CHAPTER 17 Neonatology for Anesthesiologists
Advances in obstetrics and neonatology have greatly improved neonatal survival, especially for preterm neonates (Fig. 17-1). Because the survival rate of premature infants is on the rise, so is the need for anesthesia and surgery for these patients. To safely care for newborns in the operating room, anesthesiologists must appreciate and understand the enormous physiologic variability that exists among infants born between 23 and 44 weeks of gestation, so they can better understand how immature organs respond to surgery and anesthetic agents. Fetal development is also relevant, because fetal surgery is a reality in many medical centers, and providing anesthesia for midgestation fetuses (20 to 23 weeks’ gestation) via the mother poses a completely new set of challenges (Hirose et al., 2004). The purpose of this chapter is to present background information that will allow anesthesiologists to better understand the problems of neonates and to provide safe care for these patients based on an understanding of neonatal physiology. The preoperative evaluation of the neonate is arguably the most important part of anesthetic care.
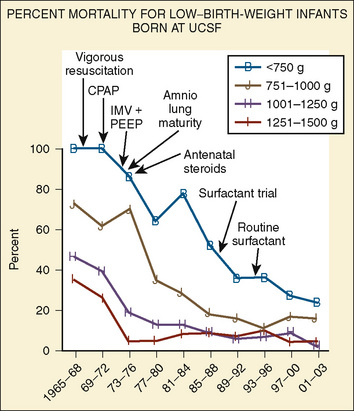
FIGURE 17-1 Percent mortality for low–birth-weight infants born at University of California, San Francisco.
Although some congenital anomalies are obvious at birth, others, such as gastrointestinal dysfunction, various central nervous system (CNS) malformations, or metabolic disorders, may not develop until later in life. In general, if one congenital anomaly is present, the anesthesiologist must be suspicious that there are others. Box 17-1 provides a list of disease entities and coexisting anomalies, and this list may be useful as part of a preoperative assessment (Jones and Pelton, 1976).
Development Of the Fetus and the Intrauterine Environment
The intrauterine environment has dramatic effects on growth and on the ability of neonates to adapt to extrauterine life. Although the impact of intrauterine events is most obvious during the first few hours and days of life, the effects of these events can last much longer. For example, phenytoin, thalidomide, alcohol, and maternal drug use has caused well-described syndromes, alterations in somatic growth, or drug-dependence and withdrawal (e.g., opioids or amphetamines). Exposure to drugs during pregnancy has been associated with anomalies of the heart, pulmonary circulation, brain, and other organs (e.g., cleft lip and palate) (Puhó et al., 2007).
However, the fetus is at a great disadvantage—it has no store of oxygen beyond that in its blood, because the lungs are filled with fluid and not gas (Dudenhausen et al., 1997). After only a minute of umbilical cord occlusion, the fetus’ Pao2 is less than 5 mm Hg. At the same time, the Paco2 steadily rises and the pH rapidly declines. The effects of intrauterine asphyxia can be sudden and profound, and they are often associated with severe hypoxia, hypotension, and acidosis (Fig. 17-2).
Labor and Delivery and Perinatal Events
The neonate’s respiratory rate increases briefly with the onset of asphyxia (see Fig. 17-2). Primary apnea quickly follows and lasts a variable amount of time. This is followed by slow gasping respirations and then by terminal apnea and death unless resuscitation is begun (Dawes, 1968). The heart rate and arterial blood pressure increase moderately, and bradycardia and hypotension follow. Because Cardiac Output (CO) is mostly dependent on heart rate in neonates, the severe, deep reduction in heart rate associated with asphyxia significantly reduces CO (Klopfenstein and Rudolph, 1978). To compensate for this reduction, blood flow is centralized to maintain blood flow and oxygen delivery to the brain, heart, and adrenal glands. The arterial blood pressure increases during gasping respirations and decreases markedly during terminal apnea. Heart rate, CO, and arterial blood pressure quickly improve with resuscitation. However, the metabolic component of acidosis may worsen as the pHa is corrected, because fixed acids are washed out of the periphery by the improved peripheral perfusion. If the pH does not improve with ventilation alone, an infusion of tromethamine (Tham) or sodium bicarbonate may be necessary. The lungs should be ventilated if sodium bicarbonate is administered, because each milliequivalent of sodium bicarbonate (when fully reacted with hydrogen ion) produces 25 cc of CO2 that must be removed via the lungs.
Labor and Delivery
Labor, fetal movements, and uterine contractions initiate removal of the 30 mL/kg of fluid that are normally present in the fetal lungs (Harding and Hooper, 1996). During labor, compression of the fetal chest by the uterus and vagina removes some of the lung fluid (Harding et al., 1990). After birth, breathing increases clearance of the lung fluid via the pulmonary capillaries and the lymphatics (see Pulmonary System, p. 518). The specific mechanism that initiates breathing at birth remains unclear, but several factors are known to play critical roles. First, placental factors that inhibit breathing in utero are removed by clamping the umbilical cord (Alvaro et al., 2004, 1997). Second, at birth the sudden release of the circumferential pressure around the chest allows the neonatal chest to recoil outward and draw gas into the lungs (Karlberg et al., 1962). Third, tactile stimulation and environmental thermal changes at birth stimulate breathing (Sinha and Donn, 2006). Lastly, the effects of hypoxia and hypercarbia on the chemosensors stimulate breathing (Taeusch et al., 2005).
Neonatal Asphyxia
Postnatal asphyxia is often the result of a continuum of intrauterine events, but it may also be caused by events that occur during labor and delivery. Immature respiratory control mechanisms can predispose neonates, especially premature neonates, to life-threatening responses to asphyxia. For example, the response to hypoxia during the first 3 to 4 weeks of life can be paradoxical, in that hypoxia produces a brief period of hyperpnea that is followed by bradypnea (Cross and Oppe, 1952; Brady and Ceruti, 1966). Hypothermia and hypercapnea blunt the initial hyperpnea (Ceruti, 1966; Rigatto et al., 1975). The ventilatory response to carbon dioxide increases with both postnatal and gestational age (see Chapter 3, Respiratory Physiology in Infants and Children) (Rigatto et al., 1975).
Although hypoxia may inflict long-term consequences on the fetus and newborn, hyperoxia can also cause significant morbidity, especially for preterm infants. For example, hyperoxia exposes preterm infants, especially those born before 32 weeks’ gestation, to a significant risk for retinopathy of prematurity (ROP; see below) and, in some cases, blindness (see Chapter 27, Anesthesia for Ophthalmic Surgery) (Sylvester, 2008). The normal Pao2 of a fetus is 20 to 30 mm Hg. After birth, a Pao2 of 60 mm Hg is probably hyperoxic for infants born at 24 to 36 weeks’ gestation. To avoid the effects of oxidative stress in the newborn, the oxygen saturation for premature infants is usually maintained between 88% and 93% (Pao2 of 45 to 60 mm Hg) in the NICU, and similar Sao2 levels are appropriate in the operating room. Continuous measurement of Sao2 makes it easier to maintain the desired oxygen saturation. Of note is one preterm infant, who never had an elevated Pao2 except in the operating room, but who developed ROP after surgery (Betts et al., 1977).
Metabolic Status: Early Postnatal Period
Thermal Environment
In a neutral thermal environment, neonates expend the least amount of energy to maintain their body temperature. The oxygen consumption (o2) of normal neonates is 6 cc/kg per minute, twice that of resting adults. During the first week of life,
o2 increases to about 10 cc/kg per minute and may be twice that during heat-related stress. Because of their high oxygen consumption, greater CO, and smaller reserve of oxygen (i.e., smaller functional residual capacity [FRC]), neonates, especially preterm neonates, develop hypoxia more quickly than adults. In fact, hypoxia may develop in as few as 10 seconds in apneic neonates, whereas it takes several minutes for this to occur in adults (Peabody et al., 1978a, 1978b).
Sodium, Calcium, and Glucose
Box 17-2 contains common fluid and electrolyte requirements for neonatal infants. Because premature, large-for-gestational age (LGA), small-for-gestational-age (SGA), and asphyxiated neonates are often hyper- or hypoglycemic, intravenous glucose may be required to maintain normoglycemia (serum glucose concentration of 40 to 90 mg/dL; see Glucose Metabolism, p. 537) (Jain et al., 2008). Similarly, calcium homeostasis is often difficult to achieve in sick infants (Bass and Chan, 2006). Initial calcium administration is usually based on normal neonatal requirements and on need, as determined by serial ionized calcium measurements. Calcium should be infused into a well-functioning intravenous catheter to avoid extravasation of calcium into the tissues and severe tissue necrosis. During surgery the calcium infusion site is usually under the surgical drapes and hard to see. Therefore, calcium should be infused into a reliable intravenous catheter, preferably a central venous catheter.
Box 17-2 Common Intravenous Fluid and Electrolyte Requirements in the Newborn
Sodium
In full-term and near-term infants, sodium is seldom added to intravenous fluids during the first 24 hours of life. Urine output is low, despite the fact that 30 mL/kg of fluid must be removed from the lungs during and after birth (Herin and Zetterström, 1994). On the second day of life, maintenance sodium (2 to 4 mEq/kg per day) is added to the intravenous fluids to replace renal and gastrointestinal tract sodium losses, to compensate for abnormal fluid metabolism, or to counter the effect of diuretics (e.g., furosemide). In the extremely low-birth weight (ELBW) infant, fluid that contains sodium is often administered to maintain adequate intravascular volume, especially when transcutaneous fluid losses are excessive. After the first few days of life, an adequate sodium intake is essential for infants of all gestational ages to permit normal growth.
Gestational Age
The SGA Infant
In part, perinatal problems are related to gestational age and birth weight (Box 17-3). Infants who weigh less than the 10th percentile of weight for gestational age at birth are usually considered to be small for their gestational age. SGA infants have different problems from those of preterm infants (fewer than 37 weeks’ gestation) of the same weight (Lubchenco et al., 1963). Consequently, it is necessary to relate birth weight to gestational age. Although primary fetal (e.g., chromosomal disorders) and maternal (e.g., smoking or chronic disease) factors have been linked to intrauterine growth retardation, SGA is often the result of placental insufficiency. SGA newborns are often hypoglycemic because they have abnormally low glycogen stores. They commonly respond to placental insufficiency by increasing their red-blood-cell mass. Although an increase in red-blood-cell mass (polycythemia) increases the oxygen-carrying capacity of blood, polycythemia also has been associated with hyperviscosity, as well as renal failure, NEC, and CNS injury, especially if the hematocrit value exceeds 65% (Pallotto and Kilbride, 2006; Rosenberg, 2008). If the hematocrit value is higher than 65% in well-hydrated neonates, an exchange transfusion should be considered to reduce the hematocrit level to 55% or lower before surgery, although there is no evidence that doing so for patients who are not having surgery improves outcome (Dempsey and Barrington, 2006). However, the combination of polycythemia, hypotension, and dehydration that are common in sick SGA infants during anesthesia and surgery may increase their risk for hyperviscosity-related problems. Arterial blood pressure should be kept normal or slightly elevated throughout surgery. SGA infants may be relatively dehydrated at birth and usually do not lose the normal 200 to 300 g of weight that appropriate-for-gestational-age (AGA) neonates lose over the first 2 to 3 days of life. SGA neonates usually gain weight from birth. In spite of their low birth weights, the heart rates and blood pressures of SGA infants are similar to those of AGA infants of similar gestational ages. SGA neonates have similar incidences of hyaline membrane disease (HMD) to AGA babies of the same gestational age.
The LGA Infant
LGA infants are prone to birth injuries (e.g., fractures or intracranial bleeds) (Lawrence, 2007). Those born to diabetic mothers (a common reason for LGA) may have difficulty maintaining normal blood glucose concentrations and glucagon may be required to do so (Van Howe and Storms, 2008). On occasion, tracheal intubation may be difficult in LGA babies because of fat deposits in their mouth, tongue, and neck. Finding adequate insertion sites for intravenous fluid may be difficult because the veins are buried in tissue. It may be necessary to insert a central venous catheter or an external jugular vein catheter to ensure adequate intravenous access.
The Premature Infant
Prematurity is defined as birth before 37 weeks of gestation. Premature infants account for 12.8% of all births in the United States (646,047 of 4,265,996), and the incidence has increased 21% since 1990 (Martin et al., 2008). The increase has been most dramatic between the gestational ages of 34 to 36 weeks (Davidoff et al., 2006). While the rates of VLBW (fewer than 1500 g) and ELBW (fewer than 1000 g) deliveries remained relatively unchanged from 2005 to 2006, the percentage of moderately low birth weight (1500 to 2499 g) deliveries increased slightly. In 2005, short gestation and low birth weight was the second leading cause of infant death (16.5%), ranked only below congenital malformations (19.5%) (Martin et al., 2008). However, in non-Hispanic black, and Puerto Rican infants, disorders related to short gestation and low birth weight were the leading causes of death. Today, the Centers for Disease Control (CDC) recognize prematurity, not birth defects, as the leading cause of infant mortality in the United States (Andrews et al., 2008).
Late Preterm (35 to 37 Weeks’ Gestation)
In the absence of congenital anomalies or perinatal asphyxia, infants born between 35 and 37 weeks’ gestation usually do well and have few of the common medical or surgical problems found in more premature infants. However, late preterm birth is associated with increased mortality (threefold higher than term infants), respiratory distress or apnea requiring support, and rehospitalization (Escobar et al., 2006; Tomashek et al., 2007). In addition, sepsis and intrapartum complications, centered on the placenta and cord, have contributed to increased mortality in late preterm infants (Tomashek et al., 2007). In addition, late-preterm infants may have some difficulty attaining full feeds, and the incidence of hyperbilirubinemia (not associated with Coombs’ positivity or ABO/Rh incompatibility) occurs more commonly in late preterm infants than in term infants. Late-preterm infants born to diabetic mothers may have pulmonary immaturity and respiratory distress syndrome (RDS) (Deorari et al., 1991). Late-preterm infants born to nondiabetic mothers usually have similar lung function to that of term infants. Most surgical intervention in this group of patients is for treatment of congenital anomalies.
The rate of late preterm birth has increased 25% since 1990, and attention has been drawn to the increased mortality associated with late-preterm compared with term gestation (Escobar et al., 2006; Shapiro-Mendoza et al., 2006; Engle et al., 2007; Tomashek et al., 2007; Martin et al., 2008; McIntire and Leveno, 2008). The morbidity and mortality rates of late-preterm infants are particularly noticeable because of the large number of births at this developmental stage; in 2006, late-preterm births accounted for more than 71% of all preterm births (9.14%) (Martin et al., 2008). However, the long-term outcome of this group has not been well documented, in part related to the longstanding view of this group as “near term” rather than late preterm (Jain, 2007).
30 to 34 Weeks’ Gestation
Before the introduction of exogenous pulmonary surfactant into clinical practice, this group of infants often had RDS, also known as HMD (see Respiratory Distress Syndrome, p. 520). Complications of their respiratory care, including pneumothorax, pulmonary interstitial emphysema, chronic lung disease (CLD), and blood loss from repeated blood sampling, were common. Treatment of RDS with surfactant has dramatically reduced the incidence and severity of this disease and it complications. The sequelae of pulmonary immaturity and of the supportive care used to treat lung immaturity are fewer and less severe since the introduction of exogenous surface-active material (SAM). However, a new form of bronchopulmonary dysplasia (BPD), called new BPD, occurs in small preterm infants (Bancalari, 2001; Bancalari and del Moral, 2001). Approximately 20% to 25% of these infants have some form of CLD.
Although uncommon in infants after 34 weeks’ gestation, newborns with a gestational age of 30 to 32 weeks often demonstrate temperature instability, especially if they are septic or asphyxiated. Feeding intolerance is also common, and it may take weeks before effective oral feeding and consistent weight gain occur. Before adequate enteral feeds are established, infants born at 30 to 34 weeks’ gestation are often hypocalcemic or hypoglycemic. They are also prone to NEC, especially if they have a large patent ductus arteriosus (PDA) with significant left-to-right shunting of blood (Kitterman, 1980). Because enteral feeds must be advanced slowly, peripheral or central intravenous alimentation may be required for some time. Parenteral nutrition, for even 1 to 2 weeks, can cause hepatic and renal toxicity (Moreno Villares, 2008).
The incidence of a PDA is high (20% to 30%) in infants of this gestational age, but the hemodynamic consequences are often mild. Their PDA commonly closes spontaneously. If it does not close, chemical (with indomethacin) or surgical closure of the PDA may be necessary. The incidence of intracerebral hemorrhage (ICH) is higher in this age group and increases with decreasing gestational age, sepsis, asphyxia, or precipitous birth. Apnea is also more common at this developmental stage, especially in those infants who have sepsis, temperature instability, metabolic abnormalities (e.g., hypoglycemia or hypocalcemia), or anemia (Theobald et al., 2000).
Fewer than 26 Weeks’ Gestation
In this group of patients, the CNS is fragile, and ICH is common (Kadri et al., 2006). The severity of ICH is a common determinant of an infant’s prognosis and long-term outcome, and it correlates with later neurologic injury (see The Central Nervous System, p. 528). Apnea and perioperative pulmonary insufficiency occur often and may persist for months. From the first few weeks to the first months of life, most infants of this developmental stage require mechanical ventilation, continuous positive airway pressure (CPAP), or an increased inspired oxygen concentration after surgery. Ventilatory support is often required, even if these interventions were not necessary preoperatively. Prolonged tracheal intubation is occasionally required and may lead to subglottic stenosis and lower airway obstruction.
Review of Systems and Developmental Physiology
Head and Neck
Anatomic differences of the head and neck between neonates and older patients make the management of the neonate’s airway challenging (Dickison, 1987). The cricoid ring is the narrowest portion of the neonate’s upper airway because the larynx has been thought to be cone shaped, rather than cylindrical as it is after 5 years of age (Coté et al., 1993). However, this concept has been challenged (Litman et al., 2003; Dalal et al., 2009; Motoyama, 2009). These newer studies indicate that the shape of the larynx is cylindrical, as it is in adults, but the cricoid ring remains the fixed and narrowest point of the upper airway system.
The narrowest portion of the cone is the cricoid ring. This is, in part, because the neonate’s posterior larynx is more cephalad than the anterior larynx. When looking from above, the cricoid ring is really an ellipse and not a circle. By 5 years of age, the posterior larynx descends, the cricoid ring is circular, and the vocal cords are the narrowest portion of the airway (as they are in adults). These anatomic differences place the neonatal larynx in a more cephalad position than it is later in life. The epiglottis is relatively large, which makes it more difficult to lift with a laryngoscope blade. In fact it is easier to use a straight laryngoscope blade like a curved blade, place the tip in the vellecula, and pull up and out at a 45-degree angle to bring the glottic opening into view. The cephalad larynx and relatively large tongue also make it more difficult to maintain an airway or to intubate the trachea. However, the neonate’s large occiput naturally places the head (when looking straight forward) in the “sniffing” position (i.e., an optimal position to ventilate the lungs and intubate the trachea). Extension or flexion of the head usually makes it more difficult to bag-and-mask ventilate the lungs and to intubate the trachea. A small jaw (micrognathia) or receding jaw (e.g., Pierre-Robin or Treacher-Collins syndromes) may make it difficult or impossible to directly visualize the vocal cords. A large tongue (as with hypothyroidism, Down syndrome, or Beckwith-Wiedemann syndrome) can also make tracheal intubation difficult or impossible. Techniques other than direct laryngoscopy may be needed to intubate the trachea of these infants (see Chapter 12, Airway Management).
Cleft lip, with and without a cleft palate, occurs in 1:600 to 1000 live births and is associated with other congenital defects in 13% to 50% of patients (see Chapter 25, Anesthesia for Plastic Surgery) (Arosarena, 2007). Tracheal intubation is occasionally more difficult in patients with a cleft palate because it may be difficult to fix the patient’s tongue against the palate with the laryngoscope blade. If the tongue cannot be fixed against the palate, it may flop over the laryngoscope blade and block the view of the glottis. Choanal atresia occurs in 1:7000 to 1:8000 live births and is often diagnosed in the delivery room when a catheter cannot be passed through a nostril into the pharynx or when the neonate cannot breath and becomes cyanotic and bradycardic when the mouth is closed and the airway is totally obstructed (see Chapter 12, Airway Management) (Samadi et al., 2003). Thus, when using bag-and-mask ventilation on patients who have choanal atresia, the mouth must be kept open or an oral airway must be inserted. Some patients who have choanal atresia have the CHARGE association, which is defined through the anacronym as follows: C, Colobomatous malformation; H, Heart defect; A, Atresia choane; R, Retardation; G, Growth deficiency/Genital hypoplasia; and E, Ear anomalies (Sanlaville and Verloes, 2007).
The Pulmonary System
Development
Lung development is divided into the following five stages:
The latter stage begins at about 36 weeks’ gestation and continues until at least 18 months of age (Langston et al., 1984; Kotecha, 2000a, 2000b; Joshi and Kotecha, 2007). Knowledge of these phases of lung development allows estimation of when the various lung malformations occur in utero. For example, malformations of the conducting airways (e.g., cystic adenomatoid malformation) occur before 16 weeks’ gestation. Upper airway abnormalities occur by 6 weeks’ gestation, bronchial malformations between 6 and 16 weeks of gestation, and lung hypoplasia after 16 weeks’ gestation. In general, extrauterine viability is increased after 26 weeks’ gestation, because the respiratory saccules have developed and the capillaries are in close approximation to the developing distal airways. Before this time, both the vascular network and surface area in the lungs may be inadequate for gas exchange. However, in the past decade many babies have survived after 24 to 25 weeks’ gestation, usually with enormous effort and cost; these extremely premature infants often survive with severe CLD (Langston et al., 1984).
Most alveoli develop after birth, increasing from 20 million terminal air sacs at term to about 300 to 400 million alveoli at 18 months of age (Thurlbeck, 1982; Kotecha, 2000a, 2000b). Specific cell types are not recognizable in the lung until the canalicular stage of development (17 to 28 weeks’ gestation). During the last 10% to 20% of gestation, type II pneumocytes are more commonly identified. Although present in the human fetus by 22 weeks’ gestation, type II pneumocytes are more prominent after 34 to 36 weeks of gestation. The major distinguishing feature of type II cells is the presence of osmophilic lamellar bodies, which correlate closely with the presence of SAM in the lungs (Kotecha, 2000a, 2000b). The alveolar-capillary barrier is formed by type I pneumocytes (see Chapter 3, Respiratory Physiology in Infants and Children).
By 16 weeks’ gestation, all subdivisions of the conducting airways have formed, including the main-stem bronchi and the conducting and terminal bronchioles. Preterm infants often require mechanical ventilation or CPAP and supplemental oxygen, which interfere with the normal, complex process of lung and airway development. The barotrauma, oxidative stress, and inflammatory injury associated with these life-saving interventions and the effects of superimposed infections predispose preterm infants to airway and lung injury and to abnormal airway resistance and reactivity (Van Marter, 2005).
The diaphragm arises in part from the third through fifth cervical somites, and myoblasts from these somites migrate into the septum transversum to form diaphragmatic muscle (Mitchell and Sharma, 2009). The central tendon of the diaphragm arises from the mesoderm of the septum transversum. Somites of the lateral chest wall form the lateral portions of the diaphragm. The nerve supply of the diaphragm is from the third through fifth cervical somites. Bilateral pericardioperitoneal canals, located lateral to the developing diaphragm, cause a Bochdalek hernia if the canals fail to close. Complete closure of the diaphragm normally occurs at 10 to 12 weeks’ gestation, when the bowel is returning to the abdominal cavity from the amnion, where it resides in early gestation. If the diaphragm is incompletely formed, the returning bowel follows the path of least resistance and enters the chest. When this occurs lung growth ceases on the ipsilateral side. The mediastinum is then pushed into the opposite chest by the bowel and abdominal organs and contralateral lung growth is also reduced. Consequently, neonates who have a left-sided diaphragmatic hernia must survive with approximately two thirds of one lung or less (de Lorimier et al., 1967). In some cases, the amount of lung present is insufficient for survival (see Chapter 18, Anesthesia for General Surgery in the Neonate).
Failure of the lung buds to separate from the foregut causes a tracheal esophageal fistula (see Chapter 18, Anesthesia for General Surgery in the Neonate). The connection usually is found between the distal esophagus and the trachea, just above the carina. Failure of the distal and proximal ends of the esophagus to connect with each other results in esophageal atresia. Patients with esophageal atresia usually have copious secretions in the proximal esophageal pouch, and placing the patient flat without removing the secretions may cause aspiration of the secretions. Placing a suction catheter in the proximal esophageal pouch and connecting it to suction reduces this likelihood.
Early Postnatal Period: Changes in Oxygenation and Pulmonary Vascular Resistance
Although removal of fluid from the fetal lung begins during labor and delivery, the major transition to an air-filled lung occurs immediately after birth. The first active inspiration after birth produces a negative intrapleural pressure of 80 to 100 cm H2O, which is required to overcome the high surface tension, low compliance, and high resistance of the liquid-filled lungs (Scarpelli and Mautone, 1984; Vyas et al., 2005). These pressures are not necessary when artificially ventilating neonatal lungs, however. In fact, delivering large tidal volumes and high airway pressures injure neonatal lungs (Bjorklund et al., 1997). In full-term neonates, the initial few spontaneous breaths are of 20 and 80 cc O2 each. A small fraction of this volume is expired. The gas remaining in the lung forms the residual volume (RV) and the FRC, which are required for adequate gas exchange (Avery, 1974). If removal of lung fluid is delayed (e.g., with cesarean section) transient tachypnea of the newborn (TTN) develops (Guglani et al., 2008). Neonates with TTN breathe 60 to 150 times a minute and are often hypoxic and hypercarbic. Many of them require supplemental oxygen, and some require mechanical ventilation. Diuretics fail to induce a diuresis and improve lung function until a spontaneous diuresis occurs several days after birth (Lewis and Whitelaw, 2002).
Labor reduces pulmonary vascular resistance (PVR) by approximately 10%, but the most significant decrease occurs with the onset of breathing (Bland, 1983). Lung expansion increases alveolar and arterial Po2, which dilates the pulmonary arterioles, decreases PVR and increases pulmonary blood flow (Dawes, 1974). These changes in PVR and blood flow are critical for conversion of the fetal circulation to the adult form of circulation (see Chapters 3, Respiratory Physiology, and 4, Cardiovascular Physiology). That is, transition to the gas-filled lung at birth, increased Fio2 (air), and increased pH (decreased Paco2) all interact to reduce PVR and increase pulmonary blood flow. Over the next 1 to 2 months, PVR decreases to adult levels. However, infants with cyanotic congenital heart disease (CHD) or large left-to-right shunts may have elevated PVR for months or longer (Rudolph, 2007).
The dramatic rise in pulmonary blood flow at birth increases the left atrial pressure and functionally closes the foramen ovale, preventing right-to-left shunting of deoxygenated blood through this structure. An increase in systemic vascular resistance that exceeds PVR dramatically reduces right-to-left blood flow through the ductus arteriosus. The rise in arterial oxygen tension at birth constricts and physiologically closes the ductus arteriosus in full-term but not in preterm neonates (Clyman et al., 1978). Nitric oxide (NO) and prostaglandins are important for anatomic closure of the ductus arteriosus, which usually occurs by 2 to 3 weeks of age (Born et al., 1956; Seidner et al., 2001; Clyman, 2006). If during this time hypoxia, acidosis, cold, or excessive airway pressures are present, the right atrial pressure increases and right-to-left shunting of blood can occur at both the atrial and the DA levels. When right-to-left shunting of blood occurs, hypoxemia ensues. The resulting pulmonary blood flow pattern mimics the pattern present in utero and is often referred to as persistent fetal circulation (PFC).
Establishing normal pulmonary blood flow and ventilation increases both arterial and mixed venous oxygen tensions. Although the transition to air breathing increases the oxygen content of blood, the Pao2 of the neonate is usually 55 to 85 mm Hg for the first month of life, rather than the 95 to 100 mm Hg present after that. The lower Pao2 is the result of a very compliant, cartilaginous neonatal chest wall that does not recoil outward at end-expiration, as it does in older children and adults (Koch and Wendel, 1968; Davis and Bureau, 1987). Failure to recoil outward produces a transpulmonary (intrapleural) pressure of 0 cm H2O—not −5 cm H2O pressure, as occurs in older patients. Because negative intrapleural pressures are important for maintenance of a normal FRC, neonates are predisposed to atelectasis and a lower Pao2. In fact, application of sufficient negative pressure around the neonate’s chest to produce a pleural pressure of −5 cm H2O, increases the Pao2 from about 65 mm Hg to nearly 100 mm Hg, indicating that the negative intrapleural pressure expands the lungs, increases FRC, reduces atelectasis, and improves the match of ventilation to perfusion (Thibeault et al., 1968). Application of negative intrapleural pressure, positive end expiratory pressure (PEEP), or CPAP also reduces the amount of atelectasis and improves oxygenation (Gregory et al., 1975).
In addition to its effects on transpulmonary pressure, the newborn’s compliant rib cage has another unwanted effect on ventilation. That is, the cartilaginous, compliant chest wall tends to collapse inward (retract) during spontaneous inspiration, causing paradoxic chest-wall motion and limiting airflow (Knill et al., 1976). The circular configuration of the rib cage (rather than the ellipsoid rib cage of adults) and the horizontal angle of insertion of the diaphragm (rather than the oblique angle in adults) also distort the rib cage and make the diaphragm less efficient (Muller and Bryan, 1979).
Other factors also affect the infant’s work and efficiency of breathing. For instance, the full-term infant’s diaphragm is comprised of only 25% fatigue-resistant, slow-twitch, highly oxidative type I fibers, and the preterm infant has only 10% (the adult diaphragm has 55%). The lower percentage of type I fibers predisposes the diaphragm to fatigue (Keens et al., 1978). The intercostal muscles show a similar developmental pattern. Expression of various isoforms of the myosin heavy chains in muscle fibers of the diaphragm and the intercostal muscles of newborn and young infants may contribute to easier fatigability (Watchko and Sieck, 1993; Zhan et al., 1998). Less effective force-frequency, length-tension, and force-velocity relationships and a mismatch of energy supply and demand characterize diaphragmatic muscle and predisposes these newborns to fatigue, especially when there is widespread atelectasis (e.g., RDS) or poor chest wall compliance (e.g., anasarca) (Watchko et al., 1986; Watchko and Sieck, 1993). Methylxanthines improve neonatal diaphragmatic function (Maycock et al., 1992).
Healthy neonates breathe 30 to 60 times per minute to maintain normal oxygenation and to remove the increased amount of CO2 produced by an oxygen consumption of 6 to 10 cc/kg per minute. This respiratory rate also helps maintain the FRC by reducing the amount of time available for expiration and by trapping gas within the lung. At normal respiratory rates, the time constant of the lungs (compliance of lungs × airway resistance [C• × Raw]) is, on average, 0.25 seconds. A slow respiratory rate, apnea, or bradypnea can reduce the FRC, especially in preterm neonates (Fig. 17-3). A low level of PEEP (5 to 7 cm H2O) should always be added to maintain the FRC of infants and young children, especially when slow ventilatory rates are used.
Naturally occurring SAM or pulmonary surfactant is by far the most important mechanism for keeping alveoli of different sizes or diameters open. Initially, SAM appears in fetal lungs by 20 weeks’ gestation, even though no functional alveoli exist (Clements, 1961). At about 35 to 36 weeks’ gestation, the amount of SAM increases and is secreted onto the alveolar surface. The pulmonary surfactant monolayer creates a gas-liquid interface on the alveolar surface and drastically decreases surface tension during exhalation as the surface area decreases. During inspiration the monolayer is thinned, and the surface tension increases. SAM keeps the surface tension relatively constant regardless of the changes in the surface area and the radius of the alveoli. In neonates with RDS, there is loss of or inactivation of surfactant, and surface tension increases. The variability of surface tension normally occurring with changes in surface area is lost; alveoli develop higher pressures, collapse during exhalation, and remain collapsed (see Chapter 3, Respiratory Physiology in Infants and Children).
An additional mechanism that helps keep the alveoli open is the interdependence among alveoli, small airways, and lung parenchyma. Because the alveoli are attached to each other by connective tissues, the tendency of one alveolus to collapse during expiration pulls its neighbor open (Takishima and Mead, 1972). Millions of alveoli exerting this “pulling” effect help maintain FRC. Furthermore, blood-filled capillaries act as an exoskeleton for alveoli. If the pulmonary blood volume is inadequate, atelectasis develops. Increasing the blood volume increases FRC (Table 17-1) (Gregory, personal observation).
TABLE 17-1 Changes in FRC Associated with Blood Transfusion in Five Term Infants*
Functional Residual Capacity (FRC) mL/kg | Blood Transfused mL/kg |
15 ± 5 | 0 |
22 ± 4 | 5 |
27 ± 6 | 15 |
Gregory GA: Unpublished data.
Surface Active Material
The demonstration by Avery and Mead (1959) that surfactant deficiency is the primary cause of RDS eventually led Phibbs et al. (1991) and Fujiwara et al. (1990) to use commercially available surfactant prophylactically after birth or in the rescue mode after the symptoms of RDS were apparent. Intubated infants of fewer than 28 weeks’ gestation often receive 1 or 2 doses of surfactant 12 hours apart. During preoperative evaluation of a newborn with RDS, it should be determined when the last dose of SAM was given and if an additional dose is required before surgery because of progressive atelectasis or worsening blood gases and oxygen requirement.
Pulmonary gas exchange is only possible after a significant portion of the lung fluid present in utero is removed from the airways and alveoli. Increased pulmonary lymphatic flow and surface-active phospholipids and proteins facilitate this process (Humphreys et al., 1967; King, 1982). Surfactant reduces the surface tension of the gas-liquid interface of the alveoli, keeping the air spaces open at end expiration. This reduction of surface tension is critical for the maintenance of FRC and gas exchange.
Type I pneumocytes account for more than 90% of the alveolar surface cells; type II pneumocytes, the SAM-storing cells, populate most of the remaining surface. The total weight of SAM consists of about 10% protein, 90% lipids, and 0.1% carbohydrates. The lipids lower the surface tension, but the proteins allow absorption and dispersion of the lipids in the air-liquid interface. Proteins are also necessary for reuptake of SAM by type II cells. Congenital absence of these proteins leads to a chronic RDS-like state (Yurdakök, 2004). During inspiration SAM is spread over the alveolar surface. During expiration it is compacted to lower the alveolar surface tension. SAM also stabilizes small airways and is important for pulmonary defense mechanisms (Jarstarand, 1984).
Respiratory Distress Syndrome
Neonatal RDS occurs when the quantity of SAM is insufficient or when there is delayed synthesis or release of surfactant (e.g., in infants of diabetic mothers). Giving betamethasone to the fetus, by way of the mother, before 36 weeks’ gestation accelerates the production and release of SAM from the type II alveolar epithelial cells and reduces the incidence of RDS (Liggins and Howie, 1972). Typical clinical features of RDS include: early onset of tachypnea; intercostal, sternal, and suprasternal retractions; grunting respirations; oxygen desaturation; respiratory and metabolic acidosis; and death if not treated. A chest radiograph shows a diffuse reticulogranular pattern and air bronchograms (Fig. 17-4). Unless SAM is given, the natural course of RDS includes worsening symptoms for 2 to 3 days, resulting in either improvement or death. In some cases, respiratory function may deteriorate, rather than improve, because of a superinfection or complications of ventilatory support. RDS-associated death is often the result of severe lung injury induced by mechanical ventilation, sepsis, renal or hepatic failure, or CNS injury. This severe clinical pattern occurs less commonly when pulmonary surfactant is provided early. About 5% of all babies with RDS are born at term.
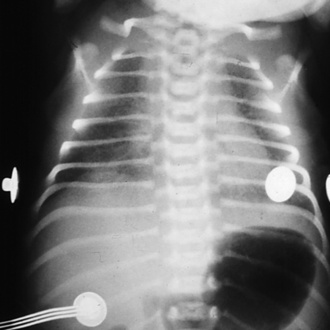
FIGURE 17-4 RDS. Note the ground-glass appearance and the presence of air bronchograms.
(From Brozanski BS, Bogen DL: Neonatology. In Zitelli BJ, Davis HW, editors: Atlas of pediatric physical diagnosis, ed 4, Philadelphia, 2002, Mosby.)
Before the development of commercially available surfactant, lung injury (e.g., pneumothorax, pulmonary interstitial emphysema, or BPD), blood loss from repeated blood sampling, and sepsis and its secondary effects (e.g., renal and hepatic dysfunction) were relatively common. Since 1990, exogenous SAM administration has significantly reduced the need for mechanical ventilation and oxygen therapy in preterm infants because of its effects on lung mechanics, including distensibility of the lung and end-expiratory volume stability (Morley et al., 2008). However, volutrauma (high tidal volume), and barotrauma (high airway pressure) persist in causing lung injury despite recent decreases in the incidence and severity of classic RDS and a reduction in the complications of pulmonary immaturity and supportive care. Despite all of these changes, the incidence of BPD has remained stable at about 20% (higher in ELBW infants) over the last 20 years (Bancalari and del Moral, 2001). But the BPD now seen in surfactant-treated lungs is different from classic BPD and is called new BPD. New BPD most commonly develops in ELBW infants, is characterized by a more uniform pattern of injury, and seems to represent an arrest of lung growth. Persistence of BPD, in spite of surfactant, suggests that other factors beside SAM deficiency contribute to BPD (Bancalari, 2001). Inflammatory and oxidative injury caused by infection, oxygen therapy, and “gentle” ventilation during periods of rapid lung growth and development, especially in the ELBW infants, seem to cause long-term pulmonary dysfunction (Kramer et al., 2009). The increased number of survivors has enlarged the number of patients (with and without BPD) requiring surgical treatment for coexisting long-term sequelae of prematurity.
Recent efforts to minimize lung injury caused by mechanical ventilation of premature infants have included introducing nasal CPAP (nCPAP) in the delivery room to prevent or treat RDS (Morley et al., 2008). This tactic avoids endotracheal intubation in patients and has markedly reduced the use of SAM. On the other hand, some infants with RDS (especially those weighing less than 1000 g) often fail a trial of nCPAP and require endotracheal intubation. They are usually treated with surfactant when their trachea are intubated.
Oxidative Injury in the Newborn
Although the role of oxygen in ROP is well established, oxygen toxicity of other organs, especially those of more mature newborns, is being increasingly recognized. Despite its widespread use for more than a century and the recognition that oxygen can be toxic for at least 50 years, the precise molecular mechanism of oxygen toxicity remains unknown (Tin, 2002). The severity of BPD has been correlated with oxygen exposure and with the oxygen saturation level used. In addition, greater oxidative injury to the heart, brain, and kidneys has been reported when neonatal resuscitation is accomplished with supplemental oxygen (Munkeby et al., 2004; Martín et al., 2007).
ROP is a multifactorial disease, but two main factors are associated with its development—prematurity and oxygen (see Chapter 27, Anesthesia for Ophthalmic Surgery). As stated, the neonate is exposed in utero to an oxygen tension of 20 to 30 mm Hg. Birth raises the Pao2 to 55 to 85 mm Hg that may represent hyperoxia, especially for preterm neonates. The retinal vessels grow radially outward from the center of the optic disc from about 16 to 40 weeks’ gestation. Neonates born before term have incompletely developed retinal vessels (Roth, 1977). Two phases of ROP have been described. The initial phase, which occurs between 30 and 32 weeks’ gestation, is associated with inhibition of growth and loss of retinal vessels. Growth of the retina under these circumstances leads to retinal hypoxia. The second phase of ROP is associated with effusive retinal neovascularization and occurs between 32 and 34 weeks’ gestation. New vessels form in the avascular regions and send up fibrous bands to the vitreous gel and lens. These strands are responsible for retinal detachment in some premature infants (Chen and Smith, 2007). Vascular endothelial cell growth factor (VEGF) is required for new vessel growth in utero and afterwards. Administering oxygen after birth decreases VEGF during the first phase of ROP, which decreases vessel growth. In the second phase of ROP, retinal hypoxia increases VEGF expression, which causes exuberant vessel overgrowth. Insulin-like growth factor-1 (IGF-1) is required for VEGF to have its maximal effect. During gestation, serum concentrations of IGF-1 increase, and the severity of ROP is directly correlated with the level of IGF-1. In utero, IGF-1 is supplied by both the placenta and the amniotic fluid. This is withdrawn at birth, decreasing the effectiveness of VEGF, and vessel growth decreases. Excessive amounts of oxygen turn off VEGF. Thus, both VEGF and IGF-1, under appropriate circumstances, inhibit vessel growth and cause vessel regression. Because of the severe retinal hypoxia in phase I of ROP, VEGF and IGF-1 concentrations increase. Retinal vessels’ growth causes the severe problems seen in the later stages of ROP. Avoidance of excessive oxygen concentrations in the operating room is therefore necessary (Betts et al., 1977).
Because there is evidence that supplemental oxygen causes a significant amount of injury, the use of supplemental oxygen should be limited to that required to maintain the oxygen saturation between 85% and 93%, both during resuscitation at birth and during the entire neonatal period (Rabi et al., 2007).
Preoperative Evaluation of the Pulmonary System
Physical Examination
Evaluating the chest movement and breathing pattern of patients from the foot of the bed often provides important information about ventilatory function. For example, do both sides of the chest move equally during inspiration? A difference in chest movement suggests a unilateral process, such as a pneumothorax, a pleural fluid collection, unilateral interstitial emphysema, or an endobroncheal intubation. Although auscultation of the chest may reveal decreased breath sounds on one side, it may not because breath sounds are readily transmitted from one side to the other in neonates. Consequently, the bilateral breath sounds of infants with a pneumothorax are often equal on each side. Percussing the chest or shining a high-intensity light through it may identify a pneumothorax when bilateral and equal breath sounds are present (Kuhns et al., 1975). Are intercostal, sternal, or suprasternal retractions present during spontaneous breathing? This is evidence of increased work of breathing and suggests collapse of lower airways (atelectasis), infiltration of air spaces (as with pneumonia, edema, meconium), pulmonary hypoplasia, or upper airway obstruction. Retractions are more prominent in preterm neonates because of the limited amount of fat and subcutaneous tissue surrounding their chests.
Does the nonintubated neonate grunt during expiration? Partial glottic closure during expiration (grunting) maintains a positive intrathoracic pressure, increases FRC, and improves oxygenation (Harrison et al., 1968). Grunting is common in patients who have reduced FRCs (as with HMD or pulmonary edema). Is expiration prolonged? Are the muscles of respiration actively used to expire? A prolonged expiratory phase of respiration suggests lower airway obstruction, for example, from secretions, masses (intrinsic or extrinsic to the airway), or bronchospasm. Additional details about treatment with bronchodilators (dose and frequency, response to various agents) and suctioning (frequency, quantity and quality of secretions, recent changes, results of cultures) are important for intraoperative planning for ventilatory care. An abnormal physical examination may suggest that further evaluation is required preoperatively (e.g., CT or magnetic resonance imaging [MRI] scan, fiber optic bronchoscopy, or pharmacologic intervention).
Stridor or a weak cry may be signs that the neonate has vocal cord injury (e.g., long-term intubation or congenital paralysis), subglottic stenosis, or subglottic granulomas (Hengerer et al., 1975). A smaller endotracheal tube may be required to prevent further laryngeal or tracheal injury, if tracheal intubation is planned in such patients. However, small endotracheal tubes are more easily occluded with secretions, and suctioning may be more difficult, especially during surgery. In addition, weaning patients from mechanical ventilation postoperatively may be difficult or impossible if the endotracheal tube is small for the infant’s age. Mask or laryngeal mask airways (LMAs) or other airway devices may make the need for tracheal intubation unnecessary; however, LMAs increase dead space by adding large volumes above the glottis (Table 17-2). Most cases of neonatal stridor require the opinion of a pediatric pulmonologist, surgeon, or otolaryngologist to define the safest and most efficient means of providing adequate evaluation and treatment of the stridor (e.g., bronchoscopy, CT scan or MRI, or surgery). Clearly, planning for these evaluations must consider how to reduce the risk-to-benefit ratio of each diagnostic or therapeutic examination, especially if deep sedation or general anesthesia is required. The need for and timing of tracheostomy should be considered early and discussed with the parents before surgery, especially when general anesthesia is required.
Blood Gas Analysis
Analysis of pHa, Paco2, and base deficit provides valuable information about the neonate’s pulmonary function. Hypercarbia is the usual, normal physiologic compensation for metabolic alkalosis (e.g., from chronic furosemide administration). The level of Paco2 alone does not tell whether the child has respiratory compensation for a metabolic acidosis or a metabolic compensation for respiratory acidosis. Acute hypercarbia produces acidosis, and the pHa is appropriate for the level of Paco2 (assuming there is no coexisting metabolic acidosis). Of course, mixed metabolic and respiratory abnormalities further complicate interpretation of the relationship between pH and Paco2. If the Paco2 is chronically elevated and the pHa is relatively normal, acutely reducing the Paco2 to normal will precipitate an acute respiratory alkalosis, which may significantly reduce cerebral blood flow. Every 1 mm Hg change in Paco2 between 20 and 80 mm Hg Paco2 causes a 2% change in cerebral blood flow. Thus, if the Paco2 is 60 mm Hg, and is acutely reduced to 40 mm Hg, cerebral blood flow will decrease by as much as 40%. If this occurs in conjunction with hypotension, cerebral perfusion may well be inadequate. Hypocarbia and severe alkalosis should be avoided when possible, because it can result in poor neurologic outcomes (Curry et al., 2008).
Mechanical Ventilation
Infants who require mechanical ventilation before surgery often require increased support of ventilation during and after surgery, and patients who are spontaneously breathing and treated with nasal CPAP before surgery, usually require tracheal intubation and mechanical ventilation for variable periods after surgery. The lungs of neonates, especially premature neonates, are sensitive to oxidative damage, volutrauma (high volume trauma) as a result of overdistention of the lungs), and shear stress injury (low volume trauma) caused by repetitive opening and collapse of alveoli and terminal airways). In particular, lungs are easily overdistended during hand ventilation. Interestingly, neonates treated with CPAP alone have less evidence of pulmonary inflammation and CLD (Jobe et al., 2002). Small tidal volumes, rapid respiratory rates, and permissive hypercapnea seem to reduce the amount and severity of neonatal lung injury, suggesting that intraoperative ventilation strategies should use the lowest effective tidal volumes (inspiratory and end-expiratory pressures) and inspired oxygen concentrations possible (Thomas and Speer, 2008). The Sao2 should be maintained between 85% and 93% in preterm neonates, which may require the use of room air. Mild hypercapnea (Paco2 of 45 to 55 mm Hg) is acceptable if there is no evidence of increased intracranial pressure, pulmonary hypertension, or severe metabolic acidosis. Aggressive hyperventilation should be avoided, and no attempt should be made to reduce the Paco2 to 40 mm Hg or less without a very good clinical reason for doing so. Although knowledge of the ventilator settings used in the NICU is important for setting the initial respiratory rate and airway pressures in the operating room, the less sophisticated ventilators used during surgery are rarely as effective as the NICU’s ventilators. Furthermore, the impact of positioning for surgery (e.g., lateral or prone), the procedure itself, retractors, surgeon’s hands, fluid administered, anesthesia, and other factors all affect the effectiveness of mechanical ventilation in the operating room.
The Cardiovascular System
Transitional Circulation
The fetal circulation is characterized by increased PVR, decreased pulmonary blood flow, decreased systemic vascular resistance, and right-to-left blood flow through a PDA and foramen ovale (Rudolph, 2007). At birth, the onset of ventilation and the elimination of the placental circulation have dramatic effects on systemic and PVRs (see Chapters 4, Cardiovascular Physiology, and 21, Congenital Cardiac Anesthesia: Non-Bypass Procedures). Pulmonary vascular resistance (PVR) decreases and pulmonary blood flow increases. Simultaneously, systemic vascular resistance increases, and the left atrial pressure rises. This functionally closes the foramen ovale and stops right-to-left shunting at this level. However, bidirectional shunting through the ductus arteriosus often continues for the first 24 hours of extrauterine life in healthy infants. If the ductus arteriosus fails to close, blood is shunted left-to-right into the pulmonary circulation as the PVR declines after birth. Anatomic closure of the ductus arteriosus occurs after several days, and shunting of blood through this structure ceases. The neonate now has the circulation of an adult.
If arterial hypoxemia or acidosis occurs during the first few days of life, the neonate’s circulation may revert to the fetal pattern (i.e., pulmonary arterial vasoconstriction, pulmonary hypertension, and reduced pulmonary blood flow). The resultant increase in right atrial pressure reestablishes right-to-left shunting of deoxygenated blood through the foramen ovale and ductus arteriosus, producing arterial hypoxemia (Rudolph and Yuan, 1966). This return to the fetal circulatory pattern, called PFC or persistent pulmonary hypertension of the newborn (PPHN), further exacerbates the hypoxemia and acidosis. Although numerous treatments for PPHN (e.g., hyperventilation or vasoactive agents) have been used, a selective and highly effective vasodilator of the pulmonary circulation has not been identified. PPHN may be an isolated phenomenon, or it may be associated with a variety of clinical conditions, including meconium aspiration, sepsis, polycythemia, diaphragmatic hernia, hypoxemia, acidosis, and severe hypotension. An echocardiogram should be performed to exclude structural cyanotic heart disease (Peckham and Fox, 1978; Murphy et al., 1981). The primary treatment of PPHN is aggressive hemodynamic support, including maintaining intravascular volume, initiating inotropic support (especially if PPHN is associated with sepsis or asphyxia), correcting acidosis and electrolyte disturbances, and administering antibiotics when appropriate. Ventilatory strategies have moved away from hyperventilation (pH greater than 7.5) and hyperoxia to more gentle approaches, including maintenance of normal oxygenation, Paco2, and pH with either conventional or high-frequency mechanical ventilators. Vasodilatory agents such as NO are often used, but the protocols vary from institution to institution. NO has significantly reduced the need for extracorporeal membrane oxygenation to treat PPHN (Field et al., 2007).
Nitric Oxide
Control of the pulmonary circulation, especially during the transition from fetal to postnatal life, is complex and depends on the interaction of a variety of mediators and factors, receptors, and neurologic, endocrine, and vascular control mechanisms, both in endothelial and smooth muscle cells (Kinsella and Abman, 1995). For example, increased oxygen tension and expansion of the lungs with the onset of breathing decreases PVR directly. The rise in blood flow imparts shear stress to the vessels, which distends them, flattening the endothelium and the smooth muscle cells, and promotes the release of various mediators (Haworth, 2006). The balance between the effects of vasodilators (e.g., NO and prostacyclin) vs. vascoconstrictors (e.g., endothelin-1 [ET-1], leukotrienes) significantly affects pulmonary blood flow. As noted by Haworth (2006), “The pioneering studies of Dr. Dawes and his colleagues in Oxford in the 1960s on fetal sheep showed that mechanical ventilation reduced PVR and that the response was enhanced when the inspired gas was enriched with oxygen” (Dawes, 1968). Fifty years later, no single factor has yet been identified as being primarily responsible for the initiation of pulmonary vasodilation at birth. Nor do we know whether the endothelial cell or the smooth muscle cell is the prime target (Haworth, 2006),
NO is produced by the pulmonary vascular endothelial cells and is important for pulmonary vasodilation. NO is used to treat PPHN and, in a less well-defined role, to stimulate angiogenesis and promote lung growth, primarily in the ELBW infant. Inhaled NO has a rapid onset of action, is potent, and is a selective pulmonary vasodilator. Unfortunately, up to 40% of infants with PPHN do not respond to NO, and with the use of this drug there has been no positive effect on the incidence of BPD or neurodevelopmental deficits, mortality, or long-term outcome (Steinhorn, 2008).
Regulation of production and metabolism of NO occurs at multiple levels. First, NO production is subject to endothelial nitric-oxide synthetase activity (eNOS; lung eNOS is type III). eNOS has both reductase and oxygenase domains. When its substrate L-arginine is available, oxidation of NADPH and NO synthetase produce NO. On the other hand, when the concentration of substrates or cofactors (e.g., heat shock protein 90) is reduced, NOS is uncoupled and, instead of NO, reactive oxygen species such as peroxynitrate are produced. In fact, oxidative stress may contribute to the development of PPHN (Brennan et al., 2003). The balance between the vasodilating effects of oxygen and the injury caused by oxidative stress is a delicate one, and the injury may occur quickly. For example, ventilating the lungs of lambs with an Fio2 of 1.0 for only 30 minutes blunted the subsequent response to NO (Lakshminrusimha et al., 2007). During high-dose NO administration, the deleterious effects of generating oxygen radicals must be balanced against its vasodilatory benefits.
Second, the activity of soluble guanylate cyclase and cyclic guanosine monophosphate (cGMP)-specific phosphodiesterase (PDE5) on smooth muscle cells contributes to NO’s effectiveness in stimulating cGMP production. PDE5 hydrolyzes cGMP, which controls the duration and magnitude of cGMP’s effect. Agents that inhibit PDE5, such as sildenafil, promote pulmonary vasodilation and have been used extensively to treat pulmonary hypertension in adults. There is less experience using these drugs in newborns with PPHN (Baquero et al., 2006; Martell et al., 2007). Development of a parenteral formula of these drugs may improve delivery of PDE5 antagonists for the treatment of PPHN (Mukherjee et al., 2009). Sildenafil has effectively diminished rebound pulmonary hypertension during weaning from NO (Atz and Wessel, 1999). Of note, both NO and sildenafil have been reported to stimulate lung growth. Ongoing trials of NO to minimize CLD may eventually define the role of this agent in the ELBW infant (Steinhorn and Porta, 2007). No agents are available that directly activate guanylate cyclase in patients.
The cyclic adenosine monophospate (cAMP) pathway is another mechanism by which pulmonary vasodilation can be induced. Prostacylin (PGI2) stimulates adenylase cyclase and increases cