6 Neurons and neuroglia
Overview
Neurons
In the central nervous system (CNS), almost all neurons are multipolar, their cell bodies or somas having multiple poles or angular points. At every pole but one, a dendrite emerges and divides repeatedly (Figure 6.1). On some neurons, the shafts of the dendrites are smooth. On others, the shafts show numerous short spines (Figure 6.2). The dendrites receive synaptic contacts from other neurons, from some on the spines and from others on the shafts.
The remaining pole of the soma gives rise to the axon, which conducts nerve impulses. Most axons give off collateral branches (Figure 6.3). Terminal branches synapse on target neurons.
Internal structure of neurons
All parts of neurons are permeated by microtubules and neurofilaments (Figure 6.4). The soma contains the nucleus and the cytoplasm or perikaryon (Gr.’around the nucleus’). The perikaryon contains clumps of granular endoplasmic reticulum known as Nissl bodies (Figure 6.5), also Golgi complexes, free ribosomes, mitochondria, and smooth endoplasmic reticulum (Figure 6.4).
Transport mechanisms
Microtubules are the supporting structures for neuronal transport. Microtubule-binding proteins, in the form of ATPases, propel organelles and molecules along the outer surface of the microtubules. Distinct ATPases are used for anterograde and retrograde work. Retrograde transport of signaling endosomes is performed by the dynein ATPase. Failure of dynein performance has been linked to motor neuron disease, described in Chapter 16.
Some points of clinical relevance are highlighted in Clinical Panel 6.1.
Clinical Panel 6.1 Clinical relevance of neuronal transport
Tetanus
Wounds contaminated by soil or street dust may contain Clostridium tetani. The toxin produced by this organism binds to the plasma membrane of nerve endings, is taken up by endocytosis, and is carried to the spinal cord by retrograde transport. Other neurons upstream take in the toxin by endocytosis – notably Renshaw cells (Ch. 15), which normally exert a braking action on motor neurons through the release of an inhibitory transmitter substance, glycine. Tetanus toxin prevents the release of glycine. As a result, motor neurons go out of control, particularly those supplying the muscles of the face, jaws, and spine. These muscles exhibit prolonged, agonizing spasms. About half of the patients who show these classic signs of tetanus die of exhaustion within a few days. Tetanus is entirely preventable by appropriate and timely immunization.
Synapses
Synapses are the points of contact between neurons.
Chemical synapses
Conventional synapses are chemical, depending for their effect on the release of a transmitter substance. The typical chemical synapse comprises a presynaptic membrane, a synaptic cleft, and a postsynaptic membrane (Figure 6.6). The presynaptic membrane belongs to the terminal bouton, the postsynaptic membrane to the target neuron. Transmitter substance is released from the bouton by exocytosis, traverses the narrow synaptic cleft, and activates receptors in the postsynaptic membrane. Underlying the postsynaptic membrane is a subsynaptic web, in which numerous biochemical changes are initiated by receptor activation.
The bouton contains synaptic vesicles loaded with transmitter substance, together with numerous mitochondria and sacs of SER (Figure 6.7). Following conventional methods of fixation, presynaptic dense projections are visible, and microtubules seem to guide the synaptic vesicles to active zones in the intervals between the projections.
Receptor activation
Transmitter molecules cross the synaptic cleft and activate receptor proteins that straddle the postsynaptic membrane (Figure 6.8). The activated receptors initiate ionic events that either depolarize the postsynaptic membrane (excitatory postsynaptic effect) or hyperpolarize it (inhibitory postsynaptic effect). The voltage change passes over the soma in a decremental wave called electrotonus, and alters the resting potential of the first part or initial segment of the axon. (See Ch. 7 for details of the ionic events.) If excitatory postsynaptic potentials are dominant, the initial segment will be depolarized to threshold and generate action potentials.
The sequence of events involved in glutamatergic synaptic transmission is shown in Figure 6.8A. In the case of peptide cotransmission with glutamate, release of (one or more) peptides is non-synaptic, as shown in Figure 6.8B.
A further kind of transmission is known as volume transmission. This kind is typical of monoamine (biogenic amine) neurons, which fall into two categories. One category synthesizes a catecholamine, namely norepinephrine (noradrenaline) or dopamine, both synthesized from the amino acid tyrosine. The other synthesizes serotonin, derived from tryptophan. As illustrated in Figure 6.9, for dopamine, the transmitter is liberated from varicosities (where they are also synthesized) as well as from synaptic contacts. The transmitter enters the extracellular fluid of the CNS and activates specific receptors up to 100 μm away before being degraded. The monoamine neurons have enormous territorial distribution, and deviation from normal function is implicated in a variety of ailments including Parkinson disease, schizophrenia, and major depression.
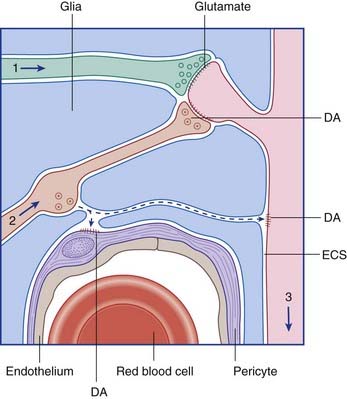
Figure 6.9 Volume transmission in the brain. The axons of a glutamatergic neuron (1) and of a dopaminergic neuron (2) are making conventional synaptic contacts on the spine of a spiny stellate cell (3) in the striatum. Dopamine (DA) is also escaping from a varicosity and diffusing through the extracellular space (ECS) to activate dopamine receptors on the dendritic shaft and on the wall of a capillary pericyte (see Ch. 5).
