CHAPTER 36 Principles of radiotherapy and chemotherapy
Radiotherapy
Radiation physics
Electromagnetic radiation
X-rays and gamma-rays (γ-rays) are part of the electromagnetic spectrum; the former are produced artificially and the latter from naturally occurring or artificially produced radioisotopes. Megavoltage X-rays for therapeutic purposes are produced by a linear accelerator (Figure 36.1) by accelerating electrons to high kinetic energies on to a target composed of tungsten or gold. Kinetic energy given up by the electrons is converted to high-energy X-rays. γ-rays are emitted as byproducts of radioactive isotopes (e.g. iridium-192) undergoing decay to reach a stable nuclide.
Radiobiology
External beam radiotherapy
Conformal radiotherapy
The CT scan images are subsequently transferred to a computer terminal (Figure 36.2) with software allowing delineation of target volumes and organs at risk according to recommendations from the International Commission on Radiation Units and Measurements. The gross tumour volume includes the palpable and/or radiologically visible tumour; it is absent if it has been surgically resected. The clinical target volume (CTV) involves the gross tumour volume and potential areas of microscopic disease (e.g. pelvic lymph nodes and parametria in cervical cancer). The CTV is grown by a margin to allow for internal organ motion and geometric uncertainties in treatment delivery; this is called the ‘planning target volume’ (PTV). The organs at risk for pelvic radiation include the rectum, bladder, small bowel and both hip joints. The kidneys and spinal cord are the dose-limiting organs at risk when radiating the para-aortic lymph nodes. The clinician specifies the dose fractionation to be used in the radiation treatment of the patient.
The final radiotherapy plan comprises the following information: the number of beams used to optimize conformity to the PTV, beam energies, beam direction, use of wedge angles and multi-leaf collimators to improve PTV conformity, and the dose distribution and percentage dose received by the PTV. A dose–volume histogram is provided to determine the dose being received by the PTV and organs at risk. The final plan is checked and approved by the clinician prior to implementation of the radiotherapy plan (Figure 36.3). Verification checks are performed to validate the treatment plan and to detect for any unforeseen errors prior to starting radiation treatment. A standard dose prescription for carcinoma of the cervix is 45–50 Gy in 25–28 fractions using 1.8 Gy/fraction over 5–5.5 weeks.
The patient is treated in a specially designed room to prevent radiation of personnel outside the room. The patient is positioned on the treatment couch with reference to the tattoo marks (Figure 36.4). Computerized transfer of planning data from the planning computer to the linear accelerator minimizes the risk of human error in transferring data. The radiographers control the linear accelerator from outside the room using a console which is used to start and stop the radiation beam, and to set the duration of treatment and the dose to be delivered. The patient is monitored using cameras in the treatment room, and communication with the patient is possible via a microphone. These safety features are designed to optimize radiation treatment and are subject to frequent quality assurance checks.
Virtual simulation
The planning CT scan images can be reconstructed using computer software to provide a digitally reconstructed radiograph (DRR). Bony landmarks are used for radiotherapy planning with the clinician using the computer software to place the radiation beams on to the DRR. This is akin to conventional radiotherapy. The advantages of virtually simulated radiotherapy planning include: the clinician is able to visualize the pelvic soft tissues by using the CT scan images, thus ensuring the tumour is encompassed within the radiation field; radiotherapy planning is less time-consuming compared with conformal radiotherapy; the clinician can apply multi-leaf collimators at the time of planning to optimize shielding of the organs at risk; and a dose distribution can be provided by the medical physicists to ensure that the PTV is receiving the prescribed dose. The disadvantages of virtually simulated radiotherapy planning are that it is not suitable for producing complex radiotherapy plans, and a dose–volume histogram cannot be provided to determine the dose being received by the organs at risk. Virtually simulated radiotherapy (Figure 36.5) is frequently utilized for pelvic radiotherapy for the reasons outlined above.
