34 Olfactory and limbic systems
Olfactory System
The olfactory system is remarkable in four respects:
Olfactory epithelium
The olfactory epithelium occupies the upper one-fifth of the lateral and septal walls of the nasal cavity. The epithelium contains three cell types (Figure 34.1):
Olfactory bulb (Figure 34.1)
Central connections
Mitral cell axons run centrally in the olfactory tract (Figure 34.2). The tract divides in front of the anterior perforated substance into medial and lateral olfactory striae.
Points of clinical interest are mentioned in Clinical Panel 34.1.
Limbic System
The limbic system comprises the limbic cortex (so-called limbic lobe) and related subcortical nuclei. The term ‘limbic’ (Broca, 1878) originally referred to a limbus or rim of cortex immediately adjacent to the corpus callosum and diencephalon. The limbic cortex is now taken to include the three-layered allocortex of the hippocampal formation and septal area, together with transitional mesocortex in the parahippocampal gyrus, cingulate gyrus, and insula. The principal subcortical component of the limbic system is the amygdala, which merges with the cortex on the medial side of the temporal pole. Closely related subcortical areas are the hypothalamus, reticular formation, and the nucleus accumbens. Cortical areas closely related to the limbic system are the orbitofrontal cortex and the temporal pole (Figure 34.3).
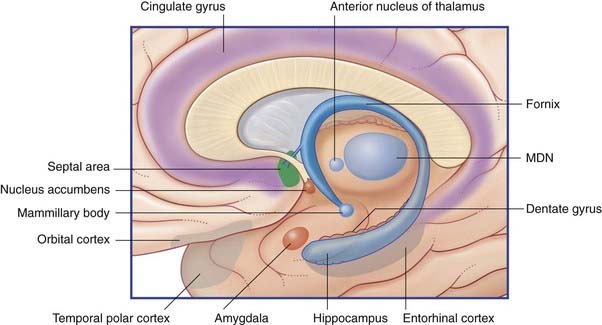
Figure 34.3 Medial view of cortical and subcortical limbic areas. MDN, mediodorsal nucleus of thalamus.
Figure 34.4 is a graphic reconstruction of mainly subcortical limbic areas.
Hippocampal complex
The hippocampal complex (or hippocampal formation) comprises the subiculum, the hippocampus proper, and the dentate gyrus (Figure 34.5). All three are composed of temporal lobe allocortex which has tucked itself into an S-shaped scroll along the floor of the lateral ventricle. The band-like origin of the fornix from the subiculum and hippocampus is the fimbria. The hippocampus is also known as Ammon’s horn (after an Egyptian deity with a ram’s head). For research purposes, it is divided into four cornu ammonis (CA) zones (Fig. 34.6A).
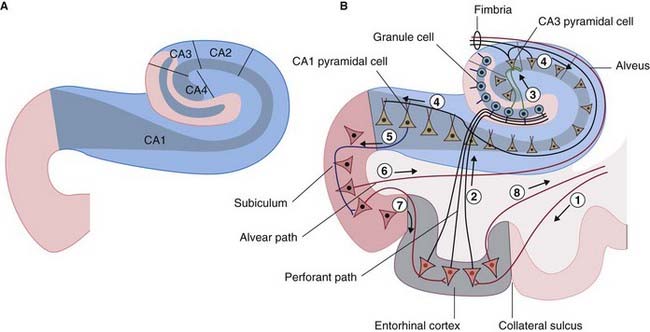
Figure 34.6 (A) The four sectors of Ammon’s horn. (B) Input–output connections of the hippocampal formation.
Connections
Afferents
The largest afferent connection of the hippocampal complex is the perforant path, which projects from the entorhinal cortex onto the dendrites of dentate granule cells (Figure 34.6B). The subiculum gives rise to a second, alvear path which contributes to a sheet of fibers on the ventricular surface of the hippocampus, the alveus.
Auditory information enters the hippocampus from the association cortex of the superior and middle temporal gyri. The supramarginal gyrus (area 40) transmits coded information about personal space (the body schema described in Ch. 32) and extrapersonal (visual) space. From the occipito-temporal region on the inferior surface, information concerning object shape and color, and facial recognition, is projected to cortex called perirhinal, or transrhinal, immediately lateral to the entorhinal cortex. From here, it enters the hippocampus. A return projection from entorhinal to perirhinal cortex is linked to the temporal polar and prefrontal cortex.