Inhibition versus disinhibition
Spontaneously active neurons are often held in check by inhibitory neurons (usually GABAergic), as shown in Figure 6.10A. The inhibitory neurons may be silenced by others of the same kind, leading to disinhibition of the target cell (Figure 6.10B). Disinhibition is a major feature of neuronal activity in the basal ganglia (Ch. 33).
Less common chemical synapses
Two varieties of axoaxonic synapses are recognized. In both cases, the boutons belong to inhibitory neurons. One variety occurs on the initial segment of the axon, where it exercises a powerful veto on impulse generation (Figure 6.11). In the second kind, the boutons are applied to excitatory boutons of other neurons, and they inhibit transmitter release. The effect is called presynaptic inhibition, any conventional contact being postsynaptic in this context (Figure 6.12).
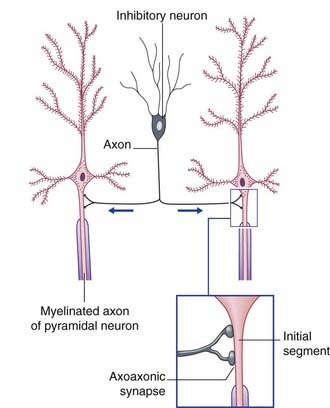
Figure 6.11 Axoaxonic synapses in the cerebral cortex. Arrows indicate direction of impulse conduction.
Dendrodendritic (D-D) synapses occur between dendritic spines of contiguous spiny neurons, and alter the electrotonus of the target neuron rather than generating nerve impulses. In one-way D-D synapses, one of the two spines contains synaptic vesicles. In reciprocal synapses, both do. Excitatory D-D synapses are shown in Figure 6.13. Inhibitory D-D synapses are numerous in relay nuclei of the thalamus (Ch. 25).
Somatodendritic and somatosomatic synapses have also been identified, but they are scarce.
Neuroglial Cells of the Central Nervous System
Astrocytes
Some astrocyte processes form glial-limiting membranes on the inner (ventricular) and outer (pial) surfaces of the brain. Other processes invest synaptic contacts between neurons. In addition, vascular processes invest brain capillaries (Figure 6.14).
Astrocytes use specific channels (Ch. 8) to mop up K+ ion accumulation in the extracellular space during periods of intense neuronal activity. They participate in recycling certain neurotransmitter substances following release, notably the chief excitatory CNS transmitter, glutamate, and the chief inhibitory transmitter, GABA.
Astrocytes can multiply at any time. As part of the healing process following CNS injury, proliferation of astrocytes and their processes results in dense glial scar tissue (gliosis). More importantly, spontaneous local proliferation of astrocytes may give rise to a brain tumor (Clinical Panel 6.2).
Clinical Panel 6.2 Gliomas
Brain tumors most commonly originate from neuroglial cells, especially astrocytes.
Progression
Expansion of a tumor may cause one or more brain hernias to develop, as shown in Figure CP 6.2.1.
Oligodendrocytes
Myelination
Myelination commences during the middle period of gestation, and continues well into the second decade. A single oligodendrocyte lays myelin on upward of three dozen axons by means of a spiraling process whereby the inner and outer faces of the plasma membrane form the alternating major and minor dense lines seen in transverse sections of the myelin sheath (Figure 6.15). Some cytoplasm remains in paranodal pockets at the ends of each myelin segment. In the intervals between the glial wrappings, the axon is relatively exposed, at nodes.
Myelination greatly increases the speed of impulse conduction, because the depolarization process jumps from node to node (see Ch. 9). During myelination, K+ ion channels are deleted from the underlying axolemma. For this reason, demyelinating diseases such as multiple sclerosis (Clinical Panel 6.3) are accompanied by progressive failure of impulse conduction.
Clinical Panel 6.3 Multiple sclerosis
Multiple sclerosis is a primary demyelinating disease: the initial feature is the development of plaques (patches) of demyelination in the white matter. The denuded axons also undergo large-scale degeneration, probably initiated by failure of the sodium pump, as described in Chapter 7. Impulse conduction in neighboring myelinated fibers is also compromised by edema (inflammatory exudate). Over time, the plaques are progressively replaced by glial scar tissue. Old plaques feel firm (sclerotic) in postmortem slices of the brain.
Common locations of early plaques are the cervical spinal cord, upper brainstem, optic nerve, and periventricular white matter (Figure CP 6.3.1) including that of the cerebellum. MS is not a systems disease: it is not anatomically selective, and a plaque may involve parts of adjacent motor and sensory pathways.
Presenting symptoms can be correlated with lesion sites as follows.
Gage FH, Kempermann G, Song H, editors. Adult neurogenesis. Cold Spring Harbor, New York: Cold Spring Harbor Laboratory Press, 2008.
Hirokawa N, Reiko T. Molecular motors in neuronal development, intracellular transport and diseases. Curr Opin Neurobiol. 2004;14:564-573.
Howe CH, Mobley WC. Long-distance retrograde neurotrophic signaling. Curr Opin Neurobiol. 2005;15:40-48.
Jessen KR. Glial cells. Int J Biochem Cell Biol. 2004;36:1861-1867.
Koehler RC, Roman RJ, Harder GR. Astrocytes and the regulation of cerebral blood flow. Trends Neurosci. 2009;32:160-169.
Pannese E. Neurocytology. Fine structure of neurons, nerve processes and neuroglial cells. New York: Thieme; 1994.
Stys PK. General mechanisms of axonal damage and its repair. J Neurol Sci. 2005;233:3-13.
Sykova E, Nicholson C. Diffusion in brain extracellular space. Physiol Rev. 2008;88:1277-1340.
Torrealba F, Carrasco MA. A review on electron microscopy and neurotransmitter systems. Brain Res Rev. 2004;47:5-17.
Triarhou LC. Cellular structure of the cerebral cortex. Basle: Karger; 2009.