6 Neuronal integration and movement
Case 6.1
Questions
• 6.1.1 What neurological structures are involved in the creation of motivation to move?
• 6.1.2 How is this thought process transformed into mechanical movement of muscles?
• 6.1.3 Based on neural pathways and integrative circuits, what theory can be formed connecting his declining activity level and his lack of motivation?
Postures and movements are controlled by a hierarchy of systems
Postures and movements are controlled simultaneously by different levels of nervous organisation including the cortex (cognitive control), the sensory system (sensory control), and the emotional system (emotive control). These levels of the organisation first suggested by Jackson are classified into a vague three-tier system (Jackson 1882).
The highest levels of cognition are concerned with the relevance and importance of the task to the present situation. This analysis seems to occur prior to communicating with the lower levels of the hierarchy. The ‘cognitive ‘component is composed of sensory, motor, and associative systems and the ‘emotive’ component is largely composed of limbic circuits (Fig. 6.1).
Limbic and hypothalamic involvement in movement
The limbic system has been traditionally described as involving a complex network of neural circuitry composed of the parahippocampal gyrus, the cingulated gyrus, the subcallosal gyrus (which is the anterior and inferior continuation of the cingulate gyrus), the hippocampal formation (which includes the hippocampus proper, the dentate gyrus, and the subiculum), various nuclei of the septal region, the nucleus accumbens (which is an extension of the caudate nucleus), neocortical areas such as the orbital frontal cortex, subcortical structures such as the amygdala, and various nuclei of the hypothalamus (Iversen et al. 2000) (Fig. 6.2).
The hypothalamus contributes to limbic system function primarily through controlling influences on the pituitary gland. Neurons in the medial basal region of the hypothalamus release peptide neurohormones that act as stimulators or releasing factors that act on the cells of the anterior pituitary gland or adenohypophysis. The pituitary cells then release a variety of hormones including luteinizing hormone (LH), the growth hormone (GH) somatotrophin, adrenocorticotrophic hormone (ACTH), thyroid stimulating hormone (TSH), follicle-stimulating hormone (FSH), and prolactin. Axons of neurons in the supraoptic and paraventricular nuclei release the neurohormone oxytocin and the antidiuretic hormone vasopressin (Fig. 6.3).
Neurons in a variety of hypothalamic nuclei also project to the intramedial lateral (IML) cell columns of the spinal cord grey regions where they modulate the activity of the preganglionic neurons of the sympathetic nervous system, which control a variety of functions including blood flow to virtually all areas of the body. This pathway is important in modulating blood flow to various muscle groups and organs including the brain, prior to and during movement. The control of the blood flow to the hypothalamus arises from postganglionic sympathetic neurons located in the superior cervical ganglion, which are under the influence of the hypothalamus itself (Fig. 6.4).
The development of motivational drives
The limbic system is deeply involved in the creation of motivational states or drives that modulate the central integrative states of neurons in wide-ranging areas of the central nervous system (CNS) that produce a variety of behavioural responses such as movement, temperature regulation, active procurement of food, sexual drive, emotional context, and curiosity (Swanson & Mogenson 1981; Brooks 1984; Kupfermann et al. 2000).
The majority of the neurons in the inferior and posterior regions of the intraparietal sulcus (Brodmann area 7) show an early response to sensory cues that relate to the execution of movement (Mountcastle et al. 1975). Smaller numbers of neurons in area 7 exhibited more complex response patterns, where activation only occurred in specific situations where a number of variables were met simultaneously, e.g. sight of food and the presence of hunger (Fig. 6.5).
Corticoneostriatal and corticopontine projections
The judgement system seems to consist of a series of complex gate systems of ‘and’ or ‘or’ gates that are both involved directly in gating inputs and are indirectly gated themselves by being involved in the more complex array of interactions involved in the complete execution command required. This complex array of gated pathways in the association cortex projects directly to the motor cortex via association fibres as well as to the striatum and pontine nuclei. These projections to the striatum and pontine nuclei further project to other areas of the nervous system and form indirect pathway projection loops from the association cortex to the motor cortex (Rolls 1983; deZeeuw et al. 1997).
The first indirect projection loop involves the striatum (caudate nucleus and putamen), whose output nuclei the globus pallidus pars interna projects through the anterior division of the thalamic fasciculus to the pars oralis area of the ventral lateral and ventral anterior thalamic nuclei. Neurons of the ventral lateral thalamic nuclei project their axons to the ipsilateral motor, premotor, and parietal cortical areas (Fig. 6.6).
The second indirect projection loop involves projections to the ipsilateral pontine nuclei via the corticopontine projection fibres, which in turn project to two output nuclei of the contralateral cerebellum via the pontocerebellar fibre system. These deep cerebellar nuclei, the dentate, and the interposed nuclei (emboliform and globose nuclei) then project to the contralateral pars caudalis area of the ventrolateral thalamic nuclei via the posterior division of the thalamic fasciculus. Neurons of the ventrolateral thalamic nuclei project their axons to the ipsilateral motor, premotor, and parietal cortical areas (Fig. 6.7).
