CHAPTER 58 Nuclear Medicine Imaging of Myocardial Viability
Coronary artery disease (CAD) remains the number one cause of mortality in the United States, responsible for nearly half of daily deaths.1 The lifetime risk of developing CAD after 40 years of age is 49% for men and 32% for women.2 In developed nations, the leading cause of left ventricular (LV) dysfunction (i.e., heart failure) is CAD.3 Retrospective analysis of 13 randomized multicenter heart failure trials showed that CAD was present in approximately 70% of the greater than 20,000 enrolled patients.4 Over the past decade, the number of patients presenting with heart failure has increased exponentially. It has been estimated that 4.7 million patients in the United States have chronic heart failure, with 550,000 new cases per year, resulting in 1 million hospitalizations.
Heart failure is the leading cause of morbidity, mortality, and hospitalization in patients older than 60 years and is the most common Medicare diagnosis-related group.5 The diagnostic and therapeutic costs associated with heart failure are estimated to be more than $29 billion per year.1 The long-term prognosis for patients with heart failure remains poor, despite advances in different therapies. More recent data from the Framingham Heart Study showed 5-year mortality rates of 59% for men and 45% for women with heart failure in the period from 1990-1999.6 Mortality rates increase in older patients with heart failure.
DEFINITION OF MYOCARDIAL VIABILITY
Before the 1980s, the conventional wisdom was that impaired LV function at rest in patients with CAD was an irreversible process. This clinical dogma was shown to be not always true when observational studies in patients with LV dysfunction undergoing coronary artery revascularization exhibited improvement in regional and global LV function.7 To explain the subsequent improvement in function, the concept of viability was introduced. Dysfunctional but viable myocardium has the potential to recover function after revascularization, whereas the revascularization of dysfunctional scar tissue does not result in improvement of function. There are several mechanisms of adaptation that the myocardium follows to maintain viability during temporary or sustained reductions in coronary blood flow.
Dysfunctional but viable myocardium has been broadly categorized as either stunned or hibernating myocardium. Stunned myocardium refers to the state of delayed recovery of regional ventricular contractile dysfunction after a transient period of ischemia that has been followed by restoration of perfusion (Fig. 58-1).8,9 Resulting dysfunction may persist for hours to days, but generally improves with time. Hibernating myocardium refers to an adaptive rather than an injurious response of the myocardium to impaired coronary flow reserve (repetitive ischemia and stunning) and reduced resting coronary blood flow (Fig. 58-2).7,9 Hibernation and stunning are categorized as different pathophysiologic states; however, these states most often exist concomitantly as a continuum in patients with dysfunctional but viable myocardium.
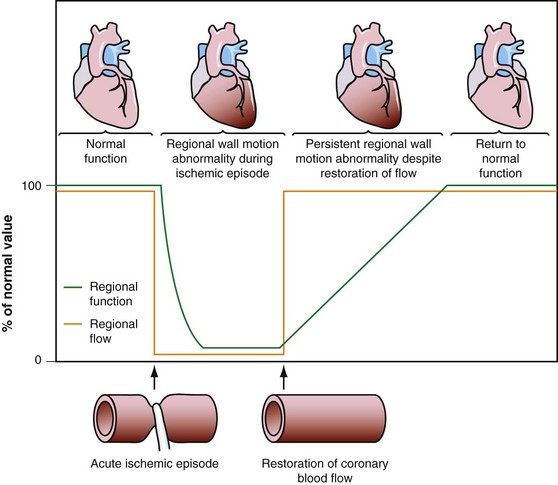
FIGURE 58-1 Schematic diagram of stunned myocardium.
(From Dilsizian V, Narula J. Nuclear investigation in heart failure and myocardial viability. In Dilsizian V, Narula J [eds]. Atlas of Nuclear Cardiology, 3rd ed. Philadelphia, Current Medicine, 2009, pp 201-224.)
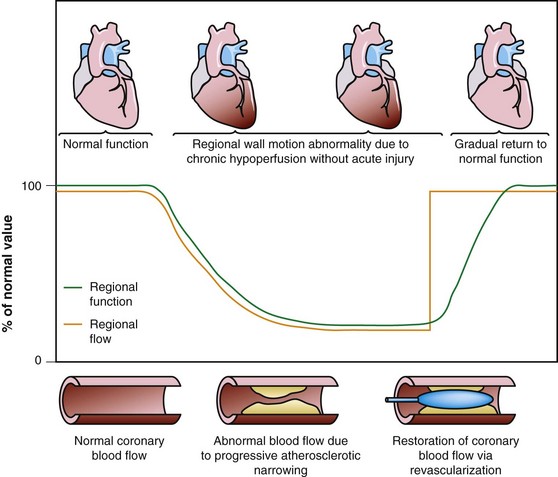
FIGURE 58-2 Schematic diagram of hibernating myocardium.
(From Dilsizian V, Narula J. Nuclear investigation in heart failure and myocardial viability. In Dilsizian V, Narula J [eds]. Atlas of Nuclear Cardiology, 3rd ed. Philadelphia, Current Medicine, 2009, pp 201-224.)
There is extensive evidence delineating the adaptations of the myocardium to reduced blood flow. Signs of energy depletion and downregulation of energy turnover have been described in hibernating myocardium.10 These alterations of energy metabolism likely cause and perpetuate contractile dysfunction, continued tissue degeneration, and subsequent cardiomyocyte loss. These responses to regional hypoperfusion are thought to preserve the minimal amount of energy needed to protect the structural and functional integrity of the cardiac myocyte. Myocardial biopsy specimens have shown disorganization of the cytoskeletal proteins, dedifferentiation (expression of more fetal proteins), and changes in the extracellular matrix with evidence of reparative fibrosis with basement membrane thickening and increased collagen fibrils and fibroblasts. Hibernating myocardium showed a loss of contractile filaments (sarcomeres), an accumulation of glycogen in the spaces previously occupied by the myofilaments, nuclei with uniformly distributed chromatin, small mitochondria, and a nearly absent sarcoplasmic reticulum.11 Additional histologic studies analyzed myocardial biopsy specimens obtained during revascularization procedures, confirming that segments with recovery after revascularization contained viable myocytes compared with the large extent of fibrosis detected in irreversibly damaged myocardium.12
Myocardial Ischemia
Imbalance between oxygen supply and oxygen demand, which is determined by regional myocardial perfusion and the rate and force of myocardial contraction, is termed ischemic myocardium. Myocardial ischemia alters myocardial substrate metabolism. As blood flow and oxygen supply decline, oxidative metabolism decreases, and glycolysis increases. As ischemia continues, glycolytic metabolism becomes overwhelmed with excess production of lactate. During states of mild ischemia, lactate continues to be removed from the myocardium by the residual blood flow, but rapidly accumulates in tissue, with a further reduction in blood flow, during more severe states of ischemia. Subsequently, increased tissue concentrations of lactate and hydrogen ions impair glycolysis, leading to loss of transmembrane ion concentration gradients, disruption of cell membranes, and cell death.13
Cell death after myocardial ischemia and reperfusion can be the consequence of apoptosis and necrosis. The induction of either apoptosis or necrosis is regulated by similar biochemical intermediates, including alterations in high-energy phosphates, intracellular calcium accumulation, and reactive oxygen species. The reduction of contractile function associated with hibernation is thought to be a protective response of the myocardium to meet minimal metabolic requirements with the reduced supply of oxygen and substrates, leading to the situation of perfusion contraction matching, preventing apoptosis and cell death.14 Histologic analysis of hibernating myocardium shows myocytes in a stable noncontractile state with intact cell membranes and cellular metabolism with little or no evidence of apoptosis.15 In contrast to programmed cell death, or apoptosis, the term programmed cell survival has been used to describe the commonality between myocardial stunning, hibernation, and ischemic preconditioning, despite their distinct pathophysiology (Fig. 58-3).16