Clinical Panel 34.2 Schizophrenia
Medications used to treat psychotic disorders such as schizophrenia are called antipsychotics, neuroleptics or major tranquillizers. All such antipsychotic medications block dopamine D2 receptors to some extent (e.g. haloperidol or olanzapine). In the normal brain, the D2 receptors are on spiny (excitatory) stellate cells in the mesocortical projection territory of the ventral tegmental nucleus. D2 receptors are inhibitory, for one or more of three possible reasons noted in Chapter 8. Interestingly, symptoms closely resembling the positive psychotic ones of schizophrenia may be induced by consuming excessive amounts of dopamine-stimulating drugs such as cocaine or amphetamine (’speed’). Amphetamine is known to increase the amount of dopamine in the forebrain extracellular space (Clinical Panel 34.5). In schizophrenia, dopaminergic overactivity seems not to be a matter of overproduction but of greater effectiveness through an increased number of postsynaptic dopamine receptors on the spiny stellate neurons. (The assistance of Professor Colm McDonald, Department of Psychiatry, NUI, Galway, Ireland is gratefully appreciated.)
Harrison PJ, Weinberger DR. Schizophrenia genes, gene expression, and neuropathology: on the matter of their convergence. Mol Psychiatry. 2005;10:40-68.
Leonard BE. Drug treatment of schizophrenia and the psychoses. In: Fundamentals of psychopharmacology. Chichester: Wiley; 2003:255-294.
Picchioni MM, Murray RM. Schizophrenia. BMJ. 2007;335:91-95.
Efferents
The largest efferent connection is a massive projection via the entorhinal cortex to the association areas of the neocortex. A second, forward projection is the fornix (Figure 34.5A). The fornix is a direct continuation of the fimbria, which receives axons from the subiculum and hippocampus proper. The crus of the fornix arches up beneath the corpus callosum, where it joins its fellow to form the trunk and links with its opposite number through a small hippocampal commissure. Anteriorly, the trunk divides into two pillars. Each pillar splits around the anterior commissure, sending precommissural fibers to the septal area and postcommissural fibers to the anterior hypothalamus, mammillary body, and medial forebrain bundle. The mammillary body projects into the anterior nucleus of thalamus, which projects in turn to the cingulate cortex, completing the Papez circuit from cingulate cortex to hippocampus, with return to cingulate cortex via fornix, mammillary body, and anterior thalamic nucleus (Figure 34.7).
The term medial temporal lobe is clinically inclusive of the hippocampal complex, parahippocampal gyrus, and amygdala. The term is most often used in relation to seizures (Clinical Panel 34.3).
Clinical Panel 34.3 Temporal lobe epilepsy
Complex focal (partial) seizures are synonymous with temporal lobe epilepsy. The initial event, or aura, may be a simple partial seizure whose electrical activity escapes into the temporal lobe. Many originate in a focus of runaway neural activity within the temporal lobe and spread over the general cortex within seconds to trigger a secondarily generalized tonic–clonic seizure (Figure CP 34.3.1) as mentioned in Chapter 30. Types of temporal lobe auras include well-formed visual or auditory hallucinations (scenes, sound sequences), a sense of familiarity with the surrounding scene (’déjà vu’), a sense of strangeness (‘jamais vu’) or a sense of fear. Attacks originating in the uncus are ushered in by unpleasant olfactory or gustatory auras. Bizzare psychic auras can occur, where the patient has an ‘out of body experience’ in the form of a sensation of floating in the air and looking down at themselves and any others present.
Emerson RG, Pedley TA. EEG and evoked potentials. In: Bradley WG, Daroff RB, Finichel GM, Jankovic J, editors. Neurology in clinical practice: principles of diagnosis and management. Philadelphia: Elsevier; 2004:465-490.
Bernasconi N, Bernasconi A, Caramanos Z, et al. Mesial temporal damage in temporal lobe epilepsy: a volumetric MRI study of the hippocampus, amygdala and parahippocampal region. Brain. 2003;126:462-469.
Jay V, Becker LE. Surgical pathology of epilepsy. Pediatr Pathol. 2003;14:731-750.
Kempermann G. The neurogenic reserve rehypothesis: what is adult hippocampal neurogenesis good for? Trends Neurosci. 2008;89:1169-1176.
