Chapter 33B Neuroimaging
Vascular Imaging: Computed Tomographic Angiography, Magnetic Resonance Angiography, and Ultrasound
Computed Tomographic Angiography
Methods
Helical CT scanner technology, providing uninterrupted volume data acquisition, can rapidly image the entire cerebral circulation from the neck to vertex of the head within minutes. Typical CT parameters use a slice (collimated) thickness of 1 to 3 mm with a pitch of 1 to 2, which represents the ratio of the table speed per rotation and the total collimation. Data are acquired as a bolus of iodinated contrast medium traverses the vessels of interest. For CTA of the carotid and vertebral arteries in the neck, the helical volume extends from the aortic arch to the skull base. Typical acquisition parameters are 7.5 images per rotation of the x-ray tube, 2.5-mm slice thickness, and a reconstruction interval (distance between the centers of two consecutively reconstructed images) of 1.25 mm. For CTA of the circle of Willis and proximal cerebral arteries, the data acquisition extends from the skull base to the vertex of the head. Typical acquisition parameters for this higher spatial resolution scan are 3.75 images per rotation, 1.25-mm slice thickness, and an interval of 0.5 mm. A volume of contrast ranging from 100 to 150 mL is injected into a peripheral vein at a rate of 2 to 3 mL/sec and followed by a saline flush of 20 to 50 mL. Adequate enhancement of the arteries in the neck or head is obtained approximately 15 to 20 seconds after injection of the contrast, although this may vary somewhat in each case. Image acquisition uses automated detection of bolus arrival and subsequent triggering of data acquisition. The resulting axial source images are typically post-processed for two-dimensional (2D) and three-dimensional (3D) visualization using one or more of several available techniques including multiplanar reformatting, thin-slab maximum-intensity projection (MIP), and 3D volume rendering. Recently introduced CT with 320 detector rows enables dynamic scanning, providing both high spatial and temporal resolution of the entire cerebrovasculature (4D CTA). The cervical vessels are imaged by acquisition of an additional spiral CT scan analogous to 64-detector row CT. Validated clinical applications of this advanced technique currently remain under investigation (Diekmann et al., 2010).
Limitations
Contrast-Induced Nephropathy
Careful consideration must be made for performing contrast-enhanced CT studies in patients with renal impairment. Exposure to all contrast agents may result in acute renal failure, called contrast-induced nephropathy (CIN), which is typically reversible but may potentially result in adverse outcomes. The incidence of renal injury appears to be associated with increased osmolality of contrast agents, which have been steadily declining with the newer generations of nonionic agents. Patients with a creatinine level above 1.5 gm/dL or estimated glomerular filtration rate below 60 mL/min/1.73m2 remain at a higher risk for developing CIN. Treatment for this condition relies on prevention of this disorder, and agents such as N-acetylcysteine and intravenous (IV) saline and/or sodium bicarbonate have been demonstrated to reduce the incidence of CIN. Avoidance of volume depletion and discontinuation of nonsteroidal antiinflammatory drugs, which may cause renal vasoconstriction, is recommended for patients prior to the procedure. Patients on hemodialysis are recommended to undergo dialysis as soon as possible afterwards to reduce contrast exposure (Asif and Epstein, 2004; Kim et al., 2010).
Metal Artifacts
Metallic implants such as clips, coils, and stents are generally safe for CT imaging, but it should be noted that they may lead to severe streaking artifacts, limiting evaluation. These artifacts occur because the density of the metal is beyond the normal range of the processing software, resulting in incomplete attenuation profiles. Several processing methods are available to reduce the artifact signal, and operator-dependent techniques such as gantry angulation adjustments and use of thin sections to reduce partial volume artifacts may help decrease this signal distortion. Generally, knowledge of the composition of metallic implants may help in determining the potential severity of artifacts on CT. Cobalt aneurysm clips produce much more artifact than titanium clips. For patients with stents, careful consideration must be made in evaluating stenosis, as these implants may lead to artificial lumen narrowing on CTA. The degree of artificial lumen narrowing decreases with increasing stent diameter. Lettau et al. evaluated patients with various types of stents and found that CTA may be superior to magnetic resonance angiography (MRA) at 1.5T for stainless steel and cobalt alloy carotid stents, whereas MRA at 3T may be superior for nitinol carotid stents (Lettau et al., 2009; van der Schaaf et al., 2006).
Applications
Extracranial Circulation
Carotid Artery Stenosis
In evaluating occlusive disease of the extracranial carotid artery, CTA complements conventional or digital subtraction angiography (DSA) and serves as an alternative to MRA (Fig. 33B.1). In the grading of carotid stenosis using the North American Symptomatic Carotid Endarterectomy Trial (NASCET) criteria, Randoux and colleagues (2001) found that the rate of agreement between 3D CTA and DSA was 95%. Relative to DSA (the reference standard), severe stenosis (70%-99%) was detected with a sensitivity and specificity of 100% and 100%, respectively, for CTA and 93% and 100%, respectively, for contrast-enhanced MRA (CE-MRA). In addition, CTA and CE-MRA were significantly correlated with DSA in depicting the length of the stenotic segment.
Other investigators have reported lower sensitivity (80%-89%) yet comparable specificity (96%-100%) for CTA in detecting severe stenosis (Binaghi et al., 2001; Magarelli et al., 1998). Those investigators found that time-of-flight (TOF) MRA had higher sensitivity (92%-93%) than CTA and similar specificity (98%-100%). Binaghi and colleagues also compared CTA with DSA and showed that the sensitivity was the same (89%), whereas specificity was higher for CTA (100%) than for DSA (81%). In 2006, Wardlaw et al., performed a meta-analysis on studies comparing CTA with DSA for the diagnosis of carotid artery stenosis. For detection of severe (70% to 99%) stenoses, CTA demonstrated a pooled sensitivity and specificity of 77% and 95%, respectively. Data for moderate (50%-69%) stenoses were determined to be sparse and unreliable (Wardlaw et al., 2006). An earlier systematic review found CTA to be a reliable method for detecting severe (70%-99%) stenoses, with a sensitivity and specificity of 85% and 93%, respectively. For detection of a complete occlusion, the sensitivity and specificity were 97% and 99%, respectively (Koelemay et al., 2004). Saba et al. evaluated the use of multidetector CTA and carotid ultrasound in comparison to surgical observation for evaluating ulceration, which is a severe complication of carotid plaques. CTA was found to be superior, with 93.75% sensitivity and 98.59% specificity compared to carotid ultrasound, which demonstrated 37.5% sensitivity and 91.5% specificity (Saba et al., 2007).
Fibromuscular dysplasia (FMD), which often involves a unique pattern of stenoses in the cervical vessels, may be detected by CTA, although no large studies have evaluated the sensitivity and specificity for detection. This disorder, which characteristically demonstrates a string-of-beads pattern of vascular irregularity on angiography, has been reliably demonstrated on carotid artery evaluations from case reports. This may potentially reduce the need for more invasive angiographic imaging in the future, although further studies in this area are required (de Monye et al., 2007).
Carotid and Vertebral Dissection
Dissections of the cervicocephalic arteries, including the carotid and vertebral arteries, account for up to 20% of ischemic strokes in young adults (Leys et al., 1995). CTA findings include demonstration of a narrowed eccentric arterial lumen in the presence of a thickened vessel wall, with occasional detection of a dissecting aneurysm. In subacute and chronic dissection, CTA has been shown to detect a reduction in the thickness of the arterial wall, recanalization of the arterial lumen, and reduction in size or resolution of dissecting aneurysm. CTA or MRA is superior to DSA in depicting the distal portions of the cervical internal carotid artery (ICA), a common site of dissection. CTA is likely superior to MRI alone in evaluating aneurysms at these sites because MRI findings are often complicated by the presence of flow-related artifacts. CTA depiction of dissections at the level of the skull base may be complicated in some cases because of to beam hardening and other artifacts that obscure dissection findings, including similarities in the densities of the temporal and sphenoid bones with the dissected ICA.
A retrospective review compared combined multidetector CT/CTA with MRI/MRA among 18 patients with 25 dissected vessels in both anterior and posterior circulations. CT/CTA was preferred for diagnosis in 13 vessels, whereas MRI/MRA was preferred in 1 vessel, and the techniques were deemed equal in the remaining 11 vessels. It should be noted that such combinations of noninvasive angiographic study with other CT or MRI components is common and therefore does not reflect the role of CTA or MRA alone. A significant preference for CT/CTA was noted for vertebral artery dissections but not for ICA dissections (Vertinsky et al., 2008). When compared with DSA, a small retrospective review of patients found that multidetector CTA had a sensitivity of 100% and specificity of 95% for detecting vertebral artery dissections (Pugliese et al., 2007). A small study evaluated multislice CTA with cervical axial T1-weighted MRI and MRA among seven patients with carotid artery dissection. The combination of MRI and MRA identified dissection in five of the seven patients that were identified by CTA. Additionally, a dissecting aneurysm was identified by CTA that was missed by MRI and MRA. These findings suggest that CTA may be at the very least a complementary study to provide additional information (Elijovich et al., 2006).
Intracranial Circulation
Acute Ischemic Stroke
Computed tomography angiography is a reliable alternative to MRA in evaluating arterial occlusive disease near the circle of Willis in patients with symptoms of acute stroke (Knauth et al., 1997; Shrier et al., 1997) (Fig. 33B.2). CTA shows clinically relevant occlusions of major cerebral arteries and enhancement caused by collateral flow distal to the site of occlusion. CTA may be superior to transcranial Doppler (TCD) ultrasound in diagnosing atherothrombotic MCA disease in Asian patients presenting with middle cerebral artery (MCA) stroke (Suwanwela et al., 2002). CTA detected MCA stenosis measuring more than 50% in twice as many patients as TCD. The difference resulted primarily from improved detection by CTA of distal M1 and M2 stenosis. Because half of the patients studied by Suwanwela and colleagues had distal M1 and M2 disease, the authors concluded that TCD should not be used to screen for MCA stenosis.
In the detection of intracranial steno-occlusive disease, Hirai and colleagues (2002) have shown that combined CTA and MRA provide substantially higher sensitivity, specificity, and accuracy than MRA alone. Review of the CTA depiction of vessels in conjunction with the 3D time-of-flight (TOF) MRA reduced the frequency of overestimation of stenosis when compared with MRA alone. In the identification of 50% or greater stenosis, the sensitivity, specificity, and accuracy for the combined CTA and MRA evaluation were 100%, 99%, and 99%, respectively, and the values for 3D TOF-MRA alone were 92%, 91%, and 91%, respectively. The grading of stenosis by the combined approach agreed with DSA grading in 98% of cases. In a retrospective review of their cases, the authors found that CTA did not always correctly delineate arterial lumina with circumferential calcification and the cavernous portion of the ICA. Nguyen et al. evaluated 475 vessel segments in 41 patients who received both CTA and DSA studies and found that for arterial occlusions, CTA had demonstrated 100% sensitivity and specificity. For detection of 50% or more stenosis, CTA had 97.1% sensitivity and 99.5% specificity. There was no difference observed in CTA accuracy for vessel segments in the anterior versus posterior circulation (Nguyen-Huynh et al., 2008). In a recent review of published studies, Latchaw et al. found that in comparison to conventional angiography, CTA demonstrated sensitivities ranging from 92% to 100% and specificities of 82% to 100% for the detection of intracranial vessel occlusion. The sensitivities for detection of intracranial stenoses range from 78% to 100%, with specificities of 82% to 100% (Latchaw et al., 2009). CTA is considered to be superior to TCD in detecting intracranial stenoses and occlusions, with a high false-negative rate noted for Doppler ultrasound. Studies also suggest that CTA has a higher sensitivity when directly compared with 3D TOF-MRA. Bash et al. found that CTA had a sensitivity of 98% while MRA had a sensitivity of 70% for detection of intracranial stenosis. The sensitivity for detecting occlusions on CTA was 100% and only 87% on MRA. Additionally, CTA was noted to superior to both MRA and DSA in detecting posterior circulation stenoses when slow or balanced flow states were present, possibly owing to longer scan time, which allows for more contrast to pass through a critical stenosis. Although previous studies noted decreased accuracy with the presence of atheromatous calcifications, the sensitivity and specificity of CTA for stenosis quantification were not compromised by this when appropriate window and level adjustments were made to account for the blooming artifacts that are frequently associated with heavy calcifications (Bash et al., 2005).
Computed tomography angiography source images (CTA-SI) may be used to provide an estimate of perfusion by taking advantage of the contrast enhancement in the brain vasculature that occurs during a CTA, possibly making it unnecessary to perform a separate CT perfusion study with a second contrast bolus. In normal perfused tissue, contrast dye fills the brain microvasculature and appears as increased signal intensity on the CTA-SI. In ischemic brain regions with poor collateral flow, contrast does not readily fill the brain microvasculature. Thus, these regions demonstrate low attenuation (Schramm et al., 2002). The hypoattenuation seen on CTA-SI correlate with abnormality on diffusion-weighted MRI (DWI) and have been found to be more sensitive than noncontrast CT scans for the detection of early brain infarction (Camargo et al., 2007). The sensitivity of CTA-SI and DWI when directly compared has been found to be similar in detecting ischemic regions, but DWI is better at demonstrating smaller infarcts and those in the brainstem and posterior fossa. Such findings may be useful for patients with symptoms of acute infarction who cannot undergo MRI (Latchaw et al., 2009).
In addition to perfusion status, CTA imaging may potentially be used for prognostication in patients undergoing acute stroke intervention. The 10-point Clot Burden Score (CBS) was devised as a semiquantitative analysis of CTA to help determine prognosis in acute stroke (Fig. 33B.3). The CBS subtracts 1 or 2 points each for absent contrast opacification on CTA in the infraclinoid internal carotid artery (ICA) (1), supraclinoid ICA (2), proximal M1 segment (2), distal M1 segment (2), M2 branches (1 each), and A1 segment (1). The CBS applies only to the symptomatic hemisphere. A CBS below 10 was associated with reduced odds of independent functional outcome (odds ratio (OR) 0.09 for a CBS of 5 or less; OR 0.22 for CBS 6 to 7; OR 0.48 for CBS 8 to 9; all versus CBS 10). The quantification of intracranial thrombus extent with the CBS predicts functional outcome, final infarct size, and parenchymal hematoma risk acutely. This scoring system requires external validation and could be useful for patient stratification in stroke trials (Puetz et al., 2008).