Injury as a result of myocardial ischemia can be categorized as a continuum from fully viable, through partially viable (admixture of scarred and viable tissue), to nonviable or scarred. Not all myocardium involved in an infarction is dead or irreversibly damaged. The process of infarction starts at the endocardium and spreads toward the epicardium. The extent of myocardial infarction (MI) can be reduced when the affected vascular territory is reperfused (spontaneously, pharmacologically, or mechanically), or when the area of MI is sufficiently collateralized. Even completed infarcts vary in their transmural extent, and the epicardium is usually the most likely site of viable myocardium. The likelihood of recovery of function after revascularization is related to the extent of myocyte injury and the amount of fibrosis (Fig. 58-4).17,18
Potential End Points Used in Myocardial Viability Studies
Patients with severe LV dysfunction who undergo CABG surgery or percutaneous coronary interventions can have a considerable risk of procedure-related morbidity and mortality.18,19 Identification of patients with the potential for improvement in LV ejection fraction and survival is needed to justify the higher risk of therapeutic intervention in these patients. Revascularization of viable myocardium has been shown to improve significantly regional and global contractile function.20–22 Improvement in regional contractile function is seen in approximately one third of dysfunctional segments, and an improvement in LV ejection fraction is seen in approximately 40% of patients.21,23 Revascularization of viable myocardium has also been shown to improve significantly symptoms and New York Heart Association functional class.24 The magnitude of improvement in heart failure symptoms after CABG surgery is linearly related to the preoperative extent of myocardial viability (Fig. 58-5).24
Revascularization may also be associated with many favorable clinical effects even in the absence of ventricular functional improvement. Benefits can include relief of chest pain or heart failure symptoms, improved exercise tolerance related to diminished inducible ischemia or improved diastolic function, stabilization (or reversal) of LV remodeling, stabilization of the electrophysiologic milieu, and prevention of MI.25–27 Besides improvement in LV ejection fraction, revascularization of viable myocardium may have a beneficial effect on the prevention of arrhythmias and sudden cardiac death, which may also improve longevity. One study showed that the long-term survival rates of patients who underwent CABG surgery were similar regardless of whether LV ejection fraction increased after revascularization.28 Chronic LV dysfunction can result from nontransmural necrosis in combination with viable (normal) myocardium. The subendocardial layer contributes significantly to contraction, and subendocardial necrosis of greater than 20% of the myocardial wall results frequently in akinesia.
Revascularization of these regions may not significantly improve contractile function. The prevention of remodeling and arrhythmias may be of critical clinical relevance, however. In such patients, preserved viability in the outer layers of the myocardium could prevent progressive LV dilation, despite the lack of any improvement in resting function after revascularization. The prevention or reversal of adverse LV remodeling seems to be an important determinant of long-term natural history outcomes, and strategies that prevent or reverse remodeling generally have very favorable effects on that natural history.29
A meta-analysis of the prognostic value of viability testing and the impact of therapeutic choice on survival showed a significant association between revascularization and improved survival rate in patients with LV dysfunction and evidence of myocardial viability independent of the imaging technique used.30,31 Additionally, these studies identified a 3.2% annual death rate in patients who had viable myocardium and underwent revascularization compared with a 16% annual death rate in patients who had viable myocardium and were treated medically (Fig. 58-6). Medical treatment of heart failure has improved substantially with the introduction of angiotensin-converting enzyme (ACE) inhibitors, angiotensin II receptor blockers, and spironolactone. Patients with chronic ischemic LV dysfunction with viable myocardium have a poor prognosis when treated with medical therapy alone, however.32
NUCLEAR IMAGING TECHNIQUES FOR IDENTIFYING MYOCARDIAL VIABILITY
Single Photon Emission Computed Tomography
Tc 99m Labeled Radiotracers
Tc 99m labeled flow tracers, such as Tc 99m sestamibi and Tc 99m tetrofosmin, are lipophilic cationic complexes, which are taken up by myocytes across mitochondrial membranes and at equilibrium are retained within the mitochondria because of a large negative transmembrane potential. The initial uptake and retention of sestamibi and tetrofosmin (whether injected at rest or during stress) are flow-dependent and reflect cell membrane integrity and mitochondrial function. Myocardial ischemia interferes with mitochondrial K+-ATP channel activation, changing its mitochondrial membrane potential, and finally reducing cellular radiotracer uptake.33
Experimental studies have shown that myocardial retention of Tc 99m sestamibi and Tc 99m tetrofosmin requires cellular viability.34 Histopathologic studies have shown that tissue viability is a continuum, and that there is a nearly linear relationship with sestamibi uptake and the percentage of normal (viable) myocardium in dysfunctional regions.35 The most commonly used clinical viability criterion is the percentage of tracer uptake (frequently 50% to 60%) in dysfunctional segments. Most frequently, Tc 99m labeled tracers are injected under resting conditions, and the dysfunctional segments with tracer uptake of 50% to 60% are considered viable.36 Regional Tc 99m sestamibi or Tc 99m tetrofosmin activity has been shown to be closely correlated with the redistribution phase of rest-redistribution thallium 201 study, indicating myocardial viability (Fig. 58-7).37,38
Studies have suggested that sestamibi may underestimate myocardial viability, particularly in patients with severe LV dysfunction.39–41 Despite using quantitative techniques, studies have reported that rest sestamibi imaging underestimates myocardial viability compared with 18FDG-PET. Potential factors affecting the accuracy of sestamibi in assessing viability were determined by comparing the results of sestamibi SPECT and 18FDG-PET in patients with varying degrees of LV dysfunction. The concordance between sestamibi and 18FDG was 64% in patients with severe LV dysfunction and 78% in patients with mild to moderate LV dysfunction.42 In particular, only 42% of regions with blood flow–metabolism mismatch patterns by PET (the pattern most predictive of functional recovery after revascularization) were identified as viable by quantitative sestamibi SPECT.
Tc 99m Labeled Radiotracers with Nitrate Administration
Nitrates (intravenous, oral, or sublingual) enhance collateral blood flow and radiotracer uptake in hypoperfused myocardial regions that are subtended by severely stenosed coronary arteries. In most nitrate-enhanced studies, two sets of images are obtained: a resting image and a nitrate-enhanced image. Defect reversibility after nitrate administration (i.e., a defect filling in) is considered to be indicative of viability. Nitrate-enhanced Tc 99m perfusion tracer uptake compared with baseline is thought to be a better estimate of coronary flow reserve. Several investigators have used improvement in the regional concentration of a flow tracer injected after nitrate administration as a marker of viability.43,44
A retrospective analysis of studies using Tc 99m labeled flow tracers to assess improvement in regional function after revascularization showed a mean overall sensitivity and specificity of 81% and 66%.45 Most of these studies used a resting image, and segments were classified as viable when activity exceeded a certain threshold. Nitrate-enhanced studies, when analyzed separately, showed a higher accuracy, with a sensitivity of 86% and a specificity of 83% (Fig. 58-8).46 Studies that investigated functional outcomes showed an improvement of LV ejection fraction from 47% to 53% in patients with viable myocardium, whereas the LV ejection fraction did not change in patients without viable myocardium (40% vs. 39%). When gated SPECT regional function was added to nitrate enhancement, the accuracy of the technique for predicting recovery of function after revascularization was even higher.47,48
In patients with chronic CAD and nitrate-enhanced Tc 99m perfusion studies, superior survival was shown in patients with complete revascularization compared with patients treated with medical therapy and patients who underwent incomplete revascularization. The most important prognostic predictor of future cardiac events was the number of nonrevascularized dysfunctional regions with viable tissue on sestamibi imaging. That is, patients who had viable myocardium but who did not receive adequate revascularization had poorer prognosis.49
Thallium 201
Experimental studies have shown that extraction of thallium 201 across the cell membrane is unaffected by hypoxia, chronic hypoperfusion (hibernation), or postischemic dysfunction (stunning), unless irreversible injury (scarred myocardium) is present. Necrotic myocardium cannot retain thallium 201, and, despite its initial uptake, thallium 201 washout is accelerated in necrotic tissue.50,51 Because thallium 201 is not actively taken up in regions of fibrotic or scarred myocardium, a defect on rest images that persists on redistribution images (termed irreversible or fixed defect) represents a pattern of scarred myocardium.
Thallium 201 has been used and investigated extensively for identifying myocardial viability and hibernation, and was the first radiotracer to be used for this purpose.52 Despite the excellent flow kinetics and biologic properties, however, the low-energy gamma ray emission and long half-life of thallium 201 are potential limitations, leading to attenuation of photons, particularly in patients with a large body habitus, and higher radiation exposure compared with Tc 99m labeled perfusion tracers.
Myocardial perfusion scan clinical protocols can be used with thallium 201 with an injection of a tracer either during stress (exercise or pharmacologic) or at rest with subsequent late imaging after the redistribution of the radiotracer. The initial acquisition soon after thallium 201 injection primarily reflects delivery of the tracer through blood flow. The delayed redistribution images acquired 4 to 24 hours after radiotracer injection are a marker of sarcolemmal integrity and myocardial K+ space and Na+,K+-ATPase function, which reflects tissue viability.50,53 Delayed thallium 201 imaging for assessment of myocardial viability relies on properties of thallium 201 that allow for redistribution. Thallium 201 redistribution images represent a balance between thallium 201 washout and continued thallium 201 uptake via the active Na+,K+-ATPase transport system over time, and redistribution images reflect the distribution volume of thallium 201 as representative of the myocardial K+ space. In normal myocardium, the rate of thallium 201 washout is greater than the rate of uptake, resulting in a net thallium washout, which is potentiated further in infarcted myocardium. Redistribution imaging allows time for thallium 201 washout from necrotic myocardium, and for thallium 201 wash-in or redistribution into viable tissue.
Redistribution of thallium 201 after stress depends partly on the blood levels of thallium 201. Some ischemic but viable myocardial regions may show no redistribution on either early (3- to 4-hour) or late (24-hour) redistribution imaging, unless blood levels of thallium 201 are increased. Reinjection of 1 mCi of thallium 201 at rest immediately after either stress early (3- to 4-hour) redistribution or stress late (24-hour) redistribution studies boosts the blood level of thallium 201, potentiating the differentiation of hypoperfused and scarred myocardium from hypoperfused but viable myocardium.54
Thallium 201 Protocols for Viability Assessment
Numerous thallium 201 protocols are used clinically for the detection of myocardial viability. The following two protocols are optimized for viability detection: (1) rest-redistribution (Fig. 58-9)46,55 and (2) stress–4-hour redistribution–reinjection imaging (Fig. 58-10).54 The former assesses myocardial viability alone,56 whereas the latter assesses myocardial ischemia and viability.54 A pooled analysis of rest-redistribution and stress-redistribution-reinjection thallium studies reported high sensitivity (80% to 90%) and modest specificity (54% to 80%) for the prediction of recovery of regional function after revascularization.45 These conclusions must be viewed, however, in the context of the limitations of pooled data analysis. When taking into consideration regions with reversible defects (ischemia) and success of revascularization (re-examining regional perfusion or vessel patency after revascularization), stress-redistribution-reinjection thallium 201 imaging yields excellent positive and negative predictive accuracy (both 80% to 90%) for recovery of function after revascularization (Fig. 58-11).56
Positron Emission Tomography
Rubidium 82 is a PET perfusion radiotracer and is a cation and an analog of K+ (similar to thallium 201). The extraction of rubidium 82 from the plasma into myocardial cells is flow-dependent, whereas the washout of rubidium 82 depends on sarcolemmal membrane integrity and the Na+,K+-ATPase pump. Uptake and retention of rubidium 82 are a function of blood flow and of myocardial cell integrity (Fig. 58-12).57 The half-life of rubidium 82 is only 75 seconds, with peak energy of 3.3 MeV. It is eluted from a commercially available strontium 82 generator.
N-13 ammonia consists of neutral ammonia (NH3) in equilibrium with its charged ammonium (+NH4) ion. The neutral NH3 molecule readily diffuses across plasma and cell membranes. Inside the cell, it re-equilibrates with its ammonium form, which is trapped in glutamine via the enzyme glutamine synthase.58 The first-pass trapping of N-13 ammonia at rest is high; however, it decreases with higher flow rates. N-13 ammonia localizes in the myocardium and does not persist in the cardiac blood pool. These preferable characteristics allow better quantification of regional myocardial tracer distribution.