Niedergerger E, Kuhlein H, Geissler G. Update on the neuropathology of neuropathic pain. Expert Rev proteomics. 2008;5:719-818.
Shin C. Mechanism of epilepsy. Ann Rev Med. 1994;45:379-389.
Willis WD, Westland KR. Pain system. In: Paxinos G, Mai JK, editors. The human nervous system. ed 2. Amsterdam: Elsevier; 2004:1125-1170.
Glossary
Clinical and experimental observations
Long-term potentiation (LTP) is uniquely powerful in the dentate gyrus and hippocampus. It is regarded as vital for preservation (consolidation) of memory traces. Under experimental conditions, LTP is most easily demonstrated in the perforant path–dentate granule cell connections and in the Schaffer collateral–CA1 connections. A strong, brief (milliseconds) stimulus to the perforant path or Schaffer collaterals induces the target cells to show long-lasting (hours) sensitivity to a fresh stimulus. LTP is associated with a cascade of biochemical events in the target neurons, following activation of appropriate glutamate receptors, as described in Chapter 8 in the context of pain sensitization. Repetitive stimuli may cause cAMP to increase its normal rate of activation of protein kinases involved in phosphorylation of proteins that regulate gene transcription. The outcome is increased production of proteins (including enzymes) required for transmitter synthesis, and of other proteins for construction of additional channels and synaptic cytoskeletons.
LTP is described as an associative phenomenon, because the required expulsion of the magnesium plug from the NMDA receptor (Figure 34.8) is facilitated when the powerful depolarizing stimulus is coupled with a weaker stimulus to the depolarized neuron from another source. Norepinephrine and dopamine are suitable associative candidates, one or both being released during elevation of the attentional or motivational state at the appropriate time.
Cholinergic activity in the hippocampus is also significant for learning. In human volunteers, central ACh blockade (by administration of scopolamine) severely impairs memory for lists of names or numbers, whereas a cholinesterase inhibitor (physostigmine) gives above-normal results. Clinically, hippocampal cholinergic activity is severely reduced in Alzheimer’s disease (AD), which is particularly associated with amnesia (see Clinical Panel 34.4).
Left vs right hippocampal functions
In keeping with known hemispheric asymmetries, the left anterior hippocampus and dorsolateral prefrontal cortex (DLPFC) are engaged in encoding novel material involving language function. Also consistent is the finding that the right hippocampus and right inferior parietal lobe are engaged in spatial tasks such as driving a car (Figure 34.9). Blood flow in the DLPFC increases more on the left side during driving, presumably because of the ‘inner speech’ that occurs when exploring novel territory.
Insula
The anterior insula is a cortical center for pain (Box 34.1). The central region is continuous with the frontoparietal and temporal opercular cortex, and it seems to have a language rather than a limbic function. During language tasks, PET scans show activity there as well as in the opercular speech receptive and motor areas – but not in people with congenital dyslexia, where it remains silent (Ch. 32). The posterior insula is interconnected with the entorhinal cortex and the amygdala, and is therefore presumed to participate in emotional responses – perhaps in the context of pain evaluation.
Box 34.1 Pain and the brain
Table Box 34.1.1 is a glossary of conventional terms used in relation to pain.
Peripheral pain pathways
As already noted in Chapter 9, pain is served by finely myelinated (Aδ) and unmylinated (C) fibers belonging to unipolar spinal ganglion cells. These fibers are loosely known as ‘pain fibers’, although others of similar diameters are purely mechanoreceptors and others again elicit pain only when discharging at high frequency, notably mechanical nociceptors and thermoreceptors. The latter are referred to as polymodal nociceptors in the general context of pain.
From the viscera, the distal processes share perineural sheaths with postganglionic fibers of the sympathetic system. The proximal processes mingle with the somatic fibers within Lissauer’s tract and terminate in the same region. As noted in Chapter 13, overlap of somatic and visceral afferent terminals on the dendrites of central pain-projecting neurons is thought to account for referred pain in visceral disorders such as myocardial infarction and acute appendicitis.
Sensitization of nociceptors
Injured tissue liberates molecules including bradykinin, prostaglandin, and leukotrienes, which lower the activation threshold of nociceptors. Injured C fibers also initiate axon reflexes (Ch. 11), whereby substance P ± CGRP (calcitonin gene-related peptide) is liberated into the adjacent tissue, causing histamine release from mast cells. Histamine receptors may develop on the nerve terminals and (as already noted in Ch. 8) produce arachidonic acid by hydrolysis of membrane phospholipids. The enzyme cyclooxidase converts arachidonic acid into a prostaglandin. (The main action of aspirin and other non-steroidal anti-inflammatory analgesics is to inactivate that enzyme, thereby reducing synthesis of prostaglandins.)
As already noted in Chapter 13, irritable bowel syndrome is characterized by sensitization of nociceptive interoceptors in the bowel wall. That event also underlies the painful urinary bladder condition known as interstitial cystitis.
Sensitization of C-fiber neurons may include gene transcription (Ch. 8), whereby abnormal sodium channels are inserted into the cell membrane of the parent neurons in the posterior root ganglion. Spontaneous trains of impulses generated here are thought to account for occasional failure of quite high-level nerve blocks to abolish the pain.
Central pain pathways
Central pain-projecting neurons are of two kinds, as described in Chapter 15: nociceptive-specific, with small peripheral sensory fields (about 1 cm2), and wide dynamic range, with fields of 2 cm2 or more; these are mechanical nociceptors encoding tactile stimuli by low-frequency impulses and noxious stimuli by high-frequency impulses.
The current consensus is that there exists a lateral, sensory-discriminative pathway and a medial, affective pathway in relation to pain (Figures Box 34.1.1 and 34.1.2).
Lateral pain pathway
Onward projections to the posterior parietal cortex and SII are indicated in Figures Box 34.1.1 and 34.1.2.
Not surprisingly, the spinothalamic early warning system stimulates orientation of head and eyes toward the source of pain. As mentioned in Chapter 15, the spinotectal tract ascends alongside the spinothalamic and terminates in the superior colliculus. Its imprint is somatotopic and it elicits a spinovisual reflex to orient the eyes/head/trunk toward the area stimulated. In addition to activation of this phylogenetically ancient (reptilian) reflex, the ‘Where?’ visual channel (Ch. 29) is engaged by association fibers passing to the posterior parietal cortex from SI.
Central pain states
As mentioned in Chapter 27, the central pain state known as thalamic syndrome may develop following a vascular lesion in the white matter close to the ventroposterior nucleus of the thalamus. Explanation of the bouts of severe contralateral pain sensation may lie in elimination of the normal inhibitory feedback to the posterior thalamus from the surrounding thalamic reticular nucleus.
Benarroch EE. Descending monoaminergic pain modulation. Neurology. 2008;71:217-221.
Brooks J, Tracey I. From nociception to pain perception: imaging the spinal and supraspinal pathways. J Anat. 2005;207:19-33.
Froth M, Mauguiere M. Dual representation of pain in the operculo-insular cortex in humans. Brain. 2003;126:438-450.
Gatchel RJ. Clinical essentials of pain management. Washington: American Association; 2005.
Vanegas H, Schaible H-G. Descending control of persistent pain: inhibitory or facilitatory? Brain Res Rev. 2004;46:295-309.
Villanueva L, Nathan PW. Multiple pain pathways. In: Progress in pain research and management. Seattle: IASP Press; 2000:371-386.
Willis WD, Westlund KR. Pain system. In: Paxinos G, Mai JK, editors. The human nervous system,. ed 2. Amsterdam: Elsevier; 2004:1125-1170.
Term | Meaning |
---|---|
Allodynia | Pain produced by normally innocuous stimuli. Examples: stroking sunburned skin; moving an inflamed joint. |
Central pain-projecting neurons (CPPNs) | An inclusive conventional term denoting all posterior horn neurons projecting pain-encoded information to contralateral brainstem and thalamic nuclei. Pathways included are: spinothalamic, the lateral pain pathway to posterior nucleus of thalamus; spinoreticulothalamic, medial pain pathway to the medial and intralaminar nuclei of thalamus via brainstem reticular formation; spinoamygdaloid, to the amygdala via the reticular formation; and spinotectal, to the superior colliculus. |
Central pain state | A state of chronic pain, resistant to therapy, sustained by hypersensivity of peripheral and/or central neural pathways. |
Wind-up phenomenon | Sustained state of excitation of CPPNs induced by glutamate activation of NMDA receptors. |
Fast pain | Stabbing pain perceived following activation of Aδ nociceptors. |
Hyperalgesia | Hypersensitivity to stimulation of injured tissue, and of surrounding uninjured tissue. Causes include mechanical or thermal damage, bacterial/viral inflammation, small-fiber peripheral axonal neuropathy, radiculopathy (posterior nerve root injury). |
Neurogenic inflammation | Inflammation caused by liberation of substance P (in particular) following antidromic depolarization of fine peripheral nerve fibers. |
Neuropathic pain | Chronic stabbing or burning pain resulting from injury to peripheral nerves. Examples: postherpetic neuralgia; amputation neuroma. |
Nociceptors | Peripheral receptors whose activation generates a sense of pain. These receptors occupy the plasma membrane of fine nerve endings and contain transduction channels that convert the requisite physical or chemical stimulus into trains of impulses decoded by the brain as a sense of pain. |
Polymodal nociceptors | Peripheral nociceptors (notably in skin) responsive to noxious thermal, mechanical, or chemical stimulation. |
Sensitization | Lowering the threshold of peripheral nociceptors by histamine (in particular) following peripheral release of peptides via the axon reflex. |
Slow pain | Aching pain perceived following activation of C-fiber nociceptors. |
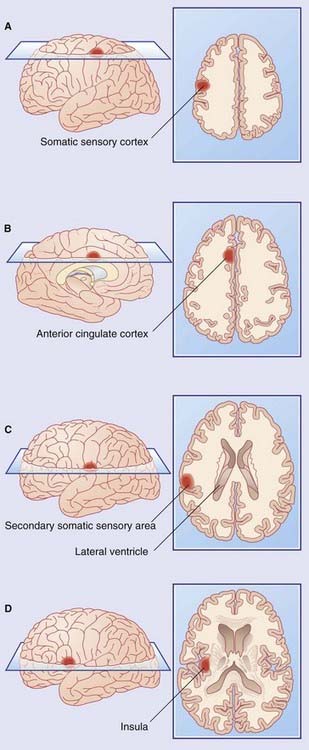
Figure Box 34.1.1 Areas showing increased metabolic activity following application of noxious heat to the right forearm.
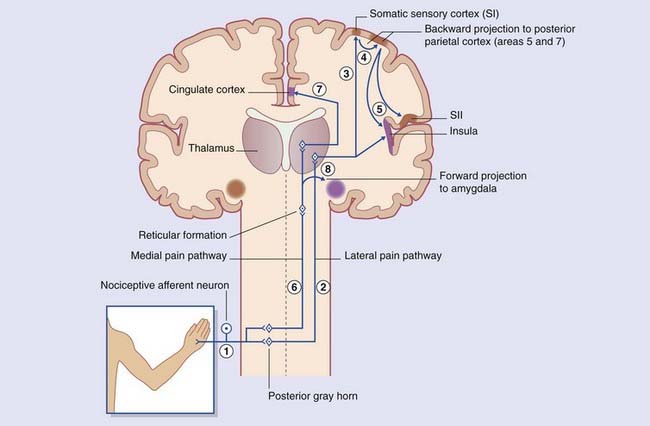
Figure Box 34.1.2 Pain pathways. Note: Violet signifies having emotional signifiance.
Cingulate cortex and posterior parahippocampal gyrus
Six functional areas can be discerned in the anterior cingulate cortex (Figure 34.10).
The posterior cingulate gyrus (area 23 of Brodmann) merges with the posterior parahippocampal gyrus (area 36). This cortical complex is richly interconnected with visual, auditory, and tactile/spatial association areas. The complex evidently contains memory stores related to these functions because PET studies reveal increased activity there when scenes or experiences are conjured up in the mind. The complex is also engaged during reading (Ch. 32).
Amygdala
The amygdala (Gr. ‘almond’; also called the amygdaloid body or amygdaloid complex) is a large group of nuclei above and in front of the temporal horn of the lateral ventricle and anterior to the tail of the caudate nucleus. The amygdala is primarily associated with the emotion of fear, as illustrated by the effect of looking at an angry or fearful face (Figure 34.11). Current clinical and basic science ambition is to gain diagnostic and therapeutic insights into the role of the amygdala with regard to various phobias and anxiety states prevalent in both the young and the adult population. The connections of the amygdala (inasmuch as these are understood) are consistent with the present perception of a ‘bottleneck’ position in the perception and expression of fear.
Afferent pathways
Within the amygdala, nuclear groups receiving afferents are predominantly laterally placed and are usually referred to collectively as the lateral nucleus. In Table 34.1 and related Figures, the afferents are segregated into subcortical and cortical.