The Alberta Stroke Program Early CT Score (ASPECTS) is a 10-point analysis of topographic CT scan score used in patients with MCA stroke (Fig. 33B.4 and Box 33B.1). Segmental assessment of MCA territory is made, and 1 point is removed from the initial score of 10 if there is evidence of infarction in the following regions: putamen, internal capsule, insular cortex, anterior MCA cortex, MCA cortex lateral to insular ribbon, posterior MCA cortex, anterior MCA territory immediately superior to M1, lateral MCA territory immediately superior to M2 and posterior MCA territory immediately superior to M3. An ASPECTS score of 7 or less predicts worse functional outcome at 3 months as well as symptomatic hemorrhage. Puetz et al. sought to determine whether the ASPECTS scoring system could be applied to CTA-SI and combined with the CBS system for improved prognostication. A 10-point ASPECTS score based on CTA-SI and the 10-point CBS were combined to form a 20-point score for patients presenting acutely with stroke who received thrombolysis treatment. For patients with a combined score of 10 or less, only 4% were functionally independent, and mortality was 50%. In contrast, 57% of patients with scores of 10 or greater were functionally independent, and mortality was 10%. Additionally, parenchymal hematoma rates were 30% versus 8%, respectively (Puetz et al., 2010). A similar semiquantitative scoring system for CTA-SI was devised for patients presenting with acute basilar artery occlusion and termed the posterior circulation (pc)-ASPECTS (Fig. 33B.5). This 10-point scoring system subtracts 1 or 2 points each for areas of hypoattenuation in the left or right thalamus, cerebellum, or posterior cerebral artery (PCA) territory, respectively (1 point), or any part of the midbrain or pons (2 points). Median follow-up pc-ASPECTS was lower in patients with a CTA-SI pc-ASPECTS less than 8 compared with patients with a CTA-SI pc-ASPECTS of 8 or higher, respectively. Hemorrhagic transformation rates were 27.3% versus 9.5%, respectively, for patients who received thrombolysis. The results indicate that such analysis can predict a larger final infarct extent in patients with basilar artery occlusion. Larger prospective trials are required for validation, but the systematic acute evaluation of CTA along with CTA-SI may potentially be used to help guide future stroke treatments (Puetz et al., 2009).
Box 33B.1 Alberta Stroke Program Early CT Score*
Intracerebral Hemorrhage
Patients presenting acutely with intracerebral hemorrhage (ICH) within the first few hours of symptom onset are known to be at increased risk for hematoma expansion. However, only a fraction of such patients arrive at a hospital within this time frame, so alternative means of identifying potential hemorrhage expansion have been sought because it is an important predictor of 30-day mortality. One such prognostic marker has been identified on CTA: the spot sign, defined as a tiny, enhancing foci seen within hematomas, with or without clear contrast extravasation. A prospective study by Wada et al. of 39 consecutive patients with spontaneous ICH within 3 hours of symptom onset identified this sign in 33% of cases. Sensitivity was found to be 91%, and specificity was 89% for predicting hematoma expansion. In patients with the spot sign, mean volume change was greater, extravasation was more common, and median hospital stay was longer (Wada et al., 2007).
A larger retrospective analysis determined that the presence of three or more spot signs, a maximum axial dimension of 5 mm or greater, and maximum attenuation of 180 Hounsfield units or more were independent predictors of significant hematoma expansion (Delgado et al., 2009). In another study, Delgado et al. noted that the presence of any spot sign increased the risk of in-hospital mortality (OR, 4.0) and poor outcome among survivors at 3-month follow-up (OR, 2.5). This was determined to be an independent predictor of both measures. The spot sign currently requires further validation but remains a promising use of CTA in guiding acute ICH management (Delgado et al., 2010).
Brain Death
The absence of cerebral circulation is an important confirmatory test for brain death, and CTA is emerging as an important alternative means of testing. A novel 4-point score was devised, with points subtracted based on the lack of opacification of the cortical segments of the MCAs and internal cerebral veins. Frampas et al. used this system to prospectively evaluate 105 patients who were clinically brain dead and found a sensitivity of 85.7% and specificity of 100%. This appears to be a possible alternative means of detecting cerebral circulatory arrest, and given that it is a fast and noninvasive technique, it may become a useful confirmatory test (Frampas et al., 2009; Escudero et al., 2009).
Cerebral Venous Thrombosis
The diagnosis of cerebral venous thrombosis (CVT) was previously often made with conventional angiography and more recently by MRI techniques. Magnetic resonance venography (MRV) is commonly considered the most sensitive noninvasive test in diagnosing CVT. However, given the prolonged imaging time and often limited availability, CTA has been studied as a potential alternate means of detecting CVT. Spiral CT with acquisition during peak venous enhancement has been implemented with single-section systems but remains limited in spatial and temporal resolution. One study directly comparing CTV with MRV demonstrated a sensitivity and a specificity of 75% to 100%, depending on the sinus or venous structure involved (Khandelwal et al., 2006). Multidetector-row CTA (MDCTA) offers higher spatial and temporal resolution, which allows for high-quality multiplanar and 3D reformatting. Two recent small studies found 100% specificity and sensitivity with MDCTA when compared to MRV. The venous sinuses could be identified in 99.2% and the cerebral veins in 87.6% of cases. MDCTA may be equivalent to MRV in visualizing cerebral sinuses, but further studies are needed to evaluate the diagnostic potential of MDCTA in specific types of CVT such as cortical venous thrombosis, thrombosis of the cavernous sinus, and thrombosis of the deep cerebral veins. The advantages of MDCTA include the short exam duration and the possible simultaneous visualization of the cerebral arterial and venous systems with a single bolus of contrast. MDCTA visualizes thrombus via contrast-filling defects and remains less prone to flow artifacts. A potential problem with this technique lies in the fact that in the chronic state of a CVT, older organized thrombus may show enhancement after contrast administration and may not produce a filling defect, leading to a false-negative result. The addition of a noncontrast CT with the MDCTA is sometimes used to remove another potential to obtain false-negative results from the presence of a spontaneously hyperattenuated clot that could be mistaken for an enhanced sinus. This phenomenon is known as the cord sign and may be seen in 25% to 56% of acute CVT cases (Gaikwad et al., 2008; Linn et al., 2007).
Cerebral Aneurysms
Although 3D TOF-MRA and dynamic 3D CE-MRA have similar sensitivity and specificity to CTA for detection of intracerebral aneurysms at least 5 mm in diameter, they have lower sensitivity for aneurysms smaller than 5 mm (Villablanca et al., 2002; White et al., 2001) (Figs. 33B.6 and 33B.7). The results of the International Study of Unruptured Intracranial Aneurysms (ISUIA) (Wiebers et al., 2003) suggest that MRA, despite its lower sensitivity for smaller aneurysms, would not significantly change management, because incidental aneurysms smaller than 10 mm should not be treated (exceptions may be made for individuals with daughter aneurysm formation, a family history of subarachnoid hemorrhage, and young patients). However, in general, the accuracy of CTA is felt to be at least equal if not superior to that of MRA in most circumstances, and in some cases, its overall accuracy approaches that of DSA (Latchaw et al., 2009).
Using optimized helical CTA acquisition and post-processing protocols that included 3D volume-rendered images, 3D thick-slab and grayscale 2D single-section images, and thick-slab multiplanar reformatted 2D images, Villablanca and colleagues (2002) reported a sensitivity for the detection of small aneurysms (<5 mm diameter) of 98% to 100%, compared with 95% for DSA. The specificity of both CTA and DSA was 100%. CTA image analysis times ranged from 6 to 36 minutes (mean, 16 minutes). The smallest aneurysm detected was 1.9 × 1.6 × 1.3 mm3, and 48% of aneurysms were detected in the presence of subarachnoid hemorrhage. The sensitivity of CTA exceeded that of DSA, primarily because the optimal projection necessary to visualize some aneurysms could be displayed on the post-processed CTA images but was not or could not be displayed by the 2D DSA. Other disadvantages of DSA that have been noted by investigators include superimposition of normal vessels, obscuring a small aneurysm, and the lack of an internal image scale for estimating the aneurysm sac and neck dimensions. Villablanca et al. (2002) showed that CTA can provide quantitative information such as dome-to-neck ratios and aneurysm characterization such as the presence of mural thrombi or calcium, branching pattern at the neck, and the incorporation of arterial segments in the aneurysm. The 3D images in particular provided a surgically useful display of the aneurysm sac in relation to skull base structures (see Fig. 33B.7). The authors concluded that clinically relevant aneurysms can be detected by CTA using published protocols, routine scanners, and commercially available image-processing workstations. Furthermore, CTA can be a reliable source of information for treatment planning.
Cerebral veins show much more anatomical variation than arteries. The presence of an unexpected vein or the lack of collateral drainage from a region drained by a vein that may need to be sacrificed during surgery can alter the approach to resection of an aneurysm. Kaminogo and colleagues (2002) used 3D CTA to demonstrate the venous anatomy accurately. They showed the usefulness of this information in selecting a therapeutic procedure (surgery versus endovascular coiling) and in planning the approach for surgical treatment.
Recent data indicate that CTA is a safe and accurate imaging technique for evaluating most extracranial and intracranial vessels to detect the presence of stenoses or occlusions, as well as for the detection of intracranial aneurysms. The accuracy of CTA appears equal to or superior to MRA imaging in most circumstances, and its accuracy sometimes approaches that of DSA. The development of new advanced CT scanners with more detectors may further enhance the accuracy of this technique (Latchaw et al., 2009).
Magnetic Resonance Angiography
Methods
Contrast-enhanced MRA (CE-MRA) uses scan parameters that are typical of 3D TOF-MRA but uses gadolinium to overcome the problem of saturation of the slow-flowing blood in structures that lie within the 3D slab (Fig. 33B.8). The scan time per 3D volume is on the order of 5 to 10 minutes, and data are acquired in the first 10 to 15 minutes after the bolus infusion of a gadolinium contrast agent (0.1-0.2 mmol/kg). Presaturation bands usually are ineffective at suppressing the downstream signal from vessels when gadolinium is present. In 3D CE-MRA (called fast, dynamic, or time-resolved CE-MRA), the total scan time per 3D volume (usually about 30-50 partitions) is reduced to 5 to 50 seconds (Fain et al., 2001; Melhem et al., 1999; Turski et al., 2001). Data are acquired as the bolus of the gadolinium contrast agent (0.2-0.3 mmol/kg and 2-3 mL/sec infusion rate) passes through the vessels of interest, taking advantage of the marked increase in intravascular signal (first-pass method). Vessel signal is determined primarily by concentration of injected contrast, analogous to conventional angiography. Because 3D CE-MRA entails more rapid data acquisition, and hence higher temporal resolution, than TOF-MRA, spatial resolution may be reduced. The most common approaches to synchronizing the 3D data acquisition with the arrival of the gadolinium bolus in the arteries are measurement of the bolus arrival time for each patient using a small (2 mL) test dose of contrast followed by a separate synchronized manual 3D acquisition (Foo et al., 1997) by the scanner operator (Fain et al., 2001; Foo et al., 1997). Another method rapidly and repeatedly acquires 3D volumes (<10 sec per volume) in the neck, beginning at the time of contrast bolus injection to ensure that at least one 3D volume showing only arteries will be acquired (Turski et al., 2001). Subtraction of preinjection source images from arterial phase images, termed digital subtraction MRA, is sometimes used to increase vessel-to-background contrast.
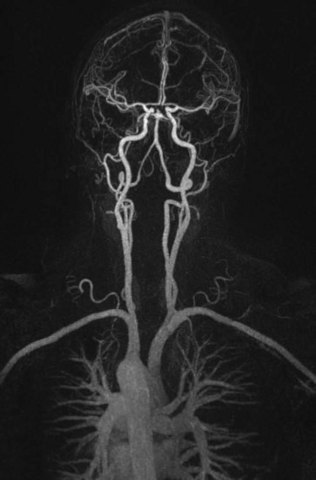
Fig. 33B.8 3T contrast-enhanced magnetic resonance angiography (CE-MRA) of the cerebrovascular system.
The advent and increasing availability of MRI scanners with 3.0 tesla (T) or even higher field strengths (up to 7T) in selected centers may also be used to improve MRA by capitalizing on higher signal-to-noise ratios and parallel imaging (Nael et al., 2006; Pruessmann et al., 1999). Parallel imaging at 3T or greater can be used to improve spatial resolution, shorten scan time, reduce artifacts, and increase anatomical coverage in first-pass CE-MRA. Recent investigation with 7T TOF-MRA demonstrated that such ultrahigh field strength allows vivid depiction of the large vessels of the circle of Willis with significantly more first- and second-order branches and can even distinguish diseased diminutive vessels in hypertensive patients (Hendrikse et al.; 2008; Kang et al., 2009; Kang et al., 2010). The 4D time-resolved MRA (4D MRA) is a novel contrast-enhanced vascular imaging method under investigation that uses novel processing techniques to achieve subsecond temporal resolution while maintaining high spatial resolution. Dynamic MRA scans may be obtained up to 60 times faster and with higher spatial resolution at 3T. The resulting images may attain the diagnostic performance of conventional DSA, allowing for better characterization of various vascular lesions (Hope et al., 2010; Parmar et al., 2009; Willinek et al., 2008).
Limitations
Nephrogenic Systemic Fibrosis
Clinicians should be aware that a rare but serious complication of contrast-enhanced MRI studies includes the development of nephrogenic systemic fibrosis (NSF).This condition is characterized by widespread thickening and hardening of the skin, with the potential for rapid systemic progression and immobility over several weeks. Although the precise mechanism remains unclear, NSF was first noted in 1997 and occurs exclusively in patients with renal failure. The majority of reported cases involved exposure to a gadolinium chelate within 2 to 3 months prior to disease onset in dialysis-dependent patients. A large percentage of cases has been specifically associated with gadodiamide (Omniscan), with a possible dose-dependent effect, although it should be noted that cases have been reported with all gadolinium agents. Careful consideration must be made for gadolinium administration in patients with renal impairment (glomerular filtration rate <30 mL/min/1.73m2), especially those on dialysis. The risks and benefits should be carefully discussed prior to any contrast-enhanced procedures, and the dose of gadolinium should be minimized. Additionally, for patients on hemodialysis, prophylactic dialysis is generally recommended as soon as possible, ideally within 3 hours after contrast administration (Kuo et al., 2007).
Metal Implant Contraindications
Limitations due to the presence of metallic materials (clips, stents, coils) remains a common concern in patients undergoing vascular imaging. Aneurysm clips made from martensitic stainless steels remain a contraindication for MRI procedures, because excessive magnetic forces may displace these implants and cause serious injury. However, most clips are now made of metals that are non-ferromagnetic, and all patients with any metallic implants require screening to determine whether they are safe to undergo an MRI study. For the majority of coils and stents that have been tested, it is unlikely that these implants would become moved or displaced as a result of exposure to MRI systems operating at 1.5T or even 3T. Additionally, it is often unnecessary to wait an extended period of time after a procedure to perform an MRI study in a patient with an implant made of non-ferromagnetic material unless there are concerns associated with MRI-related heating. Dental materials including wires and prostheses do not appear to pose a risk, although they may result in artifact on MRI. Artifacts from metal may have varied appearances on MRI related to the type or configuration of the piece of metal. Artifact sizes may increase at 3T compared to 1.5T, depending on the implant type and composition, but these distortions may be substantially reduced by optimizing imaging parameters. Patients who are deemed unsafe for MRI upon screening may be considered for CT evaluation (Olsrud et al., 2005; Shellock, 2002).
