
53. Overgaard J, Hansen HS, Overgaard M, et al. A randomized double-blind phase III study of nimorazole as a hypoxic radiosensitizer of primary radiotherapy in supraglottic larynx and pharynx carcinoma. Results of the Danish head and Neck Cancer Study (DAHANCA) Protocol 5-85. Radiother Oncol. 1998;46:135.
54. Glaser CM, Millesi W, Kornek GV, et al. Impact of hemoglobin level and use of recombinant erythropoietin on efficacy of preoperative chemoradiation therapy for squamous cell carcinoma of the oral cavity and oropharynx. Int J Radiat Oncol Biol Phys. 2001;50:705.
55. Henke M, Laszig R, Rube C, et al. Erythropoietin to treat head and neck cancer patients with anaemia undergoing radiotherapy: randomised, double-blind, placebo-controlled trial. Lancet. 2003;362(9392):1255-1260.
56. Coughlin CT, Richmond RC. Biologic and clinical developments of cisplatin combined with radiation: concepts, utility, projections for new trials, and the emergence of carboplatin. Semin Oncol. 1989;16:31.
57. Lee I, Levitt SH, Song CW. Improved tumour oxygenation and radiosensitization by combination with nicotinamide and pentoxifylline. Int J Radiat Biol. 1993;64:237.
58. Rockwell S. Cytotoxicities of mitomycin C and x rays to aerobic and hypoxic cells in vitro. Int J Radiat Oncol Biol Phys. 1982;8:1035.
59. Kinsella TJ. Radiosensitization and cell kinetics: clinical implications for S-phase-specific radiosensitizers. Semin Oncol. 1992;19(Suppl 9):41.
60. Rosenthal DI, Carbone DP. Taxol plus radiation for head and neck cancer. J Infus Chemother. 1995;5:46.
61. Pignon JP, Bourhis J, Domenge C, et al. Chemotherapy added to locoregional treatment for head and neck squamous-cell carcinoma: three meta-analyses of updated individual data. Lancet. 2000;355:949.
62. Bernier J, Domenge C, Ozsahin M, et al. Postoperative irradiation with or without concomitant chemotherapy for locally advanced head and neck cancer. N Engl J Med. 2004;350:1945.
63. Rosenthal DI, Pistenmaa DA, Glatstein E. A review of neoadjuvant chemotherapy for head and neck cancer: partially shrunken tumors may be both leaner and meaner. Int J Radiat Oncol Biol Phys. 1994;28:315.
64. Hitt R, Lopez-Pousa A, Martinez J, et al. Phase III study comparing cisplatin plus fluorouracil to paclitaxel, cisplatin and fluorouracil induction chemotherapy followed by chemoradiotherapy in locally advanced head and neck cancer. J Clin Oncol. 2005;23:8636-8645.
65. Posner MR, Hershock DM, Blajman CR, et al. TAX 324 Study Group. Cisplatin and fluorouracil alone or with docetaxel in head and neck cancer. N Engl J Med. 2007;357(17):1705-1715.
66. Induction chemotherapy plus radiation compared with surgery plus radiation in patients with advanced laryngeal cancer. The Department of Veterans Affairs Laryngeal Cancer Study Group. N Engl J Med. 1991;324:1685.
67. Spaulding MB, Fischer SG, Wolf GT. Tumor response, toxicity, and survival after neoadjuvant organ-preserving chemotherapy for advanced laryngeal carcinoma. The Department of Veterans Affairs Laryngeal Cancer Study Group. J Clin Oncol. 1994;12:1592.
68. Forastiere AA, Goepfert H, Maor M, et al. Concurrent chemotherapy and radiotherapy for organ preservation in advanced laryngeal cancer. N Engl J Med. 2003;349:2091.
69. Forastiere AA, Maor M, Weber RS, et al. Long-term results of Intergroup RTOG 91-11: a phase III trial to preserve the larynx: induction cisplatin/5-FU and radiation therapy versus concurrent cisplatin and radiation therapy versus radiation therapy (abstract). J Clin Oncol. 2006;24:284s.
70. Cooper JS, Pajak TF, Forastiere AA, et al. Postoperative concurrent radiotherapy and chemotherapy for high-risk squamous-cell carcinoma of the head and neck. N Engl J Med. 2004;350:1937.
71. Bernier J, Cooper JS, Pajak TF, et al. Defining risk levels in locally advanced head and neck cancers: a comparative analysis of concurrent postoperative radiation plus chemotherapy trials of the EORTC (#22931) and RTOG (# 9501). Head Neck. 2005;27:843.
72. Bonner JA, Harari PM, Giralt J, et al. Radiotherapy plus cetuximab for squamous-cell carcinoma of the head and neck. N Engl J Med. 2006;354:567.
73. Pfister DG, Su YB, Kraus DH, et al. Concurrent cetuximab, cisplatin, and concomitant boost radiotherapy for locoregionally advanced, squamous cell head and neck cancer: a pilot phase II study of a new combined-modality paradigm. J Clin Oncol. 2006;24:1072.
74. Coia L, Galvin J, Sontag M, et al. Three dimensional photon treatment planning in carcinoma of the larynx. Int J Radiat Oncol Biol Phys. 1991;21:183-192.
75. Leibel S, Kutcher G, Harrison L, et al. Improved dose distributions for 3D conformal boost treatment in carcinoma of the nasopharynx. Int J Radiat Oncol Biol Phys. 1991;20:823-833.
76. Esik O, Schlegel W, Boesecke R, et al. Three dimensional radiotherapy planning for laryngeal and hypopharyngeal cancer. Radiother Oncol. 1991;20:238-244.
77. Roa WH, Hazuka MB, Sandler HM, et al. Results of primary and adjuvant CT-based 3-dimensional radiotherapy for malignant tumors of the paranasal sinuses. Int J Radiat Oncol Biol Phys. 1994;28:857-865.
78. Som PM. The present controversy over the imaging method of choice for evaluating the soft tissues of the neck. AJNR Am J Neuroradiol. 1997;18:1869-1872.
79. Schechter NR, Gillenwater AM, Byers RM, et al. Can positron emission tomography improve the quality of care for head and neck cancer patients? Int J Radiat Oncol Biol Phys. 2001;51:4-9.
80. Rouviere H. Lymphatic Systems of the Head and Neck. [Tobias MJ, trans]. Ann Arbor, MI: Edwards Brothers. 1938.
81. Mukherji SK, Armao D, Joshi VM. Cervical nodal metastases in squamous cell carcinoma of the head and neck: what to expect. Head Neck. 2001;23:995-1005.
82. Lindberg RD. Distribution of cervical lymph node metastases from squamous cell carcinoma of the upper respiratory and digestive tracts. Cancer. 1972;29:1446-1449.
83. Byers RM, Wolf PF, Ballantyne AJ. Rationale for elective modified neck dissection. Head Neck Surg. 1988;10:160-167.
84. Shah JP. Patterns of cervical lymph node metastasis from squamous carcinomas of the upper aerodigestive tract. Am J Surg. 1990;160:405-409.
85. Robbins KT, Medina JE, Wolfe GT, et al. Standardizing neck dissection terminology. Official report of the Academy’s Committee for Head and Neck Surgery and Oncology. Arch Otolaryngol Head Neck Surg. 1991;117:601-605.
86. Robbins KT. Integrating radiological criteria into the classification of cervical lymph node disease. Arch Otolaryngol Head Neck Surg. 1999;125:385-387.
87. Eisbruch A, Marsh LH, Dawson LA, et al. Recurrences near the base of the skull following IMRT of head and neck cancer: implications for target delineation in the high neck, and for parotid sparing. Int J Radiat Oncol Biol Phys. 2004;59:28-42.
88. van Asselen B, Dehnad H, Raaijmakers CP, et al. The dose to the parotid glands with IMRT for oropharyngeal tumors: the effect of reduction of positioning margins. Radiother Oncol. 2002;64:197-204.
89. Mohan R, Wu Q, Manning M, et al. Radiobiological considerations in the design of fractionation strategies for intensity modulated radiation therapy of the head and neck. Int J Radiat Oncol Biol Phys. 2000;46:619-630.
90. Wu Q, Manning M, Schmidt-Ullrich R, et al. The potential for sparing of parotids and escalation of biologically equivalent dose with intensity modulated radiation treatments of head and neck cancers: a treatment design study. Int J Radiat Oncol Biol Phys. 2000;46:195-205.
91. De Neve W, De Gersem W, Derycke S. Clinical delivery of IMRT for relapsed or second-primary head and neck cancer using a multileaf collimator with dynamic control. Radiother Oncol. 1999;50:301-314.
92. Yao M, Dornfeld KJ, Buatti JM, et al. Intensity modulated treatment for head and neck squamous cell carcinoma: the University of Iowa experience. Int J Radiat Oncol Biol Phys. 2005;63:410-421.
93. Kam MK, Leung SF, Zee B, et al. Prospective randomized study of intensity-modulated radiotherapy on salivary gland function in early-stage nasopharyngeal carcinoma patients. J Clin Oncol. 2007;25:4873-4879.
94. Pow EHN, Kwong DLW, McMillan AS, et al. Xerostomia and quality of life after intensity modulated radiotherapy vs conventional radiotherapy for early stage nasopharyngeal carcinoma: initial report on a randomized controlled trial. Int J Radiat Oncol Biol Phys. 2006;66:981-991.
95. Sultanem K, Shu HK, Xia P, et al. Three-dimensional intensity-modulated radiotherapy in the treatment of nasopharyngeal carcinoma: the University of California-San Francisco experience. Int J Radiat Oncol Biol Phys. 2000;48:711-722.
96. Lu TX, Mai WY, Teh BS, et al. Initial experience using intensity-modulated radiotherapy for recurrent nasopharyngeal carcinoma. Int J Radiat Oncol Biol Phys. 2004;58:682-687.
97. Adams E, Nutting CM, Convey DJ, et al. Potential role of intensity modulated radiotherapy in the treatment of tumors of the maxillary sinus. Int J Radiat Oncol Biol Phys. 2001;51:579-588.
98. Tsien C, Eisbruch A, McShan R, et al. Intensity-modulated radiation therapy (IMRT) for locally advanced paranasal sinus cancer: application of clinical decisions in the planning process. Int J Radiat Oncol Biol Phys. 2003;55:776-784.
99. Duthoy W, Boterberg T, Claus F, et al. Postoperative intensity-modulated radiotherapy in sinonasal carcinoma: clinical results in 39 patients. Cancer. 2005;104:71-82.
100. De Arruda FF, Puri DR, Zhung J, et al. Intensity-modulated radiation therapy for the treatment of oropharyngeal carcinoma: the Memorial Sloan-Kettering Cancer Center experience. Int J Radiat Oncol Biol Phys. 2006;66:966-974.
101. Ritchie JM, Smith EM, Summersgill KF, et al. Human papillomavirus infection as a prognostic factor in carcinomas of the oral cavity and oropharynx. Int J Cancer. 2003;104:336.
102. Chao KS, Ozyigit G, Tran BN, et al. Patterns of failure in patients receiving definitive and postoperative IMRT for head-and-neck cancer. Int J Radiat Oncol Biol Phys. 2003;55:312-321.
103. Braaksma MM, Wijers OB, Levendag PC, et al. Optimisation of conformal radiation therapy by intensity modulation: cancer of the larynx and salivary gland function. Radiother Oncol. 2003;66:291-302.
104. Clark CH, Bidmead AM, Nutting CM, et al. Intensity-modulated radiotherapy improves target coverage, spinal cord sparing and allows dose escalation in patients with locally advanced cancer of the larynx. Radiother Oncol. 2004;70:189-198.