Conventional radiotherapy
Conventional external beam radiotherapy uses bony landmarks to define the target volume for pelvic radiotherapy. A simulator film is obtained after the patient is aligned on the simulator couch using orthogonal lasers. Cross wires mounted in the light beam from the simulator define the size of the area to be treated, and anterior–posterior and lateral pelvic X-rays are obtained as permanent records. The clinician is able to identify areas where shielding can be applied to reduce dose to the organs at risk (Figure 36.6). As diagnostic X-rays have poor soft tissue resolution, the clinician must rely on information gathered from clinical examination and radiological investigations to determine the target volume. Studies have reported underdosing of the regional lymph nodes in up to 40% of patients using conventional radiotherapy. However, conventional radiotherapy planning still has a role in centres where access to CT planning software is restricted due to resource implications, in patients unable to fit into a CT scan machine due to gross obesity, and in palliative radiotherapy.
Brachytherapy
Intracavitary brachytherapy
This technique is routinely used for the radical treatment of cervical cancer where hysterectomy has not been performed, and occasionally in patients with inoperable endometrial cancer. The procedure is carried out under general anaesthesia unless contraindicated, in which case spinal anaesthesia provides a suitable alternative. The patient is examined to assess tumour size and parametrial involvement, and a urinary catheter is inserted. Following cervical dilatation, a sound is used to assess the uterine length. The width of the vaginal vault is estimated to guide the applicator sizes required. The intrauterine tube is inserted first, followed by the vaginal applicators. A rectal retractor may also be inserted to push the anterior rectal wall further away from the applicators; alternatively, a gauze pack may be used to achieve this purpose and also to secure the applicators in place. A simulator film is taken with the applicators in place once the patient is awake, which is then transferred on to a planning computer to allow dosimetry calculations to be performed. The patient is treated in an appropriately shielded room using a remote afterloading system (Figure 36.7). The patient is monitored via a camera inside the treatment room, and a microphone can be used to communicate with the patient. Once treatment is completed, the applicator and gauze packing are removed and the patient is discharged home.
A number of applicator systems are available for intracavitary brachytherapy, all of which are based around the principle of delivering a high radiation dose to the cervix, parametrium and upper vagina. Two main types of applicator are available (Figure 36.8a,b,c).
Vaginal vault brachytherapy
Following hysterectomy, women with cervical or endometrial cancer with pathological features suggestive of a high risk of relapse are often recommended to have adjuvant radiotherapy, including vaginal vault brachytherapy where appropriate. Vaginal vault brachytherapy is increasingly being used as the sole adjuvant treatment for endometrial cancer in women with intermediate–high risk of pelvic relapse. A number of vaginal applicators are available for use, including vaginal cylinders (Figure 36.8D) of varying sizes, vaginal ovoids or individualized vaginal moulds. Applicator insertion may be carried out on the ward or in the brachytherapy suite, although some brachytherapists prefer the procedure to be carried out in theatre under general anaesthesia.
Interstitial brachytherapy
Interstitial brachytherapy is used for the treatment of tumours of the vagina and vulva, either as primary treatment for small tumours or to boost the primary tumour following external beam radiotherapy. It can also be used for palliating symptoms for locally recurrent or metastatic cancers of the vagina and vulva. Either LDR iridium wire interstitial implants or HDR afterloading implant techniques can be used for perineal implants using some form of perineal template (Figure 36.9). Perineal implants are performed under general or spinal anaesthesia. Computer-based planning allows for more accurate visualization of the tumour in relation to the implant (Figure 36.10).
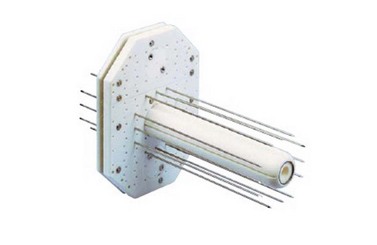
Figure 36.9 A Martinez universal perineal interstitial template for interstitial treatment of carcinoma of the vagina.
Image courtesy of Nucletron.
Image-guided adaptive brachytherapy
Simulator-based dosimetry for intracavitary brachytherapy relies on doses to points (e.g. Point A) rather than to volumes. For advanced cervical tumours, prescribing to Point A may lead to tumour underdosage as a proportion of the tumour may lie outside the high-dose envelope. Image-guided adaptive brachytherapy uses CT and/or MRI imaging to allow visualization of the tumour in relation to the applicator and the organs at risk at the time of brachytherapy to enable dose adaptation to the target whilst improving the sparing of normal tissues (Figure 36.11). The possibility of fusing FDG-PET images with planning CT software provides another potential avenue for the use of adaptive brachytherapy to improve tumour control.
Radiation morbidity
Treatment-related morbidity is the dose-limiting factor when utilizing radiotherapy for the curative treatment of any cancer, and has potentially important physical and psychosocial consequences for the patient. The risk of unwanted radiation effects is determined by the interaction of tumour- and patient-related factors. Unwanted radiation effects are divided into early effects, arbitrarily defined as those occurring within 90 days of starting treatment, and late effects, defined as those occurring more than 90 days after starting treatment. In pelvic radiotherapy, the small bowel, rectum and bladder are the main organs at risk. The kidneys and spinal cord are the vital organs at risk when para-aortic lymph node irradiation is required. Table 36.1 summarizes the early and potential late effects associated with pelvic radiation.
Organ | Early effect | Late effect |
---|---|---|
Skin | Erythema, desquamation | Telangiectasia, fibrosis |
Small bowel, sigmoid and rectum | Radiation colitis or proctitis | Radiation proctitis, diarrhoea, bowel stenosis, fistulae |
Bladder | Radiation cystitis | Radiation cystitis, fistulae |
Hip joints and pelvic bones | Osteoradionecrosis, insufficiency fractures | |
Ovaries | Infertility, menopause | |
Vagina | Mucositis | Vaginal stenosis, fistulae |
Lymph nodes | Lymphoedema | |
Spinal cord | Radiation myelopathy | |
Kidneys | Renal failure, hydronephrosis | |
General | Secondary cancers |
Chemotherapy
Pharmacology
Principles of combination therapy
Classification
The most common classification of cytotoxic drugs is based on their mechanisms of action. Drugs are divided into alkylating agents, antimetabolites, vinca alkaloids, antibiotics, topoisomerase inhibitors, tubulin-binding agents and others. Table 36.2 summarizes the mechanism of action and toxicity of some of the chemotherapy agents mentioned below.
Table 36.2 Chemotherapeutic agents used in gynaecological cancers, mechanisms of action and toxicities
Chemotherapy-related toxicity
Tissues affected by toxic effects include the bone marrow, injection site, skin, hair, mucosa, gastrointestinal tract, reproductive system, heart, lungs, liver, renal system and nervous system. Knowledge of these toxic effects is essential for the safe management of chemotherapy. The most common toxicities for each of the commonly used drugs are shown in Table 36.2. Patients with poor performance, multiple comorbidities and heavily pretreated patients are less likely to tolerate chemotherapy.
Conclusion
KEY POINTS
Acknowledgements
The authors would like to convey their gratitude to Professor Peter Hoskin, Mount Vernon Hospital, Northwood, for providing Figures 36.3, 36.5(A,B), 36.10 and 36.11(A,B); and to Ruth Wyatt, Queen Elizabeth Hospital, Birmingham, and Nucletron, for providing Figures 36.8(B,C) and 36.9.
Green J, Kirwan J, Tierney J et al 2005 Concomitant chemotherapy and radiotherapy for cancer of the uterine cervix. Cochrane Database of Systematic Reviews 3: CD002225.
King M, McConkey C, Latief TN, Hartley A, Fernando IN. Improved survival after concurrent weekly cisplatin and radiotherapy for cervical carcinoma with assessment of acute and late side effects. Clinical Oncology (Royal College of Radiologists). 2006;18:38-45.
Tanguay JS, Ansari J, Buckley L, Fernando I. Epithelial ovarian cancer: role of pegylated liposomal doxorubicin in prolonging the platinum-free interval and cancer antigen 125 trends during treatment. International Journal of Gynecological Cancer. 2009;19:361-366.
Dale RG, Jones B. The clinical radiobiology of brachytherapy. British Journal of Radiobiology. 1998;71:465-483.
Fischer DS, Knobf MT, Durivage HJ, Beaulieu NJ. The Cancer Chemotherapy Handbook, 6th edn. Philadelphia: Mosby Inc.; 2003.
. Radiotherapy in Practice: External Beam Radiotherapy. Hoskin P. Oxford University Press, Oxford, 2006.
Hoskin P, Coyle C, editors. Radiotherapy in Practice: Brachytherapy. Oxford: Oxford University Press, 2005.
Poole CJ, Palmer D. Chemotherapy for gynaecological malignancies. In: Shafi MI, Leusley DM, Jordan JA, editors. Handbook of Gynaecological Oncology. London: Harcourt Publishers Limited, 2001.