Applications
Extracranial Carotid and Vertebral Circulation
Time-of-Flight Mra
Earlier clinical reports outlined the advantages and pitfalls of TOF-MRA for imaging of the extracranial circulation and estimation of degree stenosis at the carotid bifurcation (Norris and Rothwell, 2001). The degree of stenosis tends to be overestimated by the traditional 2D TOF-MRA method. A corollary of this observation is that a 2D TOF study with normal or near-normal findings effectively excludes the possibility of severe (70% to 99%) stenosis. The most accurate results are obtained when short TE and small voxel size are used. Second, a consensus estimate of stenosis derived from a combination of the 2D and 3D TOF methods results in greater specificity than 2D TOF alone. This improvement results primarily from the inclusion of the 3D TOF method, in which stenosis is less likely to be overestimated, particularly if original (Fig. 33B.9) or reformatted source images are evaluated rather than the MIP images. In the combined TOF approach, the 2D TOF method is used primarily to distinguish slow flow from occlusion and, in general, the combined TOF approach is considered superior to DSA in differentiating high-grade stenosis from occlusion. A flow gap, which is a segmental dropout of signal from the carotid (or other vessels) caused by intravoxel phase dispersion or saturation, is often taken as a sign of stenosis measuring 70% or more. This association should be viewed with caution, however, because in one published series of patients, flow gap was observed with the 2D TOF technique at sites of 50% to 60% stenosis as determined by DSA.
Third, in detecting stenosis appropriate for carotid endarterectomy, TOF-MRA is less sensitive than DSA (75% MRA, 87% DSA) but more specific (88% MRA, 46% DSA); however, when stenosis estimates by TOF-MRA and DSA are concordant and are then taken together, the combined MRA and DSA examination is more sensitive (96%) and specific (85%) than either study alone. Furthermore, when patients are classified as to whether carotid endarterectomy is indicated by the noninvasive examination and then judged against the results of DSA, the misclassification rate for the concordant MRA and DSA results is much lower than that of either test alone (MRA and DSA 7.9%, MRA 18%, DSA 28%) (Johnston and Goldstein, 2001). Therefore, surgical decisions are more likely to be correct when based on concordant TOF-MRA and DSA results.
Three-Dimensional Contrast-Enhanced Mra
Compared with 2D and 3D TOF-MRA, 3D CE-MRA delineates carotid arterial stenosis better (Willig et al., 1998) (Fig. 33B.10). Surface morphology (e.g., ulcerated plaque) and nearly occluded vessels (e.g., “string sign”) are more easily identified, and arterial occlusions are more confidently identified. Severe carotid bifurcation stenosis may be detected by 3D CE-MRA with high sensitivity (93%-100%) and specificity (88%-96%) (Huston et al., 1998; Johnson et al., 2000; Lenhart et al., 2002; Remonda et al., 2002; Wutke et al., 2002) using DSA as the standard method of diagnosis. TOF techniques yield similar results to 3D CE-MRA for mild to moderate stenosis, thus obviating time spent on CE-MRA setup and processing. Advantages of 3D CE-MRA include greater anatomical coverage (Fig. 33B.11). For high-grade stenosis, which can cause intravascular flow gaps on TOF MIP images, the addition of CE-MRA to the imaging protocol provides sensitivity and specificity equivalent to CTA in determining the severity of stenosis (relative to DSA as the reference standard).
TOF-MRA and CE-MRA have been found to achieve high accuracy for the detection of high-grade ICA stenoses and occlusions, with CE-MRA having slight benefit over TOF-MRA. A systematic review and meta-analysis of 37 TOF-MRA studies and 21 CE-MRA studies was performed by Debrey et al. and found that for the detection of high-grade (≥70-99%) ICA stenoses, TOF-MRA had an overall sensitivity of 91.2%, with a specificity of 88.3%. The sensitivity of CE-MRA was higher at 94.6%, with a specificity of 91.9%. For the detection of complete ICA occlusions, the sensitivity of TOF-MRA was 94.5%, and the specificity was 99.3%, while CE-MRA demonstrated a sensitivity of 99.4% and specificity of 99.6%. However, for moderately severe ICA stenoses (50%-69%), sensitivity was found to be poor in TOF-MRA and only fair in CE-MRA studies. TOF-MRA had a sensitivity of only 37.9% and a specificity of 92.1%, while CE-MRA had a sensitivity of 65.9% with a specificity of 93.5% (Debrey et al., 2008).
The ability to detect an FMD pattern of stenoses by MRA in the carotid vessels remains uncertain. This disorder may not be as well delineated on TOF-MRA owing to limited resolution, although no large comparative studies with CE-MRA have been performed. In one series evaluating FMD in the renal arteries, Willoteaux found the sensitivity and specificity of CE-MRA to be 97% and 93%, respectively. These findings suggest that using CE-MRA to identify FMD in the cervical vessels may be possible, although further studies are required (Willoteaux et al., 2006).
Atherosclerotic narrowing of the vertebral artery commonly involves the origin or distal intracranial portion. For TOF-MRA evaluation of posterior-circulation cerebrovascular disease, the vertebral origins usually are not evaluated for the same reasons the common carotid origins are not evaluated. Typically, a 3D TOF study covering the vertebrobasilar system from the C2 level to the tip of the basilar artery is done (Fig. 33B.12). However, sequential 2D TOF-MRA of the neck is useful in determining whether proximal occlusion is present and in demonstrating flow direction in the vertebral arteries in patients with suspected subclavian steal. A 2D TOF study obtained with no presaturation band shows flow enhancement in both vertebral arteries, whereas a study obtained with a superiorly located walking presaturation band shows flow only in the vertebral artery with normal anterograde flow. The 3D CE-MRA techniques can display both the origins and distal intracranial portions of the vertebral arteries in a single acquisition and are particularly useful in evaluating vertebral artery segments with partial or complete signal loss caused by slow flow and in-plane saturation effects. The accuracy of 3D CE-MRA measurements of stenosis at the vertebral artery origin has yet to be reported, although the accuracy is unlikely to equal that of carotid bifurcation measurements because of the smaller size of the vertebral origins (Kollias et al., 1999). Nevertheless, an analysis of the elliptical centric encoding technique predicts that it can achieve an isotropic spatial resolution of 1 mm (before zero filling) in a field of view typically used for bilateral carotid and vertebral imaging (Fain et al., 1999). Stenosis or occlusion of the subclavian artery is now routinely evaluated with 3D CE-MRA.
In patients with carotid or vertebral artery dissection, MRA is complemented by MRI with fat-saturation sequences that aid in detection and characterization of dissecting hematoma, associated dissecting aneurysm, and the length and caliber of the residual patent lumen, especially at the skull base (Fig. 33B.13). Subacute hyperintense thrombus is better seen if fat suppression is implemented on thin T1-weighted images to eliminate the high signal intensity from perivascular adipose tissue. When the evaluation includes a 2D or 3D TOF combined study or 3D CE-MRA (Kollias et al., 1999), the detection of stenosis, pseudoaneurysm, or occlusion is improved. Also, the presence of a thrombosed false lumen is more convincingly demonstrated when spin-echo images are supplemented with phase-contrast or TOF images showing absence of flow in the false lumen. The improved recognition of these features afforded by MRA impacts on treatment decisions, such as deployment of stents and other endovascular devices. Serial MRA examinations are required to evaluate for recanalization of the vessel following the dissection (Fig. 33B.14) and also to evaluate for dissecting aneurysms that may occasionally develop as the hematoma resolves.
The MRA assessment of vascular stenosis after placement of a metallic stent is limited by turbulence and susceptibility effects. Stent geometry, the relative orientation of the magnetic field, and alloy composition contribute to signal intensity alterations within the stent lumen (Lenhart et al., 2000) (Fig. 33B.15). CTA is also limited in determining stenosis of the lumen of a stented vessel. The limitation is primarily due to streak artifacts from the stent, which obscure the residual lumen. Also, widened window settings and volume averaging often result in overestimation of stenosis on CTA (see Fig. 33B.15).
Intracranial Circulation
The accuracy of TOF-MRA in detecting stenosis or occlusion of the proximal intracranial arteries, compared with that of DSA, has been studied by several investigators (Furst et al., 1996; Stock et al., 1995). Initially, accuracy was limited by technical shortcomings such as long TE, lower spatial resolution, and single thick-slab acquisition. These resulted in a decrease in vascular signal due to intravoxel phase dispersion, susceptibility effects, and saturation effects. Signal loss was typically evident in the petrous, cavernous, and supraclinoid segments of the ICA and in the proximal M1 segment of the MCA. Second- and third-order branches of the cerebral arteries were poorly shown. Later studies reported that normal vessels and completely occluded vessels could be graded correctly when compared with DSA results, but stenotic segments were correctly graded (as either < or > 50% narrowing) only about 60% of the time. Subsequently, technical improvements in the 3D TOF method (variable flip angle, magnetization transfer suppression, multiple thin-slab acquisitions, and higher spatial resolution [512 matrix or greater]) improved the accuracy of stenosis grading, with investigators reporting that 80% of stenoses greater than 70% and 88% of stenoses less than 70% are quantified correctly with MRA.
The current approach to evaluating the intracranial arteries uses a multi-slab 3D TOF acquisition that covers the head from the foramen magnum extending superiorly to the areas of interest. Each slab has a transaxial orientation and may or may not have a superiorly located presaturation band. The axial source images and the reprojected MIP images (Fig. 33B.16) are reviewed in conjunction with other MR images. In the setting of acute infarction with the potential for thrombolytic treatment, protocols often include a rapidly acquired 2D PC-MR study of the circle of Willis instead of the more time-consuming 3D TOF study. Other clinical settings in which MRA reportedly complements routine MRI include sickle cell disease, moyamoya syndrome, hemifacial spasm, and trigeminal neuralgia. Flow dynamics (magnitude and direction) in the circle of Willis are more easily determined with the PC method, especially when vessel diameters are 1 mm or more.
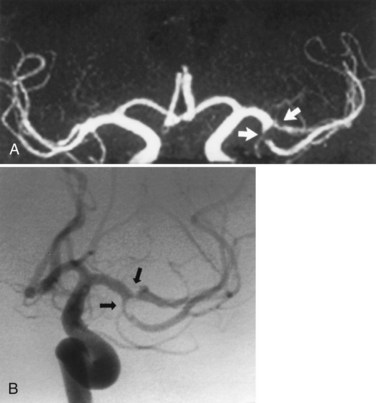
Fig. 33B.16 Proximal middle cerebral artery (MCA) stenosis (same patient as in Fig. 33B.4). A, Coronal projection magnetic resonance angiogram was produced from the axial source images shown in Fig. 33B.4. Coronal view shows better than the axial view (Fig. 33B.4, C) that there is stenosis (arrows) involving both M2 branches of the MCA. B, Catheter angiography confirms the presence of both stenoses (arrows).
The simplest type of 3D CE-MRA technique uses scan parameters typical of 3D TOF-MRA acquisitions with scan times on the order of 5 to 10 minutes per 3D volume. Under these steady-state conditions, visibility of the small intracranial arteries is greater after IV gadolinium administration; however, the intracranial veins also show a much greater increase in visibility. Consequently, the MIP images become cluttered with veins, resulting in greater difficulty in identifying and delineating specific arteries. With dynamic 3D CE-MRA, as used for extracranial carotid imaging, temporal resolution is improved, and visibility of arteries is greater than that of veins. Some investigators have suggested the use of region-of-interest MIP postprocessing to further exclude veins from intracranial artery displays. Despite the limits placed on spatial resolution by the dynamic 3D CE-MRA technique, Parker and colleagues (1998) have shown that in theory, imaging with a TR of 7 to 10 msec (e.g., scan time approximately 1 minute per 3D volume) and a T1 relaxation time of 25 to 50 msec for flowing blood containing gadolinium (first-pass arterial concentration of approximately 5-10 mM) can produce images of the intracranial arteries (≈0.5 mm diameter) with vascular contrast comparable to that produced by the steady-state 3D CE-MRA technique. Dynamic 3D CE-MRA may play a prominent future role in evaluating intracranial arterial steno-occlusive disease, but the accuracy, reproducibility, and reliability of CE-MRA measurements compared with those of DSA and TOF-MRA warrant further delineation.
Subclavian Steal Syndrome
Subclavian steal syndrome describes the reversal of normal direction of flow in the vertebral artery ipsilateral to a severe stenosis or occlusion occurring between the aortic arch and vertebral artery origin. DSA remains the standard in visualizing disease in the great vessels, along with abnormal retrograde filling of the affected vertebral artery. Given the invasive nature of DSA, Doppler sonography is often used, but this study may be limited by lack of visualization of the relevant pathology in the subclavian artery. Therefore, MRA offers a reliable comprehensive means to test patients with suspected subclavian steal syndrome. PC-MRA methods encode direction of flow and can accurately depict subclavian stenosis along with reversal of flow in the vertebral artery. Although TOF-MRA does not possess true flow-encoded information, flow direction can be deduced with suppression of flow from a single direction by a saturation pulse that allows for selective arterial or venous MRI, with reversal of flow presenting as a flow void. This finding may also be seen with severe stenosis or occlusion but may be distinguished by anatomical imaging of vessel patency, such as with 3D CE-MRA. A 3D CE-MRA has a potential disadvantage in the lone evaluation of subclavian steal syndrome, because it does not possess inherent flow-encoded information. However, the low-resolution 2D TOF localizer acquisition that is often performed beforehand has been shown to provide the same information as a formal TOF-MRA sequence (Sheehy et al., 2005).
Acute Ischemic Stroke
As advances are made in rapid MRI techniques, a novel application for MRA has been the use of this technique to potentially guide acute stroke intervention. In a study by Marks et al., patients with isolated MCA occlusion and early recanalization at TOF-MRA demonstrated more favorable clinical response than those with tandem ICA/MCA occlusion and early recanalization, among those treated with IV tissue plasminogen activator (tPA) 3 to 6 hours after symptom onset (Marks et al., 2008). The correlation of early angiographic findings with clinical outcomes in stroke has prompted further investigation into utilizing MRA as predictive instrument. The Boston Acute Stroke Imaging Scale (BASIS) was devised as a simple classification instrument to help predict outcomes in acute stroke. In this algorithm, patients are classified as having major stroke if they either demonstrate a proximal cerebral artery occlusion (distal intracranial ICA, proximal [M1 or M2] MCA, and/or basilar artery) or if large parenchymal acute ischemia is found on diffusion MRI or early CT imaging in the event no proximal cerebral artery occlusion is identified. All other circumstances are classified as a minor stroke by imaging. Using this classification, Torres-Mozqueda et al. found that a total of 71.4% of major stroke survivors and 15.4% of minor stroke survivors were discharged to a rehabilitation facility, whereas 14.3% and 79.2%, respectively, were discharged to home. The mean length of hospitalization was 12.3 days for major stroke and 3.3 days for minor stroke. Prospective validation of the BASIS classification instrument, along with future investigations examining dynamic MRA changes, remain promising potential uses of MRA for guidance of stroke treatment (Torres-Mozqueda et al., 2008).
Cerebral Aneurysms
Three-dimensional TOF-MRA is now readily accepted as a noninvasive screening tool for familial aneurysmal disease. It has also been used as an alternative to DSA for the surgical management of patients with ruptured aneurysms. A review of the relevant literature for 1988 to 1998 found that TOF-MRA (and CTA) depicted aneurysms with an accuracy of about 90% (White et al., 2000). Sensitivity was much greater for detection of aneurysms larger than 3 mm (94%) than for detection of aneurysms 3 mm or smaller (38%). Diagnostic accuracy was similar for anterior and posterior circulation aneurysms (Fig. 33B.17). In general, noninvasive imaging evaluation includes a review of T1- and T2-weighted (fast) spin-echo images and T2*-weighted gradient echo images, in addition to the source images and MIP images from the MRA acquisition.
The role of 3D TOF-MRA in assessing intracranial aneurysms before endovascular treatment is adjunctive to the definitive DSA study (Adams et al., 2000). Based on a composite assessment that included aneurysm detection rate, aneurysm morphology, neck interpretation, and branch vessel relationship to the aneurysm, Adams and colleagues found MRA to be inferior to DSA overall and to have missed aneurysms smaller than 3 mm. Nevertheless, MRA provided complementary information to DSA in anatomically complex areas or in the presence of intramural thrombus. The authors applied the assessment to four different types of image data display: axial source images, multiplanar reconstruction (MPR) of the source image data, MIP images, and 3D isosurface-rendered images. Among these types of images, the MPR and 3D isosurface-rendered images were comparable to the DSA images in all categories of the composite assessment, whereas the MIP images scored poorly in all categories except aneurysm detection. These findings indicate that better noninvasive characterization of aneurysms with TOF-MRA can be achieved by adding MPR or 3D isosurface-rendered images to the source and MIP images that are now routinely reviewed in clinical practice.
Endovascularly coiled intracranial aneurysms are increasingly being followed with MRA imaging to detect aneurysm recurrences, although the data remain mixed regarding its accuracy (Fig. 33B.18). One meta-analysis evaluated 16 studies that compared 1.5T TOF-MRA and 1.5T CE-MRA with DSA in the follow-up of coiled intracranial aneurysms. Pooled sensitivity and specificity of TOF-MRA for the detection of residual flow within the aneurysmal neck or body were 83.3% and 90.6%, respectively. Pooled sensitivity and specificity of CE-MRA for the detection of residual flow were 86.8% and 91.9%, respectively, but were not found to be significantly different. The included studies were of moderate methodological quality, and all pooled estimates were subject to heterogeneity (Kwee and Kwee, 2007). A prospective analysis was performed to compare TOF-MRA and CE-MRA at 1.5T and 3T to a reference standard of DSA in the evaluation of previously coiled intracranial aneurysms. For the detection of any aneurysm remnant, the sensitivity was 90%, 85%, 88%, and 90% for 1.5T TOF, 1.5T CE, 3T TOF, and 3T CE-MRA, respectively. These sensitivities dropped to 50%, 67%, 50%, and 67%, respectively, for the detection of only larger (class 3 and 4) aneurysm remnants, because several of these remnants were underclassified as a smaller remnant by MRA. CE-MRA at 1.5T and 3T had a better sensitivity for larger remnants than TOF-MRA, which may be related to greater flow-related artifacts within larger aneurysm remnants on TOF-MRA compared with the luminal contrast-filling characteristics of aneurysms on CE-MRA. Specificities of these four MRA techniques for detecting any aneurysm remnant were 52%, 65%, 52%, and 64%, respectively. Specificities improved to 85%, 84%, 85%, and 87%, respectively, for the detection of larger (class 3 and 4) aneurysm remnants, reflecting the difficulty in detecting smaller remnants with MRA. Regarding the detection of any aneurysm growth since previous comparison angiograms, sensitivities for these MRA techniques were 28%, 28%, 33%, and 39%, respectively, and specificities were 93%, 95%, 98%, and 95%. Although CE-MRA is more likely than TOF-MRA to classify larger aneurysm remnants appropriately, TOF-MRA better identifies the location of coil masses and may be more advantageous if suboptimal CE-MRA contrast bolus is given. Therefore, the advantage of CE-MRA over TOF-MRA remains uncertain and consideration for both exams may be made in the follow-up of patients with coiled intracranial aneurysms (Kaufmann et al., 2010).
Venous Disorders
Imaging of the cerebral venous system differs from approaches tailored to the arterial system because of the lower velocity of venous flow and the morphology of the venous sinuses. For suspected dural sinus occlusion resulting from thrombosis or tumor invasion, the primary technique is 2D TOF-MRA in conjunction with spin-echo imaging. To establish the diagnosis of venous thrombosis, lack of visualization of a vein or sinus on the source images and angiogram must be accompanied by identification of the clot on the spin-echo images at the location of the suspected occlusion (Fig. 33B.19). With the 2D TOF technique, optimal flow enhancement in a section is achieved when it is perpendicular to the flow direction. This condition is best approximated using coronal sections to image the sagittal, straight, and transverse sinuses (as well as the internal cerebral veins, basal veins of Rosenthal, and to a lesser degree, the vein of Galen). The acquisition of coronal sections can be augmented by the acquisition of oblique sagittal sections to allow better flow enhancement in the posterior portions of the transverse sinuses and the cortical veins draining into the superior sagittal sinus. With the 2D TOF-MR venography technique, arterial signal is reduced or eliminated by an axial presaturation band placed across the upper neck below the skull base. A common diagnostic pitfall of the technique is the presence of flow gaps in the transverse sinus. Ayanzen and colleagues (2000) observed these gaps in 31% of patients with normal MRI findings. Flow gaps were not observed in the superior sagittal, straight, or dominant transverse sinuses, so gaps occurring in these locations should raise suspicion of venous obstruction. The authors found that the nondominant transverse sinuses (90% of gaps) or codominant transverse sinuses (10%) that demonstrated the flow gaps were hypoplastic yet patent by DSA.
Alternative techniques for demonstrating cerebral veins and dural venous sinuses lack the robustness of the 2D TOF technique. The 3D TOF technique suffers from saturation effects and hence frequent signal loss in the veins and dural sinuses. The 2D phase-contrast technique is limited by gradient heterogeneity, eddy currents, aliasing artifacts, and lower spatial resolution. Although the phase-contrast technique can be useful in differentiating very slow flow in the dural sinuses from thrombosis, it was recently found to be inferior to the 2D TOF technique and a contrast-enhanced 3D FLASH (fast low-angle shot) MRA technique in displaying the normal septal veins, internal cerebral veins, and the basal veins (Kirchhof et al., 2002). Both the 3D FLASH technique and a 3D magnetization-prepared, rapid-acquisition gradient echo MRI technique (Liang et al., 2001) are contrast-enhanced methods and reportedly are superior to the 2D TOF technique in depicting normal venous structures, especially in overcoming the flow gap artifact. These contrast-enhanced techniques, though, have two potential limitations: (1) both techniques involve rapid acquisition (1-2 minutes per 3D volume), so the intensity of the intravascular signal depends on the timing of the contrast infusion relative to data acquisition, and (2) chronic thrombus may enhance with gadolinium and mimic a patent lumen. Consequently, these two contrast-enhanced techniques should be viewed as adjuncts to the 2D TOF technique.
Vascular Malformations
Traditional MRA methods (2D and 3D TOF and PC-MRA) have played a secondary role to DSA in evaluating intracranial arteriovenous malformations (AVMs) because of a lack of consistent and complete demonstration of all components of an AVM: feeding arteries, nidus, and draining veins. For this reason, and because of the general impression that TOF-MRA adds little to the spin-echo MRI findings useful for preliminary staging of an AVM (nidus size and location and central versus peripheral pattern of venous drainage in the Spetzler-Martin Grading Scale) before definitive DSA, many investigators have considered TOF-MRA of AVMs superfluous (Table 33B.1). Phase-contrast techniques have been used by some investigators to estimate blood flow velocities and volume flow rates in the largest arteries supplying the AVM. A high-resolution 3D gradient echo technique based on the paramagnetic property of deoxyhemoglobin has been used to detect cerebral veins with submillimeter resolution, resulting in greater sensitivity in identifying the presence of small AVMs. But compared with TOF-MRA, the technique provides poorer detection of feeding arteries and is markedly limited in its delineation of nidus size and shape when there are susceptibility artifacts from nearby bone, air, or blood products (hemosiderin) (Essig et al., 1999). High-resolution, real-time, auto-triggered, elliptic, centric-ordered 3D CE-MRA (Farb et al., 2001) and lower-resolution time-resolved 2D CE-MRA (Klisch et al., 2000) have also been applied to the evaluation of AVMs and the results compared with those of TOF-MRA and intraarterial DSA. In an initial investigation, Farb and colleagues found that their 3D CE-MRA technique was superior to 3D TOF-MRA, particularly in depicting nidus and draining veins. The 3D CE-MRA technique consistently showed AVM components and their spatial relationships on MIP images and was equivalent to DSA in depicting AVM components in 70% to 90% of cases (total patients = 10), based on blinded independent assessments by two experienced neuroradiologists. Several small studies prospectively examined 4D CE-MRA at 3T for evaluating AVM and found that 4D CE-MRA yielded 100% accuracy in classifying Spetzler-Martin classification (nidus size, venous drainage, eloquence) of cerebral AVMs when compared to DSA. One study demonstrated a 93% rate of detection of feeding arteries using 4D MRA alone. However, the combined use of selective ASL provided additional functional or anatomical information in 4 of 16 cases (25%), enabling the detection of a cross-filling feeding artery that was not identified by 4D MRA without selective arterial spin labeling (ASL) technique, and improved sensitivity to 96% (Kukuk et al., 2010). These novel techniques may prove to be a noninvasive alternative to DSA in the assessment of patients with cerebral AVMs (Hadizadeh et al., 2008).
Dural arteriovenous fistulas (AVFs) most commonly involve the cavernous, transverse, and sigmoid sinuses along the skull base. Arterial feeders not seen on spin-echo MR sometimes are detected on 3D TOF-MRA but much less often than on catheter angiography. Transverse and sigmoid sinus occlusion and dilated cortical veins are detected better by MRA than spin-echo imaging, yet neither technique achieves the accuracy of catheter angiography. Traditional 3D TOF-MRA is useful in detecting cavernous sinus fistulas, because flow enhancement in the cavernous sinus and contiguous veins can provide evidence of the fistula (Fig. 33B.20). This finding must be regarded with caution because venous flow signal has been observed in the cavernous sinus and inferior petrosal sinus in a variable percentage (from 4% to 36%, depending on unspecified technical differences between MR scanners) of patients without clinical evidence of carotid-cavernous fistula (Ouanounou et al., 1999). Farb et al. compared the use of 3T time-resolved MRA for the diagnosis and classification of a cranial dural AVF with DSA. Patients underwent a commercially available contrast-enhanced time-resolved imaging of contrast kinetics (TRICKS) MRA (trMRA) technique that combines k-space segmentation into central, mid, and peripheral zones with superimposed elliptic centric-view ordering. High temporal resolution is obtained by sampling the central zones of the k-space at a more frequent rate than peripheral zones. The high spatial and temporal resolution allows for identification of dural AVFs by identifying both early opacification of a dural sinus and reflux into the connecting cortical veins if present. In 93% (39/42) of dural AVF cases, investigators had unanimously correct interpretations of the trMRA to correctly identify or exclude all fistulas and accurately classify them when found. The small series suggests that trMRA may be a reliable technique in the screening and surveillance of DAVFs in certain clinical situations, but further validation studies are required (Farb et al., 2009).
Spine Disorders
Spinal MRA is used as an adjunct to MRI to improve the visibility of the millimeter-sized intradural vessels and to help differentiate abnormal from normal ones. The combined MR examination provides better characterization of spinal vessels and thus more effective noninvasive screening for vascular lesions (e.g., dural AVFs) than MRI alone (Saraf-Lavi et al., 2002). The improved screening facilitates decisions regarding invasive catheter angiography for definitive diagnosis and endovascular treatment. The combined MR examination also allows the largest normal vessels to be localized noninvasively before surgical or endovascular procedures that carry a risk of cord injury (Yamada et al., 2000).
Enhancement of the intradural vessels with gadolinium contrast agents has been found necessary for optimal detection on MRA. The 3D CE-MRA technique with steady-state conditions (i.e., TOF pulse sequence parameters) detects the largest intradural veins in healthy volunteers: the posterior and anterior median veins and the great medullary veins draining from the surface of the cord to the epidural space. This technique, and to a lesser extent the 3D phase-contrast technique, also detects the abnormally enlarged and tortuous veins draining dural AVFs (Fig. 33B.21) and intramedullary AVMs. In detecting the presence of dural AVFs, the steady-state 3D CE-MRA technique combined with spin-echo MRI had a sensitivity ranging from 80% to 100%, specificity of 82%, and accuracy of 81% to 94% in a randomized blinded review by 3 neuroradiologists of 11 control subjects and 20 patients with proven dural AVFs (Saraf-Lavi et al., 2002). More importantly, in determining the vertebral level of the fistula, the correct level was predicted in 73% of cases by combined MRA and MRI, representing a significant improvement over MRI alone. Improved noninvasive localization of the fistula level potentially expedites the subsequent invasive catheter angiography study. Preliminary studies of spinal vascular malformations using time-resolved or fast 3D CE-MRA indicate that such first-pass studies may provide better depiction of the dural AVF in the neural foramen because of diminished extradural venous enhancement and improved visibility of the feeding arteries of AVMs (Binkert et al., 1999). Studies have also shown that patients undergoing catheter angiography following MRA require half the fluoroscopy time and half the volume of iodinated contrast if the level and side of the fistula have been identified on screening MRA (Luetmer et al., 2005). MRA and CTA are reported to have similar sensitivities in screening for the level of the artery of Adamkiewicz (Yoshioka et al., 2003).