Nature | Subcortical source | Cortical source |
---|---|---|
Tactile | Ventral posterior nucleus of thalamus | Parietal lobe |
Auditory | Medial geniculate body | Superior temporal gyrus |
Visual | Lateral geniculate body* | Occipital cortex |
Olfactory | — | Piriform lobe |
Mnemonic | — | Hippocampus/entorhinal cortex |
Cardiac | Hypothalamus | Insula |
Nociceptive | Midbrain reticular formation | — |
Cognitive | — | Orbital cortex |
Attention-related | Cerulean nucleus | Basal nucleus of Meynert |
* Afferents from LGB to amygdala have yet to be clearly identified.
Subcortical access, depicted in Figure 34.12, is thought to be especially important in infancy and childhood, at a time when the amygdala is developing faster than the hippocampus and is capable of acquiring fearful memory traces without hippocampal participation. Such memories cannot be consciously recalled at any later time despite generating physical responses of an ‘escape’ nature. The general-sense and special-sense pathways listed and depicted are sufficiently comprehensive to account for the acquisition of almost any specific ‘unexplained’ phobia (e.g. enclosed spaces, smoke, heights, dogs, faces).
As indicated in Figure 34.13, all sensory association areas of the cortex have direct access to the lateral nucleus of the amygdala. These areas are also linked to the prefrontal cortex through long association fiber bundles, rendering all conscious sensations subject to cognitive evaluation.
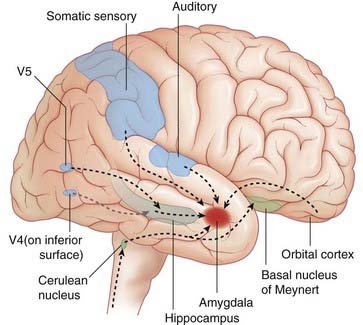
Figure 34.13 Cortical afferents to lateral nucleus of amygdala. V4, object/face recognition area; V5, motion detection area.
The orbital prefrontal cortex of the right side, with its bias toward ‘withdrawal’ rather than ‘approach’ (Ch. 32), is commonly active (in PET scans) along with the right amygdala in fearful situations, e.g. when a specific phobia is presented to a susceptible subject. On the one hand, this offers the ‘downside’ potential to ‘feed on one’s fear’. On the other hand, expert social/psychological conditioning may eventually suffice to reduce the ‘negative drive’ of the orbital cortex. When conditioning is combined with use of anxiolytic drugs, specific phobias may be abolished completely.
The insula is omitted from Figure 34.13 but, as noted earlier, its posterior part also has direct access to the amygdala, probably related to the emotional evaluation of pain.
Efferent pathways (Table 34.2)
Easily identified in the postmortem brain is the stria terminalis (Figure 34.14), which, upon emerging from the central nucleus of the amygdala, follows the curve of the caudate nucleus and accompanies the thalamostriate vein along the sulcus terminalis between thalamus and caudate nucleus. The stria sends fibers to the septal area and hypothalamus before entering the medial forebrain bundle and (downstream) the central tegmental tract. Some fibers of the stria terminate in a bed nucleus, above the anterior commissure. The bed nucleus is regarded by some workers as part of the ‘extended amygdala’; it may be more active than the amygdala proper, on PET scans, in anxiety states.
Target nucleus/pathway | Function/effect |
---|---|
Periaqueductal gray matter (to medulla/raphespinal tract) | Antinociception |
Periaqueductal gray matter (to medullary reticulospinal tract) | Freezing |
Cerulean nucleus | Arousal |
Norepinephrine medullary neurons (projection to lateral gray horn) | Tachycardia/hypertension |
Hypothalamus/dorsal nucleus of vagus (to heart) | Bradycardia/fainting |
Hypothalamus (liberation of corticotropin-releasing hormone) | Stress hormone secretion |
Parabrachial nucleus (to medullary respiratory nuclei) | Hyperventilation |
A second efferent projection, the ventral amygdalofugal pathway, passes medially to synapse within the nucleus accumbens (Figure 34.15). This connection is considered in the context of schizophrenia (Clinical Panel 34.4).
Clinical Panel 34.4 Alzheimer’s disease (AD)
MRI brain scans usually reveal severe atrophy of the cerebral cortex, with widening of the sulci and enlargement of the ventricular system. As seen in Figure CP 34.4.1, the medial temporal lobe (hippocampal complex and entorhinal cortex) are most severely affected. The primary sensory and motor areas, and the upper regions of the prefrontal cortex, are relatively well preserved.
Postmortem histological studies of the cerebral cortex reveal:
Duara R, Loewenstein DA, Potter E, et al. Medial lobe temporal atrophy on MRI scans and the diagnosis of Alzheimer disease. Neurology. 2008;71:1986-1992.
Gendron TF, Petrucelli L. The role of tau in neurodegeration. Mol Neurodegener. 2009;4:13-51.
Whitwell JL, Jack CR Jr. Neuroimaging in dementia. Neurol Clin. 2007;25:843-857.
Notes on the efferent target connections
Periaqueductal gray matter (PAG). A source of supraspinal antinociception was described in Chapter 24, namely the opioid-containing axons from the hypothalamus which disinhibit the excitatory projection from the PAG to the serotonergic cells of origin of the raphespinal tract. The excitatory cells of the dorsal PAG are directly stimulated by axon terminals entering from the amygdala via the medial forebrain bundle.
Medullary adrenergic neurons. As noted in Chapter 24, these neurons are a component of the baroreflex pathway sustaining the blood pressure against gravitational force. Sudden stimulation by the direct projection from the amygdala may send the heart dullthudding and cause a major elevation of systemic blood pressure.
Limbic striatal loop. This circuit is depicted in Chapter 33, passing from the prefrontal cortex through the nucleus accumbens and medial dorsal nucleus of thalamus, with return to the prefrontal cortex. However, the central nucleus of the amygdala participates in this circuit through an excitatory projection to the nucleus accumbens. In the right hemisphere, this projection is likely to facilitate a withdrawal response; in the left, it may facilitate an approach response.
Nucleus accumbens
The full name is nucleus accumbens septi pellucidi, ‘the nucleus leaning against the septum pellucidum’. More accurately, the nucleus abuts septal nuclei located in the base of the septum. Figures 34.15 and 34.18C show this relationship. The accumbens is one of many deep-seated brain areas where electrodes have been inserted on a therapeutic trial basis, notably in the hope of providing pain relief. Stimulation of the accumbens induces an intense sense of well-being (hedonia), comparable to that experienced by intake of drugs of addiction such as heroin (see Clinical Panel 34.5). This ‘high’ feeling is attributed to flooding of the nucleus, and of the medial prefrontal cortex, by synaptic and volume release of dopamine from the neurons projecting from the ventral tegmental area. Normally, dopamine is released in small amounts and quickly retrieved from the extracellular space by a specific dopamine reuptake transporter.
Clinical Panel 34.5 Drugs of dependency
Experimental evidence from the injection of drugs of abuse has yielded the following results (Figures CP 34.5.1 and 34.5.2):
Septal area
The septal area comprises the septal nuclei, merging with the cortex directly in front of the anterior commissure, together with a small extension into the septum pellucidum (Figure 34.16).
Afferents to the septal nuclei are received from:
The two chief efferent projections are the:
Basal forebrain
The basal forebrain extends from the bifurcation of the olfactory tract as far back as the infundibulum, and from the midline to the amygdala (Figure 34.18). In the floor of the basal forebrain is the anterior perforated substance, pierced by anteromedial central branches arising from the arterial circle of Willis (Ch. 5). Here the cerebral cortex is replaced by scattered nuclear groups, of which the largest is the magnocellular basal nucleus of Meynert.
The cholinergic neurons of the basal forebrain have their somas mainly in the septal nuclei and basal nucleus of Meynert (Figure 34.19). The basal nucleus projects to all parts of the cerebral neocortex, which also contains scattered intrinsic cholinergic neurons.
Neurogenesis in the adult brain
Adolphs R. Fear, faces, and the human amygdale. Curr Opin Neurobiol. 2008;18:166-172.
Bayley PJ, Gold JJ, Hopkins RO, Squire L. The neuroanatomy of remote memory. Neuron. 2005;46:799-810.
Becerra L, Borsook D. Signal valence in the nucleus accumbens to pain onset and offset. Eur J Pain. 2008;12:866-869.
Benaroch EE. Descending monoaminergic pain modulation. Neurology. 2008;72:217-221.
Bruen PD, McGeown WJ, Shanks MF, Veneri A. Neuroanatomical correlates of neuropsychiatric symptoms in Alzheimer’s disease. Brain. 2008;131:2455-2463.
Derntl B, Habel U, Windischberger C, et al. General and specific responsiveness of the amygdala during explicit motion recognition in females and males. BMC Eurosci. 2009;10:91-115.
Diederich NJ, Goetz CG. The placebo treatments in neurosciences. Neurology. 2008;71:677-684.
Doetch F, Hen R. Young and excitable: the function of new neurons in the adult mammalian brain. Curr Opin Neurobiol. 2005;15:121-128.
Dolan RJ, Paulesu E, Fletcher P. Human memory systems. In: Frackowiak RSJ, Friston KJ, Frith CD, Dolan RJ, editors. Human brain function. London: Academic Press; 1997:367-404.
Duvernoy HM, The human hippocampas with drawings by J.L. Vannson, ed 2, Springer, Berlin, 1998.
Friedman JH, Dubinsky R. The placebo effect. Neurology. 2008;71:25-26.
Hansson E. Could chronic pain and spread of pain sensation be induced and maintained by glial activation? Acta Physiol. 2006;187:321-327.
Henriksen G, Willch I. Imaging of opioid receptors in the central nervous system. Brain. 2008;131:1171-1196.
Johansen-Berg H, Gutman DA, Behrens TJ, et al. Anatomical connectivity of the subgenual cingulate region targeted with deep brain stimulation for treatment-resistant depression. Cereb Cortex. 2008;18:1374-1383.
Kemperrmann G, Wiskott L, Gage FH. Functional significance of adult neurogenesis. Curr Opin Neurobiol. 2004;14:186-191.
Kim E, Shirvalkar P, Herrera DG. Regulation of neurogenesis in the aging vertebrate brain: role of oxidative stress and neuroactive trophic factors. Clin Neurosci Res. 2003;2:285-343.
Kretschmann H-J, Weinrich W. Neurofunctional systems: 3D reconstructions with correlated neuroimaging: text and CD-ROM. New York: Thieme; 1998.
Leonard BE. Fundamentals of psychopharmacology, ed 3. Chichester: Wiley; 2003.
Liddell BL, Brown KJ, Kemp AH, et al. A direct brainstem-amgdala-cortical ‘alarm’ system for subliminal signals of fear. NeuroImage. 2005;24:235-243.
Liss B, Roeper J. Individual dopamine midbrain neurons: functional diversity and flexibility in health and disease. Brain Res Rev. 2008;58:314-321.
Moscovitch M, Rosenbaum RS, Gilboa A, et al. Functional neuroanatomy of remote episodic, semantic and spatial memory. J Anat. 2005;207:35-66.
Passingham D, Sakai K. The prefrontal cortex and working memory: physiology and brain imaging. Curr Opin Neurobiol. 2004;14:163-168.
Perry RJ, Hodges JR. Attention and executive deficits in Alzheimer’s disease. Brain. 1999;122:383-404.
Phelps EA. Human emotion and memory: interactions of the amygdala and hippocampal complex. Curr Opin Neurobiol. 2004;14:198-202.
Phelps EA, The human amygdala and the control of fear, In Tait MJ, Sadoun S, Bell A, Bass A, editors: Water movements in the brain: role of aquaporins, Trends Neurosci, 31, 37-43, 2008.
Richardson MP, Strange B, Dolan RJ. Encoding of emotional memories depends on the amygdala and hippocampus and their interactions. Nat Neurosci. 2000;7:278-285.
Schott G. Pain. In: Clarke C, Howard R, Rossor M, Shorvon S, editors. Neurology: A Queen Square Textbook. London: Wiley-Blackwell; 2009:847-870.
Selden NR, Gitleman DR, Salammon-Murayama N, Parrish T, Mesulam M-M. Trajectories of cholinergic pathways within the cerebral hemispheres of the human brain. Brain. 1998;121:2249-2257.
Staley JK, Mash DC. Adaptive increase in D3 dopamine receptors in the brain reward circuits of human cocaine fatalities. J Neurosci. 1996;16:610-616.
Van der Werf YD, Scheltens P, Lindeboom J, et al. Disorders of memory, executive functioning and attention following infarction in the thalamus: a study of 22 cases with localised lesions. Neuropsychologia. 2003;41:1330-1344.
Whelan PJ, Phelps EA. The human amygdala. New York: Guilford Press; 2009.