The basal ganglionic loops
The basal ganglionic loops involve two distinct pathways. The first involves input pathways to the caudate nucleus (see Fig. 6.6); the second involves input pathways through the putamen (see Fig. 6.7). For instance, the caudate nuclei receive inputs from many areas of cortex including the association regions of the frontal, parietal, and temporal lobes. Most of the afferent or return projections from this loop arise from the globus pallidus pars interna of the basal ganglia and project to the ventral anterior nuclei of the thalamus. Neurons in the ventral anterior nuclei of the thalamus project to the premotor cortex (Brodmann area 6).
The second pathway of the basal ganglionic loop involving the putamen receives the majority of its input from the sensorimotor cortex (Brodmann areas 3, 2, 1, and 5) and projects via the globus pallidus pars interna to the ventrolateral nuclear group of the thalamus. The ventrolateral group of thalamic neurons then project to premotor areas including Brodmann area 6 (Delong et al. 1983). This projection loop is very important for basal ganglionic modulation of motor cortical output since the basal ganglia do not possess direct projections from the thalamus to primary motor cortex (Schell & Strick 1984).
The pontine and cerebellar loops receive the majority of their inputs from the primary motor (area 5), somatosensory areas (areas 4, 3, 2, and 1), and some input from association and visual areas (areas 17 and 18). Very little input to the pontine and cerebellar loops comes from high-level association areas (Rolls 1983).
The thalamocortical projections
All of the indirect loops described above contain reciprocal innervation pathways above the thalamus (thalamus to cortex, cortex to thalamus) and only unidirectional pathways below the thalamus (basal ganglia to thalamus, pontine nuclei to thalamus) (Sherman & Guillery 2001) (Fig. 6.8). In fact, the thalmocortical projections form a loop in which the ‘feedforward’ portion is part of the classic pathway relaying sensory information to the cortex and the ‘feedback’ portion is composed of cortical control or modulation over thalamic operation. This feedback control can be either excitatory or inhibitory depending on the central integrative state of the cortex and is modulated itself by sensory input from the thalamus (Llinas 1991; Destexhe 2000). This results in a variety of intrinsic thalamocortical and corticothalamic oscillations that can be observed via electroencephalograph (EEG) and quanitative electroencephalograph (qEEG) recordings over the cortex (Destexhe & Sejnowski 2003).
A brief summary thus far
To summarise thus far using our example of a pinch grasp of an object between the finger and thumb, the neural activity involved depends on the motivation for the movement in the first place. Is the object food and is the subject hungry? Is the subject just curious as to what the object is? The pathways through which movement is initiated depend on a number of variables such as prelearning of movement components, the relevance and importance of the movement at the time, and whether a variety of movement choices must be considered. Once initiated, the plastic nature of the system provides a multitract system (basal ganglionic and pontine/cerebellar) which can consider a multitude of physical variables as well as the initial motivation in order to complete the desired movement.
Formulation of the ‘how to’ instructions involves the premotor cortex (area 6), which acts as an organiser for the principal output area, the primary motor cortex (area 4) (Brooks & Thach 1981).
Instructions for acts of different complexity enter the middle-level areas via separate routes. For example, motor programmes for complex movement patterns enter from the caudate circuit of the basal ganglia, whereas instructions for less complex actions enter the premotor area via the putamen circuit of the basal ganglia as well as the lateral cerebellum pontine nuclei circuit (Brooks 1984).
Changes in neuron potentials have been recorded over the motor cortex 800 ms before the onset of voluntary movement (Krakauer & Ghez 2000). In this period before the onset of movement the higher centres seem to be presetting the neuron response patterns in relation to the specific preset programmes that specify the intended movement. The set of preset programmes that describe a specific movement are referred to as a motor set.
Output commands from the motor cortex
The output instructions from the motor cortex are relayed through two different component systems. The first component arises from the postcentral cortical areas and projects through the pyramidal and extrapyramidal pathways to areas in the dorsal grey matter of the spinal cord where modulation of the central integrative state of spinal dorsal horn cells takes place. The second component is the slower precentral system, which modulates the central integrative state of alpha motor neurons in the anterior horn of the grey matter via the axons of the corticospinal tracts (Brooks 1984).
The organisation of the neurons in the cortex of area 4 is somatotopic due to the extensive and specific synapses formed on the spinal motor neurons via the corticospinal tracts. This somatotopic representation is known as the motor homunculus of man (Fig. 6.9). Neurons in the somatosensory cortex receive information in a somatotopic array called the sensory homunculus of man (Fig. 6.10).
The neurons of the motor cortex are grouped into arrays of perpendicular columns called cortical efferent zones or motor columns. Axons from the thalamus and other cortical areas terminate in the superficial layers of the cortical efferent zones by synapsing with dendrites of the pyramidal neurons and on a wide variety of cortical interneurons. These interneurons then send impulses in a cascading fashion to other interneurons radially down the cortical columns where they finally reach the pyramidal and large pyramidal neurons. The pyramidal neurons have different adaptation patterns which seem to depend on the diameter of their axons. Pyramidal neurons with larger-diameter axons tend to fire when transient high frequency input is received and adapt very quickly to this stimulus, whereas smaller-diameter axons respond to low frequency input and adapt slowly (Krakauer & Ghez 2000).
The fine tuning of motor control and the basal ganglia
The basal ganglia are a group of nuclei situated deep in the brain at the junction of telencephalic and diencephalic functional areas. These nuclei include the caudate and putamen, which are composed of similar cell types and fused anteriorly, the globus pallidus, the subthalamic nuclei, and the substantia nigra (Anderson et al. 2003). All input to the basal ganglia projects onto either the caudate nucleus or the putamen. The input arises from four main areas which include wide areas of motor and association cortex, the midline intralaminar thalamic nuclei, the zona compacta of the substantia nigra, and the raphe nuclei of the midbrain. The basal ganglia do not project directly to neurons that would allow them to effect control of motor activity but seems to act in a coordination role between the cortex and the thalamus by filtering or gating the information stream that the thalamus receives from the periphery (Fig. 6.11). The basal ganglia are also involved in the coordination of movement necessary in the maintenance of static postures and the initiation of controlled or detailed movements (Martin 1967; Kornhuber 1971).
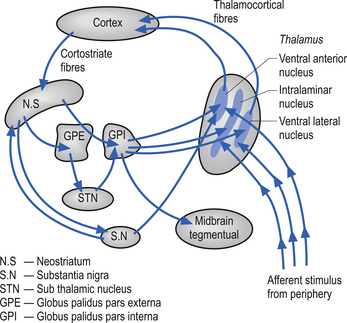
Figure 6.11 Diagram outlining the gating action of the basal ganglia on the thalamus. There are two predominant pathways (direct and indirect) from the neostriatum to the output nuclei of the basal ganglia, the globus pallidus pars internus (GPi), and the substantia nigra pars reticulate (SN). The direct pathway involves the neostriatal projections to the GPi, which in turn projects to the thalamic nuclei. The indirect pathway involves the neostriatal projections to the globus pallidus pars externus (GPe). The GPe then projects to the subthalamic nuclei, which in turn projects to the GPi. The GPi then completes the loop to the thalamus. Because of the different neurotransmitters released through the loops, they each result in a different modulatory activity on the thalamus (see Chapter 11).
The final common pathway and supraspinal modulation
The second group of pathways arises from neurons in the cortex and the red nucleus and descends in the contralateral lateral funiculi of the white matter. These axons terminate in the intermediate zone of grey matter and on the motor neurons innervating the more distal muscles. The tracts of this group include the lateral corticospinal tract and the rubrospinal tract. The corticospinal tracts arise from the pyramidal neurons of the sensory–motor cortex and are characteristically focused on a small number of muscle groups and are thus suited for delicate fine movement control. Another pathway that arises from the pyramidal neurons in the motor cortex descends ipsilaterally in the ventromedial area and is called the ventral corticospinal tract. This tract is mostly involved in intricate control of postural muscles of the spinal column (Fig. 6.12).
In order for meaningful supraspinal coordination of movement to occur the intention command and the actual movement performed must be compared and evaluated for accuracy. This is accomplished via feedforward activation, collateral activation, and feedback mechanisms. This requires that both output information and feedback information are accurate and quickly corrected when they are not accurate. Correction of variances between the command and the actual performance is initially evaluated by a mechanism referred to as the motor servo loop. This loop involves feedback from the muscle spindle via the dorsal root ganglion cell to the alpha motor neuron innervating the same muscle. The muscle servo loop is usually self-correcting but is under the modulatory control of supraspinal neurons, which can alter the balance of the servo loop in either direction (Fig. 6.13).
Summary
Movement involves the enlistment and activation of an amazingly complex sequence of events.
Case 6.1
6.1.3
Activation levels in one area of the cortex may influence activity levels in other areas of the cortex. Depression may result from a decreased activation of frontal cortical areas that result in a decreased activation of the motor cortex and association cortex of the frontal lobes. Further to this theory, a decrease in limbic activation may decrease the drive to move, which would also decrease the activation level in the motor cortical circuits.
References
Brooks V.B. The Neural Basis of Motor Control. Oxford: Oxford University Press, 1984.
Llinas R.R. Of dreaming and wakefulness. Neuroscience. 1991;44:521-535.
Martin J.P. The Basal Ganglia and Posture. London: Pitman Medical, 1967. pp. 1–152
Sherman S.M., Guillery R.W. Exploring the Thalamus. New York: Academic Press, 2001.