The use of 3T time-resolved spine MRA has been recently investigated for presurgical localization of the artery of Adamkiewicz prior to reimplantation of the feeding intercostal artery, lumbar artery, or both during aortic aneurysm repair. Bley et al. identified the artery of Adamkiewicz and the location of the feeding intercostal and/or lumbar artery with high confidence in 88% of cases (68 patients). The artery of Adamkiewicz and the anterior spinal artery were identified and differentiated from the great anterior radiculomedullary vein, even in patients with substantially altered hemodynamics from aortic disease (Bley et al., 2010).
Ultrasound
Methods
The physics and underlying methodology of ultrasound techniques used in clinical practice is an extensive topic that has been explained in detail elsewhere. Ultrasound studies are often reported in the relatively simplistic terms of flow-velocity measurements and associated diagnoses of steno-occlusive lesions in the cerebral circulation. Comprehending the physical basis of such findings may provide the clinician with an even greater appreciation for subtle findings and insight on the strengths and limitations of these ultrasound techniques (Kremkau, 2006; Tegeler and Ratanakorn, 1999).
In brightness-mode (B-mode) imaging, the transducer is swept in a single plane to produce a 2D structural and anatomical display of a slice of tissue based on its acoustic properties (Tegeler et al., 2005). These are static images, but the image can be updated 15 to 30 times per second so that it appears to be moving in real time. Duplex ultrasonography makes use of both pulsed-wave Doppler ultrasound and B-mode imaging to obtain both hemodynamic and anatomical information (Fig. 33B.22). Color-flow imaging (CFI) uses Doppler flow-velocity information obtained from multiple sample volumes within the image, which is then color-coded based on the speed and direction of flow and overlaid onto the appropriate anatomical site in the grayscale B-mode image (Fig. 33B.23) (Tegeler et al., 2005). Modern transducers for extracranial high-resolution B-mode carotid imaging operate at frequencies of 7.5 to 10.0 MHz, whereas those used for transcranial CFI operate at 2 to 3 MHz. Power Doppler imaging (PDI) is a variation of CFI that uses the integrated intensity or amplitude of power in the Doppler spectrum to provide information regarding the amount of blood flow detected at each point, rather than the mean velocity (Bluth et al., 2000; Griewing et al., 1996).
Techniques
Carotid Ultrasonography
Because of predictable hemodynamic changes as stenosis develops, duplex Doppler ultrasound can be used to estimate the severity of stenosis. Very mild degrees of stenosis have little hemodynamic effect and cause little change in flow velocity or in the Doppler spectral waveform. Progressive stenosis first causes increased peak-systolic velocity across the narrowed segment. Additional narrowing causes further increase in the peak-systolic velocity and a disturbed flow pattern, or turbulence, emerges (Fig. 33B.24). Severe stenosis prevents adequate blood-flow volume across the lesion, even though the peak-systolic and end-diastolic velocity values are both increased. In very tight carotid stenosis approaching occlusion, peak-systolic and end-diastolic velocity values may increase even further or may begin to decrease as critical narrowing is reached.
High-resolution B-mode imaging also has a unique ability to evaluate the specific features of atherosclerotic plaques (Fig. 33B.25) (Tegeler et al., 2005). Identifiable characteristics include the distribution of plaque (concentric, eccentric, length), surface features (smooth, irregular, crater), echodensity and presence of any calcification producing acoustic shadowing, and texture (homogeneous, heterogeneous, or intraplaque hemorrhage). The presence of hypoechoic plaques and the presence of plaques that are quite heterogeneous with prominent hypoechoic regions (complex plaque) identify an increased risk of stroke (Polak et al., 1998). High-resolution B-mode imaging is more accurate than Doppler ultrasound testing for defining atherosclerosis of the vessel wall early in the course of the disease. Measurement of the intima-media thickness, which increases in the early stages of plaque formation, has been correlated with risk of cardiovascular disease and is used as a surrogate endpoint for clinical trials assessing whether lipid-lowering medications might slow or reverse atherosclerosis (Polak, 2005). The sensitivity of B-mode imaging for detection of surface ulceration is approximately 77% in plaques causing less than 50% linear stenosis and 41% for plaques causing more than 50% linear stenosis, with no significant differences between B-mode carotid imaging and arteriography. Although associated with a somewhat worse outcome, surface irregularity or crater formation appears to be a less important morphological risk factor than echodensity and heterogeneity.
Advantages of CFI include rapid determination of the presence and direction of blood flow, with more accurate placement of the Doppler sample volume and determination of the angle of insonation. Absence of color filling in what appears to be the vessel lumen provides clues about the presence of a hypoechoic plaque, and the contour of the color column can provide information about surface features. If a crater or ulcer is open to the lumen, color further details the surface architecture. Newer instruments with sensitive CFI designed to detect very low flow velocities are able to accurately differentiate critical stenosis from total occlusion (87%-100% sensitivity, 84% specificity versus angiography), negating the need for conventional angiography (Sitzer et al., 1996). The addition of CFI improves understanding of many unusual anatomical configurations such as kinks or coils. Although difficult to quantify accurately, CFI probably adds approximately 5% to the overall diagnostic accuracy of carotid duplex ultrasound.
Conventional criteria for reporting carotid stenosis use flow velocity to estimate the linear percent stenosis. However, increased flow velocity may be seen in other conditions such as a hyperperfusion state seen in anemia that might be misconstrued as stenosis. To avoid such mistakes, various methods have been devised to evaluate volume flow rate in the extracranial cerebral vessels. Processing techniques such as color velocity imaging quantification (CVI-Q) may be implemented, and normal volume flow rate values (330 mL/minute for women and 375 mL/minute for men) have been defined. Use of the CCA volume flow rate in patients with carotid stenosis reveals characteristic decreases in the rate with progressive stenosis. In some laboratories, measurement of CCA volume flow rate is a standard part of the carotid evaluation for patients whose flow velocity suggests 75% or greater carotid stenosis (Fig. 33B.26); this technique may better delineate hemodynamic changes (Knappertz et al., 1996; Tan et al., 2002). There appears to be an acceptable correlation between results of CVI-Q and Doppler-based methods (Likittanasombut et al., 2006), and diminished extracranial cerebral volume flow rate may identify increased risk for recurrent stroke (Han et al., 2006).
The optimal noninvasive imaging method for determining severity of carotid artery stenosis remains uncertain. MRA and CTA are being used with rapidly increasing frequency to determine the degree of stenosis. Although duplex carotid ultrasound should not be used as the sole method for definitive diagnosis of carotid disease, this inexpensive imaging technique remains a valid screening tool. A systematic review of published studies comparing carotid ultrasound with DSA showed that for distinguishing severe stenosis (70%-99%), duplex carotid ultrasound had a pooled sensitivity of 86% and a pooled specificity of 87%. For recognizing occlusion, duplex carotid ultrasound had a sensitivity of 96% and a specificity of 100% (Nederkorn, van der Graaf, Hunink, 2003). Another study found high concordance rates among CTA, contrast-enhanced MRA, and ultrasound for patients with asymptomatic carotid stenosis (Nonent et al., 2004). However, a study comparing ultrasound and MRA to DSA determined that ultrasound alone would have misassigned 28% of patients to receive carotid endarterectomy, whereas ultrasound combined with CE-MRA reduced this misassignment rate to 17% (Johnston et al., 2000).
Vertebral Ultrasonography
Because posterior circulation cerebrovascular disease is quite common, study of the vertebral arteries is considered part of the routine extracranial duplex ultrasound examination. The same techniques described for use in the carotid arteries can be used to study the vertebral arteries and the proximal subclavian or innominate arteries. As such, there should be duplex Doppler and B-mode imaging of these arterial segments. CFI is also helpful for identification and interrogation of the vertebral arteries. The vertebral artery can virtually always be evaluated in the pretransverse and intertransverse cervical segment of C5-C6, whereas the origin can be studied only on the right in 81% and on the left in 65% of cases. Because there is mostly a low-resistance distal vascular bed, the vertebral artery usually shows a low-resistance Doppler spectral pattern similar to that seen with the ICA. Unlike the carotid arteries, there are no widely accepted criteria for stenosis in the extracranial vertebral artery. As with the carotid system, spectral analysis provides insight into proximal and distal disease. Another confounding factor is contralateral occlusive disease, associated with increased carotid volume flow which may result in an overestimation of the severity of stenosis. Given the variable factors associated with carotid duplex sonography, it has been recommended that each laboratory validate its own Doppler criteria for clinically relevant stenosis and undergo certification by an independent organization such as the Intersocietal Commission for Accreditation of Vascular Laboratories Essentials and Standards for Accreditation in Noninvasive Vascular Testing. Studies have shown that the accuracy of duplex ultrasound examination is much better from accredited versus nonaccredited laboratories (Latchaw et al., 2009).
Transcranial Doppler Ultrasonography
Most commercially available TCD ultrasonography instruments use a low-frequency 2-MHz probe to allow insonation through the cranium. These pulsed-wave Doppler instruments have an effective insonation depth range of 3.0 to 12.0 cm or more that can be evaluated by increments of 2 or 5 mm. At an insonation depth of 50 mm, the sample volume is usually 8 to 10 mm axially and 5 mm laterally. TCD probes also differ from the 4- to 10-MHz transducers used to monitor the progress of intraoperative neurosurgical procedures (Unsgaard et al., 2002). Advantages of TCD include the maneuverability of the relatively small probes, the Doppler sensitivity, and—compared to transcranial color-coded duplex and MRA—the relatively low price of instruments.
Applications
Acute Ischemic Stroke
Transcranial Doppler studies obtained within hours from the onset of symptoms of stroke in the carotid territory may reveal stenosis or occlusion of the distal intracranial ICA or proximal MCA in 70% of patients. When compared with DSA, TCD is more than 85% sensitive and specific in detecting supraclinoid ICA or MCA M1 segment lesions. The use of contrast-enhanced color-coded duplex sonography can be especially useful in this context. The use of TCD in the early hours of stroke may also provide important prognostic information. Patency of the MCA by TCD testing within 6 hours of the onset of stroke symptoms is an independent predictor of better outcome (Allendoerfer et al., 2006).
Transcranial Doppler can also help in monitoring the effect of thrombolytic agents. Testing before and after the administration of streptokinase or tPA can assess the agent’s efficacy in obtaining arterial patency and ascertain continued patency during the days after treatment (Yasaka et al., 1998). In addition, investigators have adjusted the dosage and duration of IV thrombolytic agent administration based on information provided by continuous TCD monitoring. Rapid arterial recanalization is associated with better short-term improvement (Alexandrov et al., 2001). Continuous TCD insonation during IV thrombolysis and for 1 hour thereafter significantly increases the rate of recanalization or improvement in the stroke severity score (Alexandrov et al., 2004). The addition of microbubbles during this period of monitoring may further improve the results. Ultrasound energy has been observed to accelerate enzymatic fibrinolysis, possibly by allowing increased transport of drug molecules into the clot and promoting the motion of fluid throughout the thrombus. This observation has led to studies that allow for real-time monitoring of vessel recanalization while potentially providing additional therapeutic benefit from the ultrasound energy. Studies have demonstrated that IV tPA combined with continuous TCD safely increased recanalization rates and produced a trend toward better functional outcomes compared with IV tPA alone. Other small trials demonstrated an improvement in clot lysis when TCCD was combined with IV tPA. In one small study of patients with acute M1 MCA occlusions, 1.8-MHz pulsed-wave ultrasound combined with IV tPA demonstrated higher recanalization rates than patients who received IV tPA alone (58% versus 22%). However, 16% developed a symptomatic intracranial hemorrhage compared to 6% in the non-ultrasound group (Eggers et al., 2008). Another study using ultrasound-mediated thrombolysis found a 36% rate of symptomatic ICH when IV tPA was administered with a 300-kHz therapeutic ultrasound. These studies highlight the need to determine minimum and safe amounts of ultrasound energy necessary to enhance thrombolysis (Rubiera and Alexandrov, 2010). One meta-analysis looking at various ultrasound technologies found that the rate of symptomatic ICH was 3.8% in the IV tPA plus TCD group, 11.1% in the IV tPA plus TCCD group, 35.7% in the IV tPA plus low-frequency ultrasound group, and 2.9% in the IV tPA–alone group. Complete recanalization rates were higher in patients receiving a combination of TCD with IV tPA compared to patients treated with IV tPA alone (37.2% versus 17.2%) (Tsivgoulis et al., 2010). Administration of microbubbles using Perflutren lipid microspheres remains under investigation and may transmit energy momentum from an ultrasound wave to residual flow to promote further recanalization, thereby enhancing the effect of ultrasound on thrombolysis (Alexandrov et al., 2008; Molina et al., 2009). Additional operator-independent devices, different microbubble-related techniques, and other means of improving sonothrombolysis are currently being studied in clinical trials.
Transcranial power motion–mode Doppler (PMD-TCD) is a technique that along with spectral information simultaneously displays real-time flow signal intensity and direction over 6 cm of intracranial space. One study compared PMD-TCD with CTA and found a sensitivity of 81.8% and specificity of 94% for detecting an acute arterial occlusion. The sensitivity for detecting MCA occlusions was 95.6%, and the specificity was 96.2%. For the anterior circulation, PMD-TCD demonstrated a sensitivity of 100% and specificity of 94.5%. For the posterior circulation, sensitivity was 57.1%, and specificity was 100% (Brunser et al., 2009).
Recent Transient Ischemic Attack or Stroke
Compared to other available methods, ultrasound testing offers a safe, accurate, noninvasive, and less expensive method to evaluate for extracranial cerebrovascular disease. It is considered the initial test of choice for identifying significant carotid stenosis in patients with recent transient ischemic attack (TIA) or stroke (Tegeler and Ratanakorn, 1998). For the carotid territory, this should include duplex ultrasonography, with or without CFI. Reports should address the severity of stenosis based on Doppler flow-velocity measurements. There also must be information provided about the presence of any plaque, as well as the morphology, based on high-resolution B-mode imaging. Additional helpful ultrasound tools include PDI and volume flow rate measurement. Results of carotid ultrasound testing must then be integrated with other available testing modalities if additional information is needed. At present, this often means a combination of ultrasound and MRA or CTA, with DSA reserved for those in whom results of the preceding tests are technically inadequate, equivocal, or contradictory. The combination of ultrasound and MRA is more cost-effective than the use of routine DSA in this setting. However, the best algorithm for evaluation may vary depending on the services and expertise available at each medical center.