105. Penagaricano JA, Ratanatharathorn V, Papanikolaou N, et al. Intensity-modulated radiation therapy reduces the dose to normal tissue in T2N0M0 squamous cell carcinoma of the glottic larynx. Med Dosim. 2004;29:254-257.
106. Lee N, O’Meara W, Chan K, et al. Concurrent chemotherapy and intensity-modulated radiotherapy for locoregionally advanced laryngeal and hypopharyngeal cancers. Int J Radiat Oncol Biol Phys. 2007;69:459-468.
107. Eisbruch A, Ten Haken R, Kim HM, et al. Dose, volume and function relationships in parotid glands following conformal and intensity modulated irradiation of head and neck cancer. Int J Radiat Oncol Biol Phys. 1999;45:577-587.
108. Eisbruch A, Schwartz M, Rasch C, et al. Dysphagia and aspiration after chemoradiotherapy for head-and-neck cancer: which anatomic structures are affected and can they be spared by IMRT? Int J Radiat Oncol Biol Phys. 2004;60:1425-1433.
109. Yeung AR, Malyapa RS, Mendenhall WM, et al. Dosimetric comparison of IMRT and proton therapy for head and neck tumors. Int J Radiat Oncol Biol Phys. 2006;66:S412.
110. Mock U, Georg D, Bogner J, et al. Treatment planning comparison of conventional, 3D conformal, and intensity-modulated photon (IMRT) and proton therapy for paranasal sinus carcinoma. Int J Radiat Oncol Biol Phys. 2004;58:147-154.
111. Cozzi L, Fogliata A, Lomax A, et al. A treatment planning comparison of 3D conformal therapy, intensity modulated photon therapy and proton therapy for treatment of advanced head and neck tumours. Radiother Oncol. 2001;61:287-297.
112. Lomax AJ, Goitein M, Adams J. Intensity modulation in radiotherapy: photons versus protons in the paranasal sinus. Radiother Oncol. 2003;66:11-18.
113. Liebsch NJ, Truong M, Lopes VV, et al. Conformal proton radiotherapy for primary sphenoid sinus malignancies. Int J Radiat Oncol Biol Phys. 2006;66:S419-419.
114. Laramore GE, Krall JM, Griffin TW, et al. Neutron versus photon irradiation for unresectable salivary gland tumors: final report of an RTOG-MRC randomized clinical trial. Radiation Therapy Oncology Group. Medical Research Council. Int J Radiat Oncol Biol Phys. 1993;27:235-240.
115. Mizoe JE, Tsujii H, Kamada T, et al. Dose escalation study of carbon ion radiotherapy for locally advanced head-and-neck cancer. Int J Radiat Oncol Biol Phys. 2004;60:358-364.
116. Barker JLJr, Garden AS, Ang KK, et al. Quantification of volumetric and geometric changes occurring during fractionated radiotherapy for head-and-neck cancer using an integrated CT/linear accelerator system. Int J Radiat Oncol Biol Phys. 2004;59:960-970.
117. Brenner DJ, Hall EJ. Conditions for the equivalence of continuous to pulsed low dose rate brachytherapy. Int J Radiat Oncol Biol Phys. 1991;20:181.
118. Visser AG, van den Aardweg GJMJ, Levendag PC. Pulsed dose rate and fractionated high dose rate brachytherapy: choice of brachytherapy schedules to replace low dose rate treatments. Int J Radiat Oncol Biol Phys. 1996;34:497.
119. Fowler JF. Loss of local control with prolongation in radiotherapy. Int J Radiat Oncol Biol Phys. 1992;23:457.
120. Robertson C, Robertson AG, Hendry JH, et al. Similar decreases in local tumor control are calculated for treatment protraction and for interruptions in the radiotherapy of carcinoma of the larynx in four centers. Int J Radiat Oncol Biol Phys. 1998;40:319.
121. Brizel DM, Wasserman T. The influence of intravenous amifostine on xerostomia and survival during radiotherapy for head and neck cancer: two year follow-up of a prospective randomized trial (abstract). Proc Am Soc Clin Oncol. 2004;23:495a.
122. Buentzel J, Micke O, Adamietz IA, et al. Intravenous amifostine during chemoradiotherapy for head-and-neck cancer: a randomized placebo-controlled phase III study. Int J Radiat Oncol Biol Phys. 2006;64:684.
123. Vacha P, Fehlauer F, Mahlmann B, et al. Randomized phase III trial of postoperative radiochemotherapy ± amifostine in head and neck cancer: is there evidence for radioprotection? Strahlenther Onkol. 2003;179:38107.
124. Sonis ST, Eilers JP, Epstein JB, et al. Validation of a new scoring system for the assessment of clinical trial research of oral mucositis induced by radiation or chemotherapy. Mucositis Study Group. Cancer. 1999;85:2103.
125. Vera-Llonch M, Oster G, Hagiwara M, et al. Oral mucositis in patients undergoing radiation treatment for head and neck carcinoma. Cancer. 2006;106:329.
126. Braam PM, Roesink JM, Moerland MA, et al. Long-term parotid gland function after radiotherapy. Int J Radiat Oncol Biol Phys. 2005;62:659.
127. Eisbruch A, Ship JA, Martel MK, et al. Parotid gland sparing in patients undergoing bilateral head and neck irradiation: techniques and early results. Int J Radiat Oncol Biol Phys. 1996;36:469.
128. Sciubba JJ, Goldenberg D. Oral complications of radiotherapy. Lancet Oncol. 2006;7:175.
129. Wahl MJ. Osteoradionecrosis prevention myths. Int J Radiat Oncol Biol Phys. 2006;64:661-669.
130. Mendenhall WM. Mandibular osteoradionecrosis. J Clin Oncol. 2004;22:4867-4868.
131. Niewald M, Barbie O, Schnabel K, et al. Risk factors and dose-effect relationship for osteoradionecrosis after hyperfractionated and conventionally fractionated radiotherapy for oral cancer. Br J Radiol. 1996;69:847.
132. Beumer J, Harrison R, Sanders B, et al. Osteoradionecrosis: predisposing factors and outcomes of therapy. Head Neck Surg. 1984;6(4):819-827.
133. Ben-David M, Diamante M, Radawski M, et al. Lack of osteoradionecrosis of the mandible after intensity-modulated radiotherapy for head and neck cancer: likely contributions of both dental care and improved dose distributions. Int J Radiat Oncol Biol Phys. 2007;68:396-402.
134. Wong JK, Wood RE, McLean M. Conservative management of osteoradionecrosis. Oral Surg Oral Med Oral Pathol Oral Radiol Endod. 1997;84:16.
135. Hao SP, Chen HC, Wei FC, et al. Systematic management of osteoradionecrosis in the head and neck. Laryngoscope. 1999;109:1324.
136. Bui QC, Lieber M, Withers HR, et al. The efficacy of hyperbaric oxygen therapy in the treatment of radiation-induced late side effects. Int J Radiat Oncol Biol Phys. 2004;60:871.
137. Annane D, Depondt J, Aubert P, et al. Hyperbaric oxygen therapy for radionecrosis of the jaw: a randomized, placebo-controlled, double-blind trial from the ORN96 Study Group. J Clin Oncol. 2004;22:4893.
138. Tell R, Lundell G, Nilsson B, et al. Long-term incidence of hypothyroidism after radiotherapy in patients with head-and-neck cancer. Int J Radiat Oncol Biol Phys. 2004;60:395.
139. Coleman JJ3rd. Treatment of the ruptured or exposed carotid artery: a rational approach. South Med J. 1985;78:262.
140. Lam HC, Abdullah VJ, Wormald PJ, et al. Internal carotid artery hemorrhage after irradiation and osteoradionecrosis of the skull base. Otolaryngol Head Neck Surg. 2001;125:522.
141. Marcus RBJr, Million RR. The incidence of myelitis after irradiation of the cervical spinal cord. Int J Radiat Oncol Biol Phys. 1990;19:3-8.