In contrast to N-13 ammonia, oxygen 15–water diffuses freely across plasma membranes, and with a high first-pass extraction and metabolically inert characteristics, it is theoretically better suited for blood flow measurement. The relatively short half-lives of N-13 ammonia (10 minutes) and oxygen 15–water (2 minutes) necessitate an on-site cyclotron. Oxygen 15–water does not exhibit a plateau effect at high flow rates. Additionally, the tracer persists in the cardiac blood pool and is present in the cardiac chambers and the myocardium, resulting in poor contrast, requiring subtraction of cardiac blood pool for the accurate semiquantitative and quantitative assessment. Because of these limitations, some investigators have reported heterogeneity of flow measurements.59
Estimates of myocardial blood flow by oxygen 15–water may differ from estimates by N-13 ammonia in dysfunctional myocardium. The properties of N-13 ammonia are such that a quantified average of transmural myocardial blood flow is obtained, whereas the oxygen 15–water technique measures flow only in the fraction of the myocardium that is able to exchange water rapidly and not in scar tissue.59,60 Some investigators have explored the ability of oxygen 15–water to assess myocardial viability through the modification of the blood flow information (Fig. 58-13).61 Rather than reliance on the net transmural blood flow, the volume of perfusable and nonperfusable tissue within a myocardial region was measured.60,61 When this perfusable tissue index method of oxygen 15–water was tested in patients with acute and chronic ischemic heart disease, the determination of myocardial viability was comparable to that obtained using N-13 ammonia and 18FDG metabolism.61,62
TECHNIQUE DESCRIPTIONS
Myocardial Metabolism
Fatty Acid Radiotracer Imaging
Radioiodine-labeled branched-chain fatty acid BMIPP allows the assessment of fatty acid metabolism using SPECT technology and has been extensively investigated. BMIPP is taken up by the myocyte and undergoes ATP-dependent thioesterification, but does not undergo significant mitochondrial β-oxidation (Fig. 58-14).63 As a result, BMIPP is trapped in the intracellular lipid pool. BMIPP tracks myocardial fatty acid uptake and is retained within the myocardium for longer periods, facilitating imaging. BMIPP has been studied in conjunction with perfusion radiotracers, including thallium 201, Tc 99m sestamibi, and Tc 99m tetrofosmin. Clinically, BMIPP uptake can be concordantly reduced with regional perfusion (BMIPP-perfusion match) or relatively decreased (BMIPP-perfusion mismatch). Areas with a BMIPP-perfusion mismatch have been shown to correlate with ischemia (reversible defects) on stress-redistribution thallium 201 imaging.64
Similarly, regions with BMIPP-perfusion mismatch (cold metabolic signal relative to perfusion) have been shown to correlate with 18FDG metabolic activity (hot metabolic signal relative to perfusion)—hence viability—on PET. Comparative studies support the concept that regions with BMIPP-perfusion mismatch represent areas of jeopardized, but viable myocardium. After a transient ischemic event, prolonged and persistent metabolic disturbances in fatty acid metabolism can occur for 30 hours—termed ischemic memory (Fig. 58-15).64 Corollary findings have been shown with glucose metabolism (Fig. 58-16).65–67 Such metabolic stunning, as assessed by BMIPP, has been observed in patients undergoing clinically indicated myocardial perfusion SPECT studies and in patients presenting with acute coronary syndrome.64,68
18FDG
PET imaging of myocardial metabolism is most often performed with 18FDG. 18FDG is a glucose analogue with an 18F substituted for hydroxyl (—OH) group that is taken up by the myocyte and accumulates intracellularly after initial phosphorylation by 6-hexokinase. The clinical utility of 18FDG for differentiating viable from scarred myocardium with PET was first described in the 1980s.20,69 Under aerobic conditions, the heart uses predominantly fatty acids for energy production. During conditions of myocardial ischemia, fatty acid metabolism is diminished, and glucose uptake is enhanced as the myocardium energy requirements are primarily met by glucose metabolism. As ischemia persists, increased tissue concentrations of lactate and hydrogen ions impair glycolysis, eliminating the only source of energy for the myocyte. This elimination leads to loss of transmembrane ion concentration gradients, disruption of cell membranes, and cell death. Residual glucose metabolism in dysfunctional myocardium indicates the presence of viable but functionally compromised myocardium.
18FDG-PET Clinical Protocols
In patients with diabetes mellitus (in whom supplemental intravenous insulin was not administered), only 58% of 18FDG images were of adequate quality for interpretation.70 Four common standardization schemes have been proposed to overcome this limitation: (1) oral glucose loading, (2) intravenous glucose loading, (3) hyperinsulinemic-euglycemic clamping, and (4) use of nicotinic acid derivative.
Oral Glucose Loading Protocol
Patients with clinical or subclinical diabetes mellitus can pose a problem because, owing to some degree of insulin resistance, the subsequent endogenous insulin release after glucose administration is often blunted. Decisions regarding the morning insulin dose (whether it is withheld or only half of the dose is administered), the amount of oral glucose administered, and consequent doses of intravenous insulin required to reduce the blood glucose level should be tailored for the individual diabetic patient. It has been reported that with the use of supplemental insulin, 88% of patients with diabetes could undergo successful imaging with 18FDG.70
Hyperinsulinemic-Euglycemic Clamping
To better standardize metabolic conditions during the 18FDG study, other investigators have proposed the use of hyperinsulinemic-euglycemic clamping.71 In this procedure, insulin and glucose are infused simultaneously to achieve a stable plasma insulin level of 100 to 120 IU/L and a normal plasma glucose level. The rate of glucose infusion (20% dextrose solution with potassium chloride) is adjusted intermittently on the basis of frequently measured plasma glucose levels. Although the hyperinsulinemic-euglycemic clamp technique provides excellent image quality compared with oral glucose loading, it is tedious and impractical for routine clinical studies. It has been reported that the clamp technique does not reduce the interindividual variability of 18FDG measurements compared with the oral glucose loading technique.71
Nicotinic Acid Derivative
More recently, the use of nicotinic acid derivative (e.g., acipimox) has been proposed as an alternative to the hyperinsulinemic-euglycemic clamp technique.72 Approximately 2 hours before 18FDG injection, a single dose of 250 mg of acipimox is given orally followed by glucose loading. With this technique, 18FDG image quality was shown to be comparable to that obtained after the clamp technique in the same patient population.73 Acipimox has not received FDA approval, however.
18FDG-PET Mismatch and Match Patterns
Functional Recovery
18FDG-PET provides a biologic marker of cellular viability, which is considered to be one of the most accurate noninvasive techniques for identifying viable myocardium before revascularization. In clinical practice, 18FDG is often combined with a flow tracer to assess the presence or absence of myocardial metabolism in hypoperfused myocardial regions. Preserved or enhanced glucose use in dysfunctional myocardial regions with concomitant reduction of blood flow (termed mismatch defect) is indicative of hibernating but viable myocardium (Fig. 58-17).74 Decreased (or absent) glucose use in dysfunctional myocardial regions with concomitant reduction of blood flow (termed match defect) is indicative of scarred myocardium.
In combination with myocardial perfusion, gated 18FDG-PET permits evaluation of regional myocardial metabolism and regional and global LV function. When perfusion and metabolism examinations are done in conjunction for the assessment of myocardial viability, the positive and negative predictive accuracies for a mismatch and match defect are 80% to 90% for reversibility or lack of improvement of contractile dysfunction after revascularization.17,20 Meta-analysis of studies with 18FDG used to predict improvement in regional function after revascularization showed improvement of mean LV ejection fraction from 37% to 47% in patients with viable myocardium (mismatch defect), whereas in patients without viable myocardium (matched defect), the mean LV ejection fraction remained unchanged (39% vs. 40%).75 Some myocardial regions with flow-metabolism mismatch may have prior subendocardial infarction, however, with an admixture of scarred and viable myocardium. Depending on the extent of the subendocardial damage, some of these mismatch regions may not recover contractile function after revascularization.
Prognosis
Numerous studies have evaluated the prognostic value of 18FDG-PET with regard to future cardiac events. In patients with prior MI but stable CAD, 18FDG uptake was shown to be the most important independent predictor of future cardiac events.76 In combined data analysis, the incidence of cardiac death over 1 to 2 years was 24% in patients with mismatch pattern treated with medical therapy alone compared with 10% in patients without mismatch pattern.77 The long-term survival was shown to be significantly decreased to 10% in patients with mismatch pattern (viable myocardium) who underwent revascularization compared with 42% in patients with viable myocardium who were treated with medical therapy alone.30
These long-term studies have shown a strong relationship between blood flow–metabolism mismatch pattern and subsequent development of MI or cardiac death. Critical clinical management decisions must be made in patients with high perioperative mortality imposed by the severity of LV dysfunction on one hand, and having the potential for significant improvement in symptoms, functional status, and survival after a successful revascularization on the other hand. In patients selected for revascularization on the basis of PET perfusion-metabolism mismatch classified predominantly as having viable myocardium, 1-year and 5-year survival is reported to be 70% to 80%. In contrast, when patients were managed conservatively despite the mismatch, survival was only 40% to 50%. In patients with predominantly nonviable myocardium by PET, there was no survival benefit of revascularization compared with medical therapy.78,79
Many studies have established the necessity for a critical or threshold mass of viable myocardium necessary for improvement in global LV function after revascularization. Using 18FDG-PET, in patients with two or more viable segments in a 15-segment LV model, LV ejection fraction improved from 30 ± 11% to 45 ±14%.20 In patients with ischemic cardiomyopathy (mean LV ejection fraction 28 ± 6%), the magnitude of improvement in heart failure symptoms after CABG surgery was correlated with the preoperative extent of viable myocardium as determined by PET perfusion-metabolism mismatch (see Fig. 58-5).24 Patients with evidence of viability involving 18% or more of the LV myocardium had the greatest improvement in functional status (Fig. 58-18).24 A direct correlation between the magnitude of preserved myocardial viability and the magnitude of improvement in functional status suggests that the extent of dysfunctional ischemic viable myocardium in heart failure patients can be used as a potential marker of the symptomatic benefit that would accrue as a result of revascularization. Because revascularization in patients with significant LV dysfunction is high risk, the data suggest a role for viability imaging results in prognostication and providing a signal of the potential benefit to inform the risk-benefit equation, guiding patient selection.
18FDG-SPECT
Despite the inherent resolution differences between PET and SPECT, 18FDG-SPECT shows a good agreement (94%) with 18FDG-PET.80 As with PET studies, metabolic activity measured by 18FDG-SPECT is generally interpreted in conjunction with a perfusion tracer. Dual-isotope simultaneous acquisition SPECT protocols have been developed, with 18FDG and Tc 99m perfusion tracer injected and acquired simultaneously, capitalizing on the capabilities of gamma cameras to monitor two energy peaks, assessing myocardial perfusion and glucose use and perfusion in a single acquisition.65,66
Future Opportunities for Molecular Imaging
The myocardial extracellular matrix represents a dynamic balance between the synthesis and degradation of collagen with a stable yet dynamic turnover in the normal heart. In the setting of LV remodeling, increased myocardial collagen turnover not only allows repair of damaged tissue, but also permits the muscle fiber slippage and rearrangement that precede chamber enlargement and disfiguration. Myocardial collagen degradation is regulated through the action of MMPs. Indium 111 radiolabeled and Tc 99m radiolabeled ligands have been synthesized and used to show increased MMP activity in infarcted myocardium by planar and SPECT imaging in a murine model.81
Targeting Apoptosis
Continuing cell loss, through apoptotic and necrotic pathways, contributes to adverse LV remodeling in heart failure.82 The various components of the apoptotic pathway have been well characterized, and potential molecular targets for noninvasive imaging of apoptosis have been identified. Activation of the apoptotic pathway leads to translocation of phosphatidylserine, a phospholipid normally confined to the inner aspect of the cell membrane, to the cell surface. After externalization, phosphatidylserine is bound to the phosphatidyl-binding protein annexin-V. The latter has a high affinity for binding to phosphatidylserine, and fluorescein-labeled annexin-V has been used routinely for histologic assessment of apoptosis. Tc 99m labeled annexin-V has identified cell loss after acute MI.83 In vivo imaging of apoptosis is a technique that may prove to be a valuable prognostic tool to follow patients with heart failure after revascularization or those on conservative therapy.
Targeting the Renin-Angiotensin System
The renin-angiotensin system (RAS), an adept regulator of human physiology, is frequently activated early in heart failure, and linked to LV remodeling and myocardial fibrosis through its primary effector peptide angiotensin II. Increased ACE has been seen in association with myocardial fibrosis, and inhibition of RAS modulates LV remodeling in heart failure. It is now known that the various components of RAS are locally produced in the heart, and that knowledge of the tissue expression of these enzymes and peptides could have important implications for the proper management of patients with heart failure.84,85 The discovery of the tissue RAS and its ability for local production of effector hormones (autocrine effects) has encouraged the development of PET tracers targeting ACE.86
To date, five radiolabeled ACE inhibitors have been developed, all exhibiting specific binding to the active ACE site, although certain tracers bind with higher affinity to tissue ACE than others.86–91 In explanted hearts of patients with ischemic cardiomyopathy, 18F-fluorobenzoyl-lisinopril was shown to bind specifically to myocardial tissue ACE, with the highest activity in regions adjacent to infarcted myocardium (Fig. 58-19).86 Specific ACE binding was approximately 2-fold higher than the nonspecific binding, and ACE binding in peri-infarct segments was about 1.3-fold greater than binding in remote, noninfarcted segments. A similar pattern of nonuniform distribution was observed with type 1 angiotensin II receptor (AT1R) immunoreactivity. In addition, increased ACE activity and AT1R immunoreactivity were seen in the juxtaposed areas of replacement fibrosis, consistent with their observed roles in the development of scars and remodeling of the collagen matrix in ischemic cardiomyopathy.
Lisinopril also has been successfully labeled with Tc 99m, with near-quantitative active site binding and inhibition in rats.89 If reproduced in humans, the in vivo application of these imaging techniques with either PET (for 18F-fluorobenzoyl-lisinopril) or SPECT (for Tc 99m lisinopril) would allow serial monitoring of tissue ACE activity in patients with heart failure and LV remodeling.
Targeting Autonomic Innervation
Several radiolabeled catecholamines or catecholamine analogues have been successfully used in conjunction with SPECT and PET for the evaluation of myocardial autonomic innervation. The most commonly used SPECT tracer is the radiolabeled norepinephrine analogue iodine 123–metaiodobenzylguanidine (MIBG), which competes with norepinephrine for reuptake in presynaptic vesicles, and has been successfully used to assess cardiac presynaptic sympathetic innervation. After an ischemic myocardial injury, dissociation between recovery of myocardial perfusion and myocardial innervation, as determined with MIBG, has been shown (Fig. 58-20).92 Despite considerable myocardial salvage after coronary artery reperfusion, MIBG images obtained 2 weeks after MI and reperfusion show a persistent area of myocardial denervation within the left ventricle. This area is comparable to the area of ischemic myocardium at risk, as determined by myocardial perfusion SPECT during the acute ischemic event.92
Such modifications of cardiac neuronal function have an important role in the pathophysiology of heart failure and arrhythmias. Mortality is significantly higher in heart failure patients with presynaptic sympathetic denervation, as assessed by MIBG, compared with patients with preserved MIBG uptake.93 More recent studies suggest that such characterization of the sympathetic denervation of the heart could be useful for selecting heart failure patients for β-adrenergic receptor blocker therapy.94 Another synthetic norepinephrine analogue, 11C-meta-hydroxyephedrine, has also been used as a PET tracer and has the advantage over MIBG of higher sensitivity and spatial resolution.95 In addition, PET allows for quantification and measurement of the tracer kinetics. Combined imaging with other presynaptic PET tracers, such as 11C-epinephrine and 11C-phenylephrine, could allow comprehensive evaluation of the norepinephrine uptake, storage, and metabolism in presynaptic nerve terminals.
CONCLUSION
KEY POINTS




Allman KC, Shaw LJ, Hachamovitch R, et al. Myocardial viability testing and impact of revascularization on prognosis in patients with coronary artery disease and left ventricular dysfunction: a meta-analysis. J Am Coll Cardiol. 2002;39:1151-1158.
Di Carli MF, Asgarzadie F, Schelbert HR, et al. Quantitative relation between myocardial viability and improvement in heart failure symptoms after revascularization in patients with ischemic cardiomyopathy. Circulation. 1995;92:3436-3444.
Dilsizian Dilsizian V, Narula J. Nuclear investigation in heart failure and myocardial viability. In: Dilsizian V, Narula J, editors. Atlas of Nuclear Cardiology. 3rd ed. Philadelphia: Current Medicine; 2009:201-224.
Lodge MA, Braess H, Mahmood F, et al. Developments in nuclear cardiology: transition from single photon emission computed tomography to positron emission tomography-computed tomography. J Invasive Cardiol. 2005;17:491-496.
1 Rosamond W, Flegal K, Friday G, et al. Heart disease and stroke statistics—2007 update: a report from the American Heart Association Statistics Committee and Stroke Statistics Subcommittee. Circulation. 2007;115:e69-e171.
2 Lloyd-Jones DM, Larson MG, Beiser A, et al. Lifetime risk of developing coronary heart disease. Lancet. 1999;353:89-92.
3 Bax JJ, van der Wall EE, Harbinson M. Radionuclide techniques for the assessment of myocardial viability and hibernation. Heart. 2004;90(Suppl 5):v26-v33.
4 Gheorghiade M, Bonow RO. Chronic heart failure in the United States: a manifestation of coronary artery disease. Circulation. 1998;97:282-289.
5 Massie BM, Shah NB. Evolving trends in the epidemiologic factors of heart failure: rationale for preventive strategies and comprehensive disease management. Am Heart J. 1997;133:703-712.
6 Levy D, Kenchaiah S, Larson MG, et al. Long-term trends in the incidence of and survival with heart failure. N Engl J Med. 2002;347:1397-1402.
7 Rahimtoola SH. The hibernating myocardium. Am Heart J. 1989;117:211-221.
8 Braunwald E, Kloner RA. The stunned myocardium: prolonged, postischemic ventricular dysfunction. Circulation. 1982;66:1146-1149.
9 Dilsizian V, Narula J. Nuclear investigation in heart failure and myocardial viability. In: Dilsizian V, Narula J, editors. Atlas of Nuclear Cardiology. 3rd ed. Philadelphia: Current Medicine; 2009:201-224.
10 Elsasser A, Muller KD, Skwara W, et al. Severe energy deprivation of human hibernating myocardium as possible common pathomechanism of contractile dysfunction, structural degeneration and cell death. J Am Coll Cardiol. 2002;39:1189-1198.
11 Depre C, Vanoverschelde JL, Gerber B, et al. Correlation of functional recovery with myocardial blood flow, glucose uptake, and morphologic features in patients with chronic left ventricular ischemic dysfunction undergoing coronary artery bypass grafting. J Thorac Cardiovasc Surg. 1997;113:371-378.
12 Maes A, Flameng W, Nuyts J, et al. Histological alterations in chronically hypoperfused myocardium: correlation with PET findings. Circulation. 1994;90:735-745.
13 Camici P, Ferrannini E, Opie LH. Myocardial metabolism in ischemic heart disease: basic principles and application to imaging by positron emission tomography. Prog Cardiovasc Dis. 1989;32:217-238.
14 Braunwald E, Rutherford JD. Reversible ischemic left ventricular dysfunction: evidence for the “hibernating myocardium.”. J Am Coll Cardiol. 1986;8:1467-1470.
15 Dispersyn GD, Ausma J, Thone F, et al. Cardiomyocyte remodelling during myocardial hibernation and atrial fibrillation: prelude to apoptosis. Cardiovasc Res. 1999;43:947-957.
16 Taegtmeyer H. Modulation of responses to myocardial ischemia: metabolic features of myocardial stunning, hibernation, and ischemic preconditioning. In: Dilsizian V, editor. Myocardial Viability: A Clinical and Scientific Treatise. Armonk, NY: Futura; 2000:25-36.
17 Schinkel AF, Poldermans D, Vanoverschelde JL, et al. Incidence of recovery of contractile function following revascularization in patients with ischemic left ventricular dysfunction. Am J Cardiol. 2004;93:14-17.
18 Dilsizian V. Cardiac magnetic resonance versus SPECT: are all non-infarct myocardial regions created equal? J Nucl Cardiol. 2007;14:9-14.
19 Passamani E, Davis KB, Gillespie MJ, et al. A randomized trial of coronary artery bypass surgery: survival of patients with a low ejection fraction. N Engl J Med. 1985;312:1665-1671.
20 Tillisch J, Brunken R, Marshall R, et al. Reversibility of cardiac wall-motion abnormalities predicted by positron tomography. N Engl J Med. 1986;314:884-888.
21 Bonow RO, Dilsizian V. Thallium-201 for assessment of myocardial viability. Semin Nucl Med. 1991;21:230-241.
22 Meluzin J, Cerny J, Frelich M, et al. Prognostic value of the amount of dysfunctional but viable myocardium in revascularized patients with coronary artery disease and left ventricular dysfunction. Investigators of this Multicenter Study. J Am Coll Cardiol. 1998;32:912-920.
23 Bax JJ, Visser FC, Poldermans D, et al. Relationship between preoperative viability and postoperative improvement in LVEF and heart failure symptoms. J Nucl Med. 2001;42:79-86.
24 Di Carli MF, Asgarzadie F, Schelbert HR, et al. Quantitative relation between myocardial viability and improvement in heart failure symptoms after revascularization in patients with ischemic cardiomyopathy. Circulation. 1995;92:3436-3444.
25 Bonow RO. Identification of viable myocardium. Circulation. 1996;94:2674-2680.
26 Udelson JE. Steps forward in the assessment of myocardial viability in left ventricular dysfunction. Circulation. 1998;97:833-838.
27 Dilsizian V. Myocardial viability: contractile reserve or cell membrane integrity? J Am Coll Cardiol. 1996;28:443-446.
28 Samady H, Elefteriades JA, Abbott BG, et al. Failure to improve left ventricular function after coronary revascularization for ischemic cardiomyopathy is not associated with worse outcome. Circulation. 1999;100:1298-1304.
29 Udelson JE, Konstam MA. Relation between left ventricular remodeling and clinical outcomes in heart failure patients with left ventricular systolic dysfunction. J Card Fail. 2002;8:S465-S471.
30 Allman KC, Shaw LJ, Hachamovitch R, et al. Myocardial viability testing and impact of revascularization on prognosis in patients with coronary artery disease and left ventricular dysfunction: a meta-analysis. J Am Coll Cardiol. 2002;39:1151-1158.
31 Bourque JM, Hasselblad V, Velazquez EJ, et al. Revascularization in patients with coronary artery disease, left ventricular dysfunction, and viability: a meta-analysis. Am Heart J. 2003;146:621-627.
32 Emond M, Mock MB, Davis KB, et al. Long-term survival of medically treated patients in the Coronary Artery Surgery Study (CASS) Registry. Circulation. 1994;90:2645-2657.
33 Holmuhamedov EL, Jovanovic S, Dzeja PP, et al. Mitochondrial ATP-sensitive K+ channels modulate cardiac mitochondrial function. Am J Physiol. 1998;275:H1567-H1576.
34 Takahashi N, Reinhardt CP, Marcel R, et al. Myocardial uptake of 99mTc-tetrofosmin, sestamibi, and 201Tl in a model of acute coronary reperfusion. Circulation. 1996;94:2605-2613.
35 Dakik HA, Howell JF, Lawrie GM, et al. Assessment of myocardial viability with 99mTc-sestamibi tomography before coronary bypass graft surgery: correlation with histopathology and postoperative improvement in cardiac function. Circulation. 1997;96:2892-2898.
36 Bonow RO, Dilsizian V. Thallium-201 and technetium-99m-sestamibi for assessing viable myocardium. J Nucl Med. 1992;33:815-818.
37 Udelson JE, Coleman PS, Metherall J, et al. Predicting recovery of severe regional ventricular dysfunction: comparison of resting scintigraphy with 201Tl and 99mTc-sestamibi. Circulation. 1994;89:2552-2561.
38 Matsunari I, Fujino S, Taki J, et al. Quantitative rest technetium-99m tetrofosmin imaging in predicting functional recovery after revascularization: comparison with rest-redistribution thallium-201. J Am Coll Cardiol. 1997;29:1226-1233.
39 Dilsizian V, Arrighi JA, Diodati JG, et al. Myocardial viability in patients with chronic coronary artery disease: comparison of 99mTc-sestamibi with thallium reinjection and 18F-fluorodeoxyglucose. Circulation. 1994;89:578-587.
40 Soufer R, Dey HM, Ng CK, et al. Comparison of sestamibi single-photon emission computed tomography with positron emission tomography for estimating left ventricular myocardial viability. Am J Cardiol. 1995;75:1214-1219.
41 Arrighi JA, Ng CK, Dey HM, et al. Effect of left ventricular function on the assessment of myocardial viability by technetium-99m sestamibi and correlation with positron emission tomography in patients with healed myocardial infarcts or stable angina pectoris, or both. Am J Cardiol. 1997;80:1007-1013.
42 Dilsizian V. Technetium-99m labeled myocardial perfusion tracers: blood flow or viability agents? In: Dilsizian V, editor. Myocardial Viability: A Clinical and Scientific Treatise. Armonk, NY: Futura; 2000:315-348.
43 Bisi G, Sciagra R, Santoro GM, et al. Rest technetium-99m sestamibi tomography in combination with short-term administration of nitrates: feasibility and reliability for prediction of postrevascularization outcome of asynergic territories. J Am Coll Cardiol. 1994;24:1282-1289.
44 Leoncini M, Marcucci G, Sciagra R, et al. Nitrate-enhanced gated technetium 99m sestamibi SPECT for evaluating regional wall motion at baseline and during low-dose dobutamine infusion in patients with chronic coronary artery disease and left ventricular dysfunction: comparison with two-dimensional echocardiography. J Nucl Cardiol. 2000;7:426-431.
45 Bax JJ, Poldermans D, Elhendy A, et al. Sensitivity, specificity, and predictive accuracies of various noninvasive techniques for detecting hibernating myocardium. Curr Probl Cardiol. 2001;26:147-186.
46 Dilsizian V. SPECT and PET myocardial perfusion imaging: tracers and techniques. In: Dilsizian V, Narula J, editors. Atlas of Nuclear Cardiology. 3rd ed. Philadelphia: Current Medicine; 2009:37-60.
47 Bisi G, Sciagra R, Santoro GM, et al. Technetium-99m-sestamibi imaging with nitrate infusion to detect viable hibernating myocardium and predict postrevascularization recovery. J Nucl Med. 1995;36:1994-2000.
48 Sciagra R, Bisi G, Santoro GM, et al. Comparison of baseline-nitrate technetium-99m sestamibi with rest-redistribution thallium-201 tomography in detecting viable hibernating myocardium and predicting postrevascularization recovery. J Am Coll Cardiol. 1997;30:384-391.
49 Sciagra R, Pellegri M, Pupi A, et al. Prognostic implications of Tc-99m sestamibi viability imaging and subsequent therapeutic strategy in patients with chronic coronary artery disease and left ventricular dysfunction. J Am Coll Cardiol. 2000;36:739-745.
50 Pohost GM, Zir LM, Moore RH, et al. Differentiation of transiently ischemic from infarcted myocardium by serial imaging after a single dose of thallium-201. Circulation. 1977;55:294-302.
51 Granato JE, Watson DD, Flanagan TL, et al. Myocardial thallium-201 kinetics during coronary occlusion and reperfusion: influence of method of reflow and timing of thallium-201 administration. Circulation. 1986;73:150-160.
52 Akins CW, Pohost GM, Desanctis RW, et al. Selection of angina-free patients with severe left ventricular dysfunction for myocardial revascularization. Am J Cardiol. 1980;46:695-700.
53 Kiat H, Berman DS, Maddahi J, et al. Late reversibility of tomographic myocardial thallium-201 defects: an accurate marker of myocardial viability. J Am Coll Cardiol. 1988;12:1456-1463.
54 Dilsizian V, Rocco TP, Freedman NM, et al. Enhanced detection of ischemic but viable myocardium by the reinjection of thallium after stress-redistribution imaging. N Engl J Med. 1990;323:141-146.
55 Ragosta M, Beller GA, Watson DD, et al. Quantitative planar rest-redistribution 201Tl imaging in detection of myocardial viability and prediction of improvement in left ventricular function after coronary artery bypass surgery in patients with severely depressed left ventricular function. Circulation. 1993;87:1630-1641.
56 Kitsiou AN, Srinivasan G, Quyyumi AA, et al. Stress-induced reversible and mild-to-moderate irreversible thallium defects: are they equally accurate for predicting recovery of regional left ventricular function after revascularization? Circulation. 1998;98:501-508.
57 Lodge MA, Braess H, Mahmood F, et al. Developments in nuclear cardiology: transition from single photon emission computed tomography to positron emission tomography-computed tomography. J Invasive Cardiol. 2005;17:491-496.
58 Schelbert HR, Phelps ME, Huang SC, et al. N-13 ammonia as an indicator of myocardial blood flow. Circulation. 1981;63:1259-1272.
59 Nitzsche EU, Choi Y, Czernin J, et al. Noninvasive quantification of myocardial blood flow in humans: a direct comparison of the [13N]ammonia and the [15O]water techniques. Circulation. 1996;93:2000-2006.
60 Iida H, Rhodes CG, de Silva R, et al. Myocardial tissue fraction: correction of partial volume effects and measure of tissue viability. J Nucl Med. 1991;32:2169-2175.
61 Yamamoto Y, de Silva R, Rhodes CG, et al. A new strategy for the assessment of viable myocardium and regional myocardial blood flow using 15O-water and dynamic positron emission tomography. Circulation. 1992;86:167-178.
62 Gerber BL, Melin JA, Bol A, et al. Nitrogen-13-ammonia and oxygen-15-water estimates of absolute myocardial perfusion in left ventricular ischemic dysfunction. J Nucl Med. 1998;39:1655-1662.
63 Messina SA, Aras O, Dilsizian V. Delayed recovery of fatty acid metabolism after transient myocardial ischemia: a potential imaging target for “ischemic memory.”. Curr Cardiol Rep. 2007;9:159-165.
64 Dilsizian V, Bateman TM, Bergmann SR, et al. Metabolic imaging with beta-methyl-p-[(123)I]-iodophenyl-pentadecanoic acid identifies ischemic memory after demand ischemia. Circulation. 2005;112:2169-2174.
65 He ZX, Shi RF, Wu YJ, et al. Direct imaging of exercise-induced myocardial ischemia with fluorine-18-labeled deoxyglucose and Tc-99m-sestamibi in coronary artery disease. Circulation. 2003;108:1208-1213.
66 Dou KF, Yang MF, Yang YJ, et al. Myocardial 18F-FDG uptake after exercise-induced myocardial ischemia in patients with coronary artery disease. J Nucl Med. 2008;49:1986-1991.
67 Dilsizian V. FDG Uptake as a surrogate marker for antecedent ischemia. J Nucl Med. 2008;49:1909-1911.
68 Kawai Y, Tsukamoto E, Nozaki Y, et al. Significance of reduced uptake of iodinated fatty acid analogue for the evaluation of patients with acute chest pain. J Am Coll Cardiol. 2001;38:1888-1894.
69 Marshall RC, Tillisch JH, Phelps ME, et al. Identification and differentiation of resting myocardial ischemia and infarction in man with positron computed tomography, 18F-labeled fluorodeoxyglucose and N-13 ammonia. Circulation. 1983;67:766-778.
70 Gropler RJ. Methodology governing the assessment of myocardial glucose metabolism by positron emission tomography and fluorine 18-labeled fluorodeoxyglucose. J Nucl Cardiol. 1994;1:S4-S14.
71 Knuuti MJ, Nuutila P, Ruotsalainen U, et al. Euglycemic hyperinsulinemic clamp and oral glucose load in stimulating myocardial glucose utilization during positron emission tomography. J Nucl Med. 1992;33:1255-1262.
72 Knuuti MJ, Yki-Jarvinen H, Voipio-Pulkki LM, et al. Enhancement of myocardial [fluorine-18]fluorodeoxyglucose uptake by a nicotinic acid derivative. J Nucl Med. 1994;35:989-998.
73 Bax JJ, Veening MA, Visser FC, et al. Optimal metabolic conditions during fluorine-18 fluorodeoxyglucose imaging: a comparative study using different protocols. Eur J Nucl Med. 1997;24:35-41.
74 Taegtmeyer H, Dilsizian V. Imaging myocardial metabolism and ischemic memory. Nat Clin Pract Cardiol. 2008;5:S42-S48.
75 Maddahi J, Schelbert H, Brunken R, et al. Role of thallium-201 and PET imaging in evaluation of myocardial viability and management of patients with coronary artery disease and left ventricular dysfunction. J Nucl Med. 1994;35:707-715.
76 Tamaki N, Kawamoto M, Takahashi N, et al. Prognostic value of an increase in fluorine-18 deoxyglucose uptake in patients with myocardial infarction: comparison with stress thallium imaging. J Am Coll Cardiol. 1993;22:1621-1627.
77 Schelbert HR. 18F-deoxyglucose and the assessment of myocardial viability. Semin Nucl Med. 2002;32:60-69.
78 Eitzman D, al Aouar Z, Kanter HL, et al. Clinical outcome of patients with advanced coronary artery disease after viability studies with positron emission tomography. J Am Coll Cardiol. 1992;20:559-565.
79 Di Carli MF, Davidson M, Little R, et al. Value of metabolic imaging with positron emission tomography for evaluating prognosis in patients with coronary artery disease and left ventricular dysfunction. Am J Cardiol. 1994;73:527-533.
80 Srinivasan G, Kitsiou AN, Bacharach SL, et al. [18F]fluorodeoxyglucose single photon emission computed tomography: can it replace PET and thallium SPECT for the assessment of myocardial viability? Circulation. 1998;97:843-850.
81 Su H, Spinale FG, Dobrucki LW, et al. Noninvasive targeted imaging of matrix metalloproteinase activation in a murine model of postinfarction remodeling. Circulation. 2005;112:3157-3167.
82 Grazette LP, Rosenzweig A. Role of apoptosis in heart failure. Heart Fail Clin. 2005;1:251-261.
83 Hofstra L, Liem IH, Dumont EA, et al. Visualisation of cell death in vivo in patients with acute myocardial infarction. Lancet. 2000;356:209-212.
84 Paul M, Poyan MA, Kreutz R. Physiology of local renin-angiotensin systems. Physiol Rev. 2006;86:747-803.
85 Shirani J, Narula J, Eckelman WC, et al. Novel imaging strategies for predicting remodeling and evolution of heart failure: targeting the renin-angiotensin system. Heart Fail. Clin. 2006;2:231-247.
86 Dilsizian V, Eckelman WC, Loredo ML, et al. Evidence for tissue angiotensin-converting enzyme in explanted hearts of ischemic cardiomyopathy using targeted radiotracer technique. J Nucl Med. 2007;48:182-187.
87 Hwang DR, Eckelman WC, Mathias CJ, et al. Positron-labeled angiotensin-converting enzyme (ACE) inhibitor: fluorine-18-fluorocaptopril: probing the ACE activity in vivo by positron emission tomography. J Nucl Med. 1991;32:1730-1737.
88 Lee YHC, Kiesewetter DO, Lang L, et al. Synthesis of 4-[18F]fluorobenzoyllisinopril: a radioligand for angiotensin converting enzyme (ACE) imaging with positron emission tomography. J Labelled Cpd Radiopharm. 2001;44:S268-S270.
89 Femia FJ, Maresca KP, Hillier SM, et al. Synthesis and evaluation of a series of 99mTc(CO)3+ lisinopril complexes for in vivo imaging of angiotensin-converting enzyme expression. J Nucl Med. 2008;49:1-8.
90 Fyhrquist F, Tikkanen I, Grönhagen-Riska C, et al. Inhibitor binding assay for angiotensin-converting enzyme. Clin Chem. 1984;30:696-700.
91 Matarrese M, Salimbeni A, Turolla EA, et al. 11C-Radiosynthesis and preliminary human evaluation of the disposition of the ACE inhibitor [11C]zofenoprilat. Bioorg Med Chem. 2004;12:603-611.
92 Matsunari I, Schricke U, Bengel FM, et al. Extent of cardiac sympathetic neuronal damage is determined by the area of ischemia in patients with acute coronary syndromes. Circulation. 2000;101:2579-2585.
93 Merlet P, Valette H, Dubois-Rande JL, et al. Prognostic value of cardiac metaiodobenzylguanidine imaging in patients with heart failure. J Nucl Med. 1992;33:471-477.
94 Fukuoka S, Hayashida K, Hirose Y, et al. Use of iodine-123 metaiodobenzylguanidine myocardial imaging to predict the effectiveness of beta-blocker therapy in patients with dilated cardiomyopathy. Eur J Nucl Med. 1997;24:523-529.
95 Raffel DM, Jung YW, Gildersleeve DL, et al. Radiolabeled phenethylguanidines: novel imaging agents for cardiac sympathetic neurons and adrenergic tumors. J Med Chem. 2007;50:2078-2088.