Collateral flow patterns associated with severe cervical carotid stenosis or occlusion can also be detected by TCD. They include retrograde flow of the ophthalmic artery and anterior or posterior communicating artery flow toward the hemisphere distal to the stenosed or occluded ICA. Among patients with symptomatic carotid occlusions, one study found that compared with DSA, TCD detection of collateral flow via the major intracerebral collateral branches had a sensitivity of 82% and a specificity of 79% in the anterior portion of the circle of Willis. In the posterior communicating artery, TCD demonstrated a sensitivity of 76% and a specificity of 47% (Hendrikse et al., 2008). Lesions causing stenosis of the V4 segment of the vertebral artery and the proximal basilar artery can be imaged by TCD. Focal increases of the peak-systolic and mean velocities to 120 cm/sec and 80 cm/sec or more, respectively, at depths of insonation corresponding to these arterial segments are considered significant. Velocities often exceed 200 cm/sec with lesions causing more than 50% stenosis. Compared to DSA, the sensitivity of TCD is approximately 75% in detecting vertebrobasilar stenotic lesions, and its specificity exceeds 85%. Frequent variation in the size and course of the vertebrobasilar trunk and its contribution of collateral flow to the anterior cerebral circulation are the main reasons for these relatively low figures. Contrast media and TCCD imaging can be particularly helpful in this setting (Stolz et al., 2002).
In patients with extracranial carotid disease, these signals are associated with a history of recent TIAs or cerebral infarction in the distribution of the insonated artery, and they correlate with the presence of ipsilateral severe stenosis and plaque ulceration. They are detected mainly during the week following symptoms of cerebral ischemia and resolve afterward. MES also can be detected in subjects with cardiac prosthetic valves but often correspond to gaseous microbubbles in that setting. They are less common in adequately anticoagulated patients with atrial fibrillation. The clinical impact of microembolus detection studies remains limited at this time. The presence of these signals in an arterial territory is useful in identifying proximal “active” lesions. This is especially relevant when a symptomatic patient has more than one potential lesion, such as cervical carotid stenosis and atrial fibrillation, or a suboptimal history. In this situation, laboratory data can help identify the specific cause of cerebral infarction. In addition, because the presence of MES is predictive of future cerebral ischemic events in the insonated artery’s territory (Markus et al., 2005), detecting these signals may affect therapeutic decisions. In the future, microembolus detection studies may be useful in monitoring the effect of antithrombotic agents (Junghans and Siebler, 2003). Microemboli monitoring is also of interest in the context of CEA and coronary artery bypass graft surgery. MES have been reported in 43% of patients with symptomatic carotid stenosis and 10% of patients with asymptomatic carotid stenosis. MES were reported in 25% of symptomatic versus 0% of patients with asymptomatic intracranial stenosis. In patients with cervical artery dissection presenting with TIA or stroke, 50% had MES, compared to 13% with local symptoms. Among patients with aortic embolism, patients with plaques 4 mm or larger demonstrated MES more frequently than patients with smaller plaques. MES has been shown to be useful for risk stratification in patients with carotid stenosis, but data from published studies remain insufficient to reliably predict future events in patients with intracranial stenosis, cervical artery dissection, and aortic embolism (Ritter et al., 2008).
Extracranial Stenotic Lesions
Ultrasound remains a safe and noninvasive method to monitor patients with carotid or vertebral artery disorders. Periodic evaluation can be helpful for assessing the progression or regression of existing plaques or the development of new lesions, whether symptomatic or asymptomatic. The timing of follow-up carotid testing must be individualized depending on the severity and type of lesions, as well as the onset of new or recurrent symptoms. The identification of asymptomatic carotid stenosis has become an important clinical mandate since the Asymptomatic Carotid Atherosclerosis Study (ACAS) showed the benefit of CEA in asymptomatic patients with 60% to 99% stenosis, when compared with treatment with 325 mg of aspirin daily (Executive Committee for the Asymptomatic Carotid Atherosclerosis Study, 1995). Yet, it is not cost-effective to screen the entire population, even with ultrasound. Asymptomatic individuals with cervical bruits should be studied, even though bruits are often due to another cause. Patients with multiple risk factors probably warrant study, but the clinical utility of this has not yet been confirmed. Practice guidelines are being developed for carotid screening in high-risk individuals to identify stenosis that may need clinical treatment or intervention (Qureshi et al., 2007). If vessel disease is identified, stenosis of less than 50% might be initially restudied in 12 to 24 months, whereas lesions with 50% to 75% stenosis and uncomplicated plaques might wait 6 to 12 months. For 50% to 75% stenosis with complicated plaque features, or for more than 75% stenosis, initial restudy at 3 to 6 months is appropriate. Lack of progression for several years allows lengthened intervals before restudy. When evidence of asymptomatic progression is present, a shorter interval is recommended. Development of new symptoms should prompt urgent reevaluation. After CEA, repeat ultrasound is often done at approximately 1 month after surgery and then yearly to identify potential restenosis.
Large population studies such as the Atherosclerosis Risk in Communities and the Cardiovascular Health Study have documented the association between risk factors and intima-media thickening in the wall of the carotid artery on B-mode imaging (Polak, 2005; Polak et al., 1998). This may represent an early stage in the development of atherosclerosis; the presence of significant thickening correlates with risk of heart attack as well as abnormalities on MRI of the brain. Further investigations remain ongoing regarding the clinical utility of identifying increased intima-media thickness values, but it has been suggested that B-mode imaging for evaluation of intima-media thickness should be used clinically to identify patients at high risk for coronary or cerebrovascular events or to assess responses to risk factor modification (AHA Prevention Conference V Writing Group III, 2000; Polak, 2005). The hope is that such early identification of atherosclerotic changes will allow intervention to prevent later development of clinical events.
Intracranial Stenotic Lesions
Intracranial atherosclerotic plaques are dynamic lesions that may increase in degrees of stenosis or regress over relatively short periods of time. TCD enables noninvasive monitoring of these lesions. It is often obtained at baseline in conjunction with DSA, CTA, or MRA and is subsequently repeated during the follow-up period (Fig. 33B.27). Monitoring also enables detection of new atherosclerotic plaques. However, clinical experience is limited, and further prospective investigations are needed to make recommendations regarding the frequency and timing of follow-up studies.
Aneurysmal Subarachnoid Hemorrhage
Vasoconstriction of intracerebral arteries is the leading cause of delayed cerebral infarction and mortality after aneurysmal subarachnoid hemorrhage. Vasospasm is clinically detected 3 or 4 days after the hemorrhage and usually resolves after day 12. Although the exact cause of vasospasm remains unknown, its presence correlates with the volume and duration of exposure of an intracranial artery to the blood clot. Laboratory and animal models indicate that blood breakdown products can lead to vasoconstriction. The detection of vasospasm is important because it may potentially be treated with medications, hemodynamic management, and endovascular interventions. These treatments are not innocuous, so the ability to noninvasively detect and monitor vasospasm has considerable clinical importance. Although vasospasm can be angiographically detected in 30% to 70% of patients with aneurysmal subarachnoid hemorrhage, only 20% to 40% develop clinical signs of cerebral ischemia. Thus, the presence of vasospasm is not a sufficient condition for development of a clinical focal ischemic deficit. Several factors including the severity of spasm, presence of collateral flow, condition of the patient’s intravascular volume, and cerebral perfusion pressure are considered mitigating factors. TCD studies show an increase in the flow velocities of basal cerebral arteries, usually starting on day 4 after subarachnoid hemorrhage and peaking by days 7 to 14 (Fig. 33B.28). Although a diffuse increase in velocities is often detected in patients with severe hemorrhage, arterial segments in close proximity to the subarachnoid blood clot usually have the highest velocities.
Severe vasospasm in an arterial segment can be associated with reduced regional cerebral blood flow in the artery’s distal territory. There is a linear inverse relationship between the severity of vasospasm and the amplitude of flow-velocity increase in an arterial segment. It is valid until the vasoconstriction is so severe that the flow volume is reduced, flow velocities drop, and the TCD signal becomes difficult to detect. The linear relationship can also be affected by several factors, including the presence of hyperperfusion. Angiographic studies confirm the presence of at least some degree of MCA vasospasm when the mean flow velocities are higher than 100 cm/sec, but values below 120 cm/sec are not usually considered clinically significant. Mean velocities in the 120 to 200 cm/sec range correspond to 25% to 50% angiographically determined diameter reduction; values exceeding 200 cm/sec correspond to more than 50% luminal narrowing (Sloan et al., 1999). The 200 cm/sec threshold and rapid flow-velocity increases exceeding 50 cm/sec on consecutive days are associated with subsequent infarction. TCD is used also to monitor the effects of endovascular treatment of vasospasm. Flow velocities decrease after successful angioplasty or papaverine infusion. Persistent increases after treatment indicate either extension of vasospasm to new arterial segments or hyperemia in the treated arterial segment and may constitute a valid reason for repeat cerebral angiography.
The accuracy of TCD in detecting vasospasm depends to some degree on the location of the involved arterial segment. Although TCD criteria are more than 90% specific in detecting MCA and ACA vasospasm, they are respectively 80% and less than 50% sensitive in detecting disease in these arterial segments (Sloan et al., 1999). Basilar artery vasospasm is detected with an approximate sensitivity of 75% and specificity of 80%. Several factors including the effects of hyperemia, increased intracranial pressure (ICP) and blood pressure changes, the presence of vasospasm in convexity branches not accessible by TCD, and difficulties in assessing vasospasm by angiography contribute to these findings. Because of these limitations in accuracy, the combined use of TCD and SPECT or xenon-enhanced CT has been advocated, with the expectation that it will provide a more comprehensive and accurate assessment of the clinical condition. Overall, however, TCD is considered to have acceptable accuracy for the evaluation of vasospasm in aneurysmal subarachnoid hemorrhage. It is a useful tool with limitations that must be taken into consideration in the clinical setting.
Brain Death
A characteristic pattern of changes can be detected by TCD in patients with increased ICP. Early findings consist of a mild decrease in the diastolic flow velocity and an increase in the difference between peak-systolic and end-diastolic velocities. When ICP increases further and reaches the diastolic blood pressure level, flow stops during diastole, and the corresponding flow velocity drops to zero; flow continues during systole, and spiky systolic peaks are observed. A further increase in ICP is associated with a reverberating flow pattern, with forward flow in systole and retrograde flow in diastole (see Fig. 33B.28). The net volume of flow decreases and can reach zero. At cerebral perfusion pressure values close to zero, either small systolic spikes are observed (Fig. 33B.29), or no signal at all is detected. This corresponds to a complete arrest of flow as demonstrated by cerebral angiography. The pattern of TCD changes is not specific to a particular neurological disease and can occur in a variety of conditions associated with increased ICP.
These changes are also observed in patients clinically diagnosed as brain dead. Experience remains variable among different institutions, and one retrospective study found that TCD evaluation was able to confirm brain death in 57% of patients but remained inconclusive in 43% (Sharma et al., 2010). Another recent study found that the specificity of TCD testing was 100%, with a sensitivity of 82.1%. When the evaluation was augmented by insonation of the extracranial ICA, the sensitivity was increased to 88% by allowing the detection of cerebral circulatory arrest in patients lacking temporal windows. The addition of serial examinations further increased sensitivity to 95.6% (Alexandrov et al., 2010). Thus, although TCD is useful in detecting cerebral circulatory arrest, it cannot be recommended as the sole diagnostic test for the diagnosis of brain death. The latter has to be established based on the clinical presentation and neurological examination findings. TCD and other laboratory tests can help confirm the clinical impression.
Sickle Cell Disease
An occlusive vasculopathy characterized by a fibrous proliferation of the intima often involves the basal cerebral arteries of patients with sickle cell disease. Cerebral infarction is a common complication of this vasculopathy and has a frequency of approximately 5% to 15%. As in all patients with anemia, flow velocities are diffusely increased in individuals with sickle cell anemia. Additional focal velocity increases in the basal cerebral arteries can be detected in some subjects. A time-averaged mean of the maximum velocity of 200 cm/sec or greater in the distal ICA and proximal MCA identifies neurologically asymptomatic children at an increased risk for first-time stroke (Adams et al., 1998). In addition to standard insonation techniques with the TCD probe, a recent study determined that extending the submandibular approach to include infrasiphon portions of the ICA increased the sensitivity to better identify sickle cell patients with potential sources of cerebral infarction (Gorman et al., 2009). Periodic red blood cell transfusion is associated with a 90% reduction in the rate of stroke. A Clinical Alert from the National Heart, Lung and Blood Institute recommended that children with sickle cell disease between ages 2 and 16 receive baseline TCD testing and that those with normal study results be restudied every 6 months (National Heart, Lung and Blood Institute, 1997). Discontinuation of transfusion therapy can result in a reversal of abnormal blood-flow velocities and stroke (STOP 2 Trial, 2005).
Periprocedural Monitoring
Carotid endarterectomy (CEA) and carotid artery stenting (CAS) remain important interventions for certain cases of asymptomatic and symptomatic carotid stenosis. Monitoring is often performed to identify and correct periprocedural events that can lead to cerebrovascular complications. Monitoring tests currently in use for CEA include electroencephalography. These tests are useful in detecting cerebral hypoperfusion or its consequence, cerebral ischemia, and investigations remain ongoing to determine their effectiveness in reducing the perioperative stroke rate. TCD monitoring during CEA shows a consistent pattern of flow-velocity changes. The most significant changes occur at the time of carotid clamping, with persistent and severe flow-velocity decreases to less than 15% of pre-clamp values in up to 10% of patients (Fig. 33B.30). Patients with velocities decreasing to this level usually are considered candidates for shunting. Although definitive TCD criteria for shunting have not yet been established, a post-clamp peak-systolic or mean flow-velocity decrease to less than 30% of the pre-clamp value is often considered an acceptable criterion.
TCD monitoring also has the unique capability of detecting microembolism as it occurs. This provides a considerable edge to TCD when compared with other monitoring techniques, because the majority of perioperative infarcts are thought to be secondary to cerebral embolism. Microemboli are detected at specific stages of surgery; dissection, clamp insertion and release, and the immediate postoperative period are the high-risk periods (Fig. 33B.31). The presence of solid and gaseous microemboli in patients undergoing CEA and/or carotid stenting have been associated with procedure-related acute ipsilateral ischemic strokes on MRI as well as postoperative cognitive decline (Skjelland et al., 2009). One study evaluating patients who underwent carotid endarterectomy under TCD monitoring found that low MCA mean blood-flow velocity (≤28 cm/sec) during carotid dissection was significantly associated with new postoperative neurological deficits in patients with 10 or greater MES during carotid dissection. This combined evaluation resulted in improved specificity and positive predictive value when compared with either criterion used alone (Ogasawara et al., 2008). TCD remains a relative newcomer to the field of periprocedural monitoring and provides useful information to potentially avert cerebrovascular complications.
Adams R.J., McKie V.C., Hsu L., et al. Prevention of first stroke by transfusions in children with sickle cell anemia and abnormal results on transcranial Doppler ultrasonography. N Engl J Med. 1998;339:5-11.
Adams W.M., Laitt R.D., Jackson A. The role of MR angiography in the pretreatment assessment of intracranial aneurysms: a comparative study. AJNR Am J Neuroradiol. 2000;21:1618-1628.
AHA Prevention Conference V Writing Group III. Noninvasive tests of atherosclerotic burden. Circulation. 2000;101:e16-e22.
Alexandrov A.V. Cerebrovascular Ultrasound in Stroke Prevention and Treatment. New York: Blackwell Publishing; 2004.
Alexandrov A.V., Burgin W.S., Demchuk A.M., et al. Speed of intracranial clot lysis with intravenous tissue plasminogen activator therapy. Circulation. 2001;103:2897-2902.
Alexandrov A.V., Mikulik R., Ribo M., et al. A pilot randomized clinical safety study of sonothrombolysis augmentation with ultrasound-activated perflutren-lipid microspheres for acute ischemic stroke. Stroke. 2008;39:1464-1469.
Alexandrov A.V., Molina C.A., Grotta J.C., et al. Ultrasound-enhanced systemic thrombolysis for acute ischemic stroke. N Engl J Med. 2004;351:2170-2178.
Alexandrov A.V., Tsivgoulis G., Rubiera M., et al. End-diastolic velocity increase predicts recanalization and neurological improvement in patients with ischemic stroke with proximal arterial occlusions receiving reperfusion therapies. Stroke. 2010;41:948-952.
Allendoerfer J., Goertler M., von Reutern G.M., et al. Prognostic relevance of ultra-early Doppler sonography in acute ischaemic stroke: a prospective multicentre study. Lancet Neurology. 2006;5:835-840.
Asif A., Epstein M. Prevention of radiocontrast-induced nephropathy. Am J Kidney Dis. 2004;44:12-24.
Ayanzen R.H., Bird C.R., Keller P.J., et al. Cerebral MR venography: normal anatomy and potential diagnostic pitfalls. AJNR Am J Neuroradiol. 2000;21:74-78.
Bash S., Villablanca J.P., Jahan R., et al. Intracranial vascular stenosis and occlusive disease: evaluation with CT angiography, MR angiography, and digital subtraction angiography. AJNR Am J Neuroradiol. 2005;26:1012-1021.
Binaghi S., Maeder P., Uske A., et al. Three-dimensional computed tomography angiography and magnetic resonance angiography of carotid bifurcation stenosis. Eur Neurol. 2001;46:25-34.
Binkert C.A., Kollias S.S., Valavanis A. Spinal cord vascular disease: characterization with fast three-dimensional contrast-enhanced MR angiography. AJNR Am J Neuroradiol. 1999;20:1785-1793.
Bley T.A., Duffek C.C., Francois C.J., et al. Presurgical localization of the artery of Adamkiewicz with time-resolved 3.0-T MR angiography. Radiology. 2010;255:873-881.
Bluth E.I., Sunshine J.H., Lyons J.B., et al. Power Doppler imaging: initial evaluation as a screening examination for carotid stenosis. Radiology. 2000;215:791-800.
Bowen B.C. MR angiography versus CT angiography in the evaluation of neurovascular disease. Radiology. 2007;245:357-361.
Brunser A.M., Lavados P.M., Hoppe A., et al. Accuracy of transcranial Doppler compared with CT angiography in diagnosing arterial obstructions in acute ischemic strokes. Stroke. 2009;40:2037-2041.
Camargo E.C., Furie K.L., Singhal A.B., et al. Acute brain infarct: detection and delineation with CT angiographic source images versus nonenhanced CT scans. Radiology. 2007;244:541-548.
Debrey S.M., Yu H., Lynch J.K., et al. Diagnostic accuracy of magnetic resonance angiography for internal carotid artery disease: a systematic review and meta-analysis. Stroke. 2008;39:2237-2248.
Delgado Almandoz J.E., Yoo A.J., Stone M.J., et al. Systematic characterization of the computed tomography angiography spot sign in primary intracerebral hemorrhage identifies patients at highest risk for hematoma expansion: the spot sign score. Stroke. 2009;40:2994-3000.
Delgado Almandoz J.E., Yoo A.J., Stone M.J., et al. The spot sign score in primary intracerebral hemorrhage identifies patients at highest risk of in-hospital mortality and poor outcome among survivors. Stroke. 2010;41:54-60.
de Monye C., Dippel D.W., Dijkshoorn M.L., et al. MDCT detection of fibromuscular dysplasia of the internal carotid artery. AJR Am J Roentgenol. 2007;188:W367-9.
Diekmann S., Siebert E., Juran R., et al. Dose exposure of patients undergoing comprehensive stroke imaging by multidetector-row CT: comparison of 320-detector row and 64-detector row CT scanners. AJNR Am J Neuroradiol. 2010;31:1003-1009.
Eggers J., Konig I.R., Koch B., et al. Sonothrombolysis with transcranial color-coded sonography and recombinant tissue-type plasminogen activator in acute middle cerebral artery main stem occlusion: results from a randomized study. Stroke. 2008;39:1470-1475.
Elijovich L., Kazmi K., Gauvrit J.Y., et al. The emerging role of multidetector row CT angiography in the diagnosis of cervical arterial dissection: preliminary study. Neuroradiology. 2006;48:606-612.
Escudero D., Otero J., Marques L., et al. Diagnosing brain death by CT perfusion and multislice CT angiography. Neurocrit Care. 2009;11:261-271.
Essig M., Reichenbach J.R., Schad L.R., et al. High-resolution MR venography of cerebral arteriovenous malformations. Magn Reson Imaging. 1999;17:1417-1425.
Executive Committee for the Asymptomatic Carotid Atherosclerosis Study. Endarterectomy for asymptomatic carotid artery stenosis. Executive Committee for the Asymptomatic Carotid Atherosclerosis Study. JAMA. 1995;273:1421-1428.
Fain S.B., Riederer S.J., Bernstein M.A., et al. Theoretical limits of spatial resolution in elliptical-centric contrast-enhanced 3D-MRA. Magn Reson Med. 1999;42:1106-1116.
Fain S.B., Riederer S.J., Huston J.III, et al. Embedded MR fluoroscopy: high temporal resolution real-time imaging during high spatial resolution 3D MRA acquisition. Magn Reson Med. 2001;46:690-698.
Farb R.I., Agid R., Willinsky R.A., et al. Cranial dural arteriovenous fistula: diagnosis and classification with time-resolved MR angiography at 3T. AJNR Am J Neuroradiol. 2009;30:1546-1551.
Farb R.I., McGregor C., Kim J.K., et al. Intracranial arteriovenous malformations: real-time auto-triggered elliptic centric-ordered 3D gadolinium-enhanced MR angiography—initial assessment. Radiology. 2001;220:244-251.
Foo T.K.F., Saranathan M., Prince M.R., et al. Automated detection of bolus arrival and initiation of data acquisition in fast, three-dimensional, gadolinium-enhanced MR angiography. Radiology. 1997;203:275-280.
Frampas E., Videcoq M., de Kerviler E., et al. CT angiography for brain death diagnosis. AJNR Am J Neuroradiol. 2009;30:1566-1570.
Furst G., Hofer M., Steinmetz H., et al. Intracranial stenoocclusive disease: MR angiography with magnetization transfer and variable flip angle. AJNR Am J Neuroradiol. 1996;17:1749-1757.
Gaikwad A.B., Mudalgi B.A., Patankar K.B., et al. Diagnostic role of 64-slice multidetector row CT scan and CT venogram in cases of cerebral venous thrombosis. Emerg Radiol. 2008;15:325-333.
Gorman M.J., Nystrom K., Carbonella J., et al. Submandibular TCD approach detects post-bulb ICA stenosis in children with sickle cell anemia. Neurology. 2009;73:362-365.
Griewing B., Morgenstern C., Driesner F., et al. Cerebrovascular disease assessed by color flow and power Doppler ultrasonography. Comparison with digital subtraction angiography in internal carotid artery stenosis. Stroke. 1996;27:95-100.
Hadizadeh D.R., von Falkenhausen M., Gieseke J., et al. Cerebral arteriovenous malformation: Spetzler-Martin classification at subsecond-temporal-resolution four-dimensional MR angiography compared with that at DSA. Radiology. 2008;246:205-213.
Han J.H., Ho S.S.Y., Lam W.W.M., et al. Total cerebral blood flow estimated by color velocity imaging quantification ultrasound: a predictor for recurrent stroke? J Cereb Blood Flow Metab. 2006:1-7.
Hendrikse J., Klijn C.J., van Huffelen A.C., et al. Diagnosing cerebral collateral flow patterns: accuracy of non-invasive testing. Cerebrovasc Dis. 2008;25:430-437.
Hendrikse J., Zwanenburg J.J., Visser F., et al. Noninvasive depiction of the lenticulostriate arteries with time-of-flight MR angiography at 7.0 T. Cerebrovasc Dis. 2008;26:624-629.
Hirai T., Korogi Y., Ono K., et al. Prospective evaluation of suspected stenoocclusive disease of the intracranial artery: combined MR angiography and CT angiography compared with digital subtraction angiography. AJNR Am J Neuroradiol. 2002;23:93-101.
Hope T.A., Hope M.D., Purcell D.D., et al. Evaluation of intracranial stenoses and aneurysms with accelerated 4D flow. Magn Reson Imaging. 2010;28:41-46.
Huston J., Nichols D.A., Luetmer P.H., et al. MR angiographic and sonographic indications for endarterectomy. AJNR Am J Neuroradiol. 1998;19:309-315.
Johnson M.B., Wilkinson I.D., Wattam J., et al. Comparison of Doppler ultrasound, magnetic resonance angiographic techniques and catheter angiography in evaluation of carotid stenosis. Clin Radiol. 2000;55:912-920.
Johnston D.C., Goldstein L.B. Clinical carotid endarterectomy decision making: noninvasive vascular imaging versus angiography. Neurology. 2001;56:1009-1015.
Junghans U., Siebler M. Cerebral microembolism is blocked by tirofiban, a selective nonpeptide platelet glycoprotein IIb/IIIa receptor antagonist. Circulation. 2003;107:2717-2721.
Kaminogo M., Hayashi H., Ishimaru H., et al. Depicting cerebral veins by three-dimensional CT angiography before surgical clipping of aneurysms. AJNR Am J Neuroradiol. 2002;23:85-91.
Kang C.K., Park C.A., Lee H., et al. Hypertension correlates with lenticulostriate arteries visualized by 7T magnetic resonance angiography. Hypertension. 2009;54:1050-1056.
Kang C.K., Park C.A., Park C.W., et al. Research: Lenticulostriate arteries in chronic stroke patients visualised by 7 T magnetic resonance angiography. Int J Stroke. 2010;5:374-380.
Kaufmann T.J., Huston J.3rd, Cloft H.J., et al. A prospective trial of 3T and 1.5T time-of-flight and contrast-enhanced MR angiography in the follow-up of coiled intracranial aneurysms. AJNR Am J Neuroradiol. 2010;31:912-918.
Khandelwal N., Agarwal A., Kochhar R., et al. Comparison of CT venography with MR venography in cerebral sinovenous thrombosis. AJR Am J Roentgenol. 2006;187:1637-1643.
Kim S.M., Cha R.H., Lee J.P., et al. Incidence and outcomes of contrast-induced nephropathy after computed tomography in patients with CKD: a quality improvement report. Am J Kidney Dis. 2010;55:1018-1025.
Kirchhof K., Welzel T., Jansen O., et al. More reliable noninvasive visualization of the cerebral veins and dural sinuses: comparison of three MR angiographic techniques. Radiology. 2002;224:804-810.
Klisch J., Strecker R., Hennig J., et al. Time-resolved projection MRA: Clinical application in intracranial vascular malformations. Neuroradiology. 2000;42:104-107.
Knappertz V.A., Tegeler C.H., Myers L.G. Clinical cerebrovascular applications of arterial ultrasound volume flow rate estimates. J Neuroimaging. 1996;6:1-7.
Knauth M., VonKummer R., Jansen O., et al. Potential of CT angiography in acute ischemic stroke. Am J Neuroradiol. 1997;18:1001-1010.
Koelemay M.J., Nederkoorn P.J., Reitsma J.B., et al. Systematic review of computed tomographic angiography for assessment of carotid artery disease. Stroke. 2004;35:2306-2312.
Kollias S.S., Binkert C.A., Ruesch S., et al. Contrast-enhanced MR angiography of the supra-aortic vessels in 24 seconds: a feasibility study. Neuroradiology. 1999;41:391-400.
Kremkau F.W. Diagnostic Ultrasound: Principles and Instruments, seventh ed. St. Louis, MO: Saunders Elsevier; 2006.
Kukuk G.M., Hadizadeh D.R., Bostrom A., et al. Cerebral arteriovenous malformations at 3.0 T: intraindividual comparative study of 4D-MRA in combination with selective arterial spin labeling and digital subtraction angiography. Invest Radiol. 2010;45:126-132.
Kuo P.H., Kanal E., Abu-Alfa A.K., et al. Gadolinium-based MR contrast agents and nephrogenic systemic fibrosis. Radiology. 2007;242:647-649.
Kwee T.C., Kwee R.M. MR angiography in the follow-up of intracranial aneurysms treated with Guglielmi detachable coils: systematic review and meta-analysis. Neuroradiology. 2007;49:703-713.
Latchaw R.E., Alberts M.J., Lev M.H., et al. Recommendations for imaging of acute ischemic stroke: a scientific statement from the American Heart Association. Stroke. 2009;40:3646-3678.
Lenhart M., Framme N., Volk M., et al. Time-resolved contrast-enhanced magnetic resonance angiography of the carotid arteries: diagnostic accuracy and inter-observer variability compared with selective catheter angiography. Invest Radiol. 2002;37:535-541.
Lenhart M., Volk M., Manke C., et al. Stent appearance at contrast-enhanced MR angiography: in vitro examination with 14 stents. Link J Radiol. 2000;217:173-178.
Lettau M., Sauer A., Heiland S., et al. Carotid artery stents: in vitro comparison of different stent designs and sizes using CT angiography and contrast-enhanced MR angiography at 1.5T and 3T. AJNR Am J Neuroradiol. 2009;30:1993-1997.
Leys D., Moulin T., Stojkovic T., et al. Follow up of patients with history of cervical carotid artery dissection. Cerebrovasc Dis. 1995;5:43-49.
Liang L., Korogi Y., Sugahara T., et al. Evaluation of the intracranial dural sinuses with a 3D contrast-enhanced MP-RAGE sequence: prospective comparison with 2D-TOF MR venography and digital subtraction angiography. AJNR Am J Neuroradiol. 2001;22:481-492.
Linn J., Ertl-Wagner B., Seelos K.C., et al. Diagnostic value of multidetector-row CT angiography in the evaluation of thrombosis of the cerebral venous sinuses. AJNR Am J Neuroradiol. 2007;28:946-952.
Likittanasombut P., Reynolds P., Meads D., et al. Volume flow rate of common carotid artery measured by Doppler methods and color velocity imaging quantification (CVI-Q). J Neuroimaging. 2006;16:34-38.
Luetmer P.H., Lane J.I., Gilbertson J.R., et al. Preangiographic evaluation of spinal dural arteriovenous fistulas with elliptic centric contrast-enhanced MR angiography and effect on radiation dose and volume of iodinated contrast material. AJNR Am J Neuroradiol. 2005;26:711-718.
Magarelli N., Scarabino T., Simeone A.L., et al. Carotid stenosis: a comparison between MR and spiral CT angiography. Neuroradiology. 1998;40:367-373.
Marks M.P., Olivot J.M., Kemp S., et al. Patients with acute stroke treated with intravenous tPA 3-6 hours after stroke onset: correlations between MR angiography findings and perfusion- and diffusion-weighted imaging in the DEFUSE study. Radiology. 2008;249:614-623.
Markus H.S., Droste D.W., Kaps M., et al. Dual antiplatelet therapy with clopidogrel and aspirin in symptomatic carotid stenosis evaluated using Doppler embolic signal detection: the clopidogrel and aspirin for reduction of emboli in symptomatic carotid stenosis (CARESS) Trial. Circulation. 2005;111:2233-2240.
Melhem E.R., Caruthers S.D., Faddoul S.G., et al. Use of three-dimensional MR angiography for tracking a contrast bolus in the carotid artery. AJNR Am J Neuroradiol. 1999;20:263-266.
Molina C.A., Barreto A.D., Tsivgoulis G., et al. Transcranial ultrasound in clinical sonothrombolysis (TUCSON) trial. Ann Neurol. 2009;66:28-38.
Nael K., Michaely H.J., Villablanca J.P., et al. Time-resolved contrast-enhanced magnetic resonance angiography of the head and neck at 3.0 Tesla: initial results. Investig Radiol. 2006;41:116-124.
National Heart, Lung and Blood Institute, 1997. Clinical Alert: Periodic Transfusions Lower Stroke Risk in Children with Sickle Cell Anemia. 18 September 1997.
Nederkoorn P.J., van der Graaf Y., Hunink M.G. Duplex ultrasound and magnetic resonance angiography compared with digital subtraction angiography in carotid artery stenosis: a systematic review. Stroke. 2003;34:1324-1332.
Nonent M., Serfaty J.M., Nighoghossian N., et al. Concordance rate differences of 3 noninvasive imaging techniques to measure carotid stenosis in clinical routine practice: results of the CARMEDAS multicenter study. Stroke. 2004;35:682-686.
Norris J.W., Rothwell P.M. Noninvasive carotid imaging to select patients for endarterectomy: is it really safer than conventional angiography? Neurology. 2001;56:990-991.
Nguyen-Huynh M.N., Wintermark M., English J., et al. How accurate is CT angiography in evaluating intracranial atherosclerotic disease? Stroke. 2008;39:1184-1188.
Ogasawara K., Suga Y., Sasaki M., et al. Intraoperative microemboli and low middle cerebral artery blood flow velocity are additive in predicting development of cerebral ischemic events after carotid endarterectomy. Stroke. 2008;39:3088-3091.
Olsrud J., Latt J., Brockstedt S., et al. Magnetic resonance imaging artifacts caused by aneurysm clips and shunt valves: dependence on field strength (1.5 and 3 T) and imaging parameters. J Magn Reson Imaging. 2005;22:433-437.
Optimizing Primary Stroke Prevention in Sickle Cell Anemia (STOP 2) Trial Investigators. Discontinuing prophylactic transfusions used to prevent stroke in sickle cell disease. N Engl J Med. 2005;353:2769-2778.
Ouanounou S., Tomsick T.A., Heitsman C., Holland C.K. Cavernous sinus and inferior petrosal sinus flow signal on three-dimensional time-of-flight MR angiography. AJNR Am J Neuroradiol. 1999;20:1476-1481.
Parker D.L., Tsuruda J.S., Goodrich K.C., et al. Contrast-enhanced magnetic resonance angiography of cerebral arteries. A review. Invest Radiol. 1998;33:560-572.
Parmar H., Ivancevic M.K., Dudek N., et al. Neuroradiologic applications of dynamic MR angiography at 3 T. Magn Reson Imaging Clin N Am. 2009;17:63-75.
Polak J.F. Carotid IMT: a surrogate marker for cerebrovascular disease? Semin Cerebrovascular Dis Stroke. 2005;5:66-73.
Pruessmann K.P., Weiger M., Scheidegger M.B., et al. SENSE: sensitivity encoding for fast MRI. Magn Reson Med. 1999;42:952-962.
Puetz V., Dzialowski I., Hill M.D., et al. Intracranial thrombus extent predicts clinical outcome, final infarct size and hemorrhagic transformation in ischemic stroke: the Clot Burden Score. Int J Stroke. 2008;3:230-236.
Puetz V., Sylaja P.N., Hill M.D., et al. CT angiography source images predict final infarct extent in patients with basilar artery occlusion. AJNR Am J Neuroradiol. 2009;30:1877-1883.
Puetz V., Dzialowski I., Hill M.D., et al. Malignant profile detected by CT angiographic information predicts poor prognosis despite thrombolysis within three hours from symptom onset. Cerebrovasc Dis. 2010;29:584-591.
Pugliese F., Crusco F., Cardaioli G., et al. CT angiography versus colour-Doppler US in acute dissection of the vertebral artery. Radiol Med. 2007;112:435-443.
Qureshi A.I., Alexandrov A.V., Tegeler C.H., et al. Guidelines for screening of extracranial carotid artery disease: a statement for healthcare professionals from the Multidisciplinary Practice Guidelines Committee of the American Society of Neuroimaging. J Neuroimaging. 2007;17:19-47.
Randoux B., Marro B., Koskas F., et al. Carotid artery stenosis: prospective comparison of CT, three-dimensional gadolinium-enhanced MR, and conventional angiography. Radiology. 2001;220:179-185.
Remonda L., Senn P., Barth A., et al. Contrast-enhanced 3D MR angiography of the carotid artery: comparison with conventional digital subtraction angiography. AJNR Am J Neuroradiol. 2002;23:213-219.
Ritter M.A., Dittrich R., Thoenissen N., et al. Prevalence and prognostic impact of microembolic signals in arterial sources of embolism. A systematic review of the literature. J Neurol. 2008;255:953-961.
Rubiera M., Alexandrov A.V. Sonothrombolysis in the management of acute ischemic stroke. Am J Cardiovasc Drugs. 2010;10:5-10.
Saba L., Caddeo G., Sanfilippo R., et al. CT and ultrasound in the study of ulcerated carotid plaque compared with surgical results: potentialities and advantages of multidetector row CT angiography. AJNR Am J Neuroradiol. 2007;28:1061-1066.
Saraf-Lavi E., Bowen B.C., Quencer R.M., et al. Detection of spinal dural arteriovenous fistula with MR imaging and angiography: sensitivity, specificity, and prediction of vertebral level. AJNR Am J Neuroradiol. 2002;23:858-867.
Schramm P., Schellinger P.D., Fiebach J.B., et al. Comparison of CT and CT angiography source images with diffusion-weighted imaging in patients with acute stroke within 6 hours after onset. Stroke. 2002;33:2426-2432.
Sharma D., Souter M.J., Moore A.E., et al. Clinical experience with transcranial doppler ultrasonography as a confirmatory test for brain death: a retrospective analysis. Neurocrit Care. 2010;14:370-376.
Sheehy N., MacNally S., Smith C.S., et al. Contrast-enhanced MR angiography of subclavian steal syndrome: value of the 2D time-of-flight “localizer” sign. AJR Am J Roentgenol. 2005;185:1069-1073.
Shellock F.G. Biomedical implants and devices: assessment of magnetic field interactions with a 3.0-Tesla MR system. J Magn Reson Imaging. 2002;16:721-732.
Shrier D.A., Tanaka H., Numaguchi Y., et al. CT angiography in the evaluation of acute stroke. AJNR Am J Neuroradiol. 1997;18:1011-1020.
Skjelland M., Krohg-Sorensen K., Tennoe B., et al. Cerebral microemboli and brain injury during carotid artery endarterectomy and stenting. Stroke. 2009;40:230-234.
Sitzer M., Siebler M., Steinmetz H. Noninvasive evaluation of internal carotid stenosis with color Doppler assisted duplex imaging. Clin Radiol. 1996;51(suppl. 1):24-27.
Sloan M., Wozniak M.A., Macko R.F. Transcranial Doppler monitoring of vasospasm after subarachnoid hemorrhage. In: Babikian V.L., Wechsler L.R. Transcranial Doppler Ultrasonography. second ed. Boston: Butterworth-Heinemann; 1999:109-127.
Stock K.W., Radue E.W., Jacob A.L., et al. Intracranial arteries: prospective blinded comparative study of MR angiography and DSA in 50 patients. Radiology. 1995;195:451-456.
Stolz E., Nuckel M., Medes I., et al. Vertebrobasilar transcranial color-coded duplex ultrasonography: improvement with echo enhancement. AJNR Am J Neuroradiol. 2002;23:1051-1054.
Suwanwela N.C., Phanthumchinda K., Suwanwela N. Transcranial Doppler sonography and CT angiography in patients with atherothrombotic middle cerebral artery stroke. AJNR Am J Neuroradiol. 2002;23:1352-1355.
Tan T.Y., Schminke U., Lien L.M., et al. Extracranial internal carotid artery occlusion: the role of common carotid artery volume flow. J Neuroimaging. 2002;12:144-147.
Tegeler C.H., Ratanakorn D. Neurosonology. In: Bogousslavky J., Fisher M. Textbook of Neurology. Boston: Butterworth-Heinemann; 1998:101-118.
Tegeler C.H., Ratanakorn D. Ultrasound and cerebrovascular disease. In: Toole J.F., editor. Cerebrovascular Disorders. fifth ed. Philadelphia: Lippincott Williams & Wilkins; 1999:83-128.
Tegeler C.H., Ratanakorn D., Kim J. Advances in carotid ultrasound. Semin Cerebrovascular Dis Stroke. 2005;5:74-82.
Torres-Mozqueda F., He J., Yeh I.B., et al. An acute ischemic stroke classification instrument that includes CT or MR angiography: the Boston Acute Stroke Imaging Scale. AJNR Am J Neuroradiol. 2008;29:1111-1117.
Tsivgoulis G., Eggers J., Ribo M., et al. Safety and efficacy of ultrasound-enhanced thrombolysis: a comprehensive review and meta-analysis of randomized and nonrandomized studies. Stroke. 2010;41:280-287.
Turski P.A., Korosec F.R., Carroll T.J., et al. Contrast-enhanced magnetic resonance angiography of the carotid bifurcation using the time-resolved imaging of contrast kinetics (TRICKS) technique. Top Magn Reson Imaging. 2001;12:175-181.
Unsgaard G., Gronningsaeter A., Ommedal S., et al. Brain operations guided by real-time two-dimensional ultrasound: new possibilities as a result of improved image quality. Neurosurgery. 2002;51:402-412.
van der Schaaf I.C., Velthuis B.K., Wermer M.J., et al. Multislice computed tomography angiography screening for new aneurysms in patients with previously clip-treated intracranial aneurysms: Feasibility, positive predictive value, and interobserver agreement. J Neurosurg. 2006;105:682-688.
Vertinsky A.T., Schwartz N.E., Fischbein N.J., et al. Comparison of multidetector CT angiography and MR imaging of cervical artery dissection. AJNR Am J Neuroradiol. 2008;29:1753-1760.
Villablanca J.P., Jahan R., Hooshi P., et al. Detection and characterization of very small cerebral aneurysms by using 2D and 3D helical CT angiography. AJNR Am J Neuroradiol. 2002;23:1187-1198.
Wada R., Aviv R.I., Fox A.J., et al. CT angiography “spot sign” predicts hematoma expansion in acute intracerebral hemorrhage. Stroke. 2007;38:1257-1262.
Wardlaw J.M., Chappell F.M., Best J.J., et al. Non-invasive imaging compared with intra-arterial angiography in the diagnosis of symptomatic carotid stenosis: a meta-analysis. Lancet. 2006;367:1503-1512.
White P.M., Teasdale E.M., Wardlaw J.M., et al. Intracranial aneurysms: CT angiography and MR angiography for detection: prospective blinded comparison in a large patient cohort. Radiology. 2001;219:739-749.
White P.M., Wardlaw J.M., Easton V. Can noninvasive imaging accurately depict intracranial aneurysms? A systematic review. Radiology. 2000;217:361-370.
Wiebers D.O., Whisnant J.P., Huston J.III, et al. International Study of Unruptured Intracranial Aneurysms Investigators. Unruptured intracranial aneurysms: natural history, clinical outcome, and risks of surgical and endovascular treatment. Lancet. 2003;362:103-110.
Willig D.S., Turski P.A., Frayne R., et al. Contrast-enhanced 3D MR DSA of the carotid artery bifurcation: preliminary study of comparison with unenhanced 2D and 3D time-of-flight MR angiography. Radiology. 1998;208:447-451.
Willinek W.A., Hadizadeh D.R., von Falkenhausen M., et al. 4D time-resolved MR angiography with keyhole (4D-TRAK): more than 60 times accelerated MRA using a combination of CENTRA, keyhole, and SENSE at 3.0T. J Magn Reson Imaging. 2008;27:1455-1460.
Willoteaux S., Faivre-Pierret M., Moranne O., et al. Fibromuscular dysplasia of the main renal arteries: comparison of contrast-enhanced MR angiography with digital subtraction angiography. Radiology. 2006;241:922-929.
Wutke R., Lang W., Fellner C., et al. High-resolution, contrast-enhanced magnetic resonance angiography with elliptical centric k-space ordering of supra-aortic arteries compared with selective X-ray angiography. Stroke. 2002;33:1522-1529.
Yamada N., Takamiya M., Kuribayashi S., et al. MRA of the Adamkiewicz artery: a preoperative study for thoracic aortic aneurysm. J Comput Assist Tomogr. 2000;24:362-368.
Yasaka M., O’Keefe G.J., Chambers B.R., et al. Streptokinase in acute stroke. Neurology. 1998;50:626-632.
Yoshioka K., Niinuma H., Ohira A., et al. MR angiography and CT angiography of the artery of Adamkiewicz: noninvasive preoperative assessment of thoracoabdominal aortic aneurysm. Radiographics. 2003;23:1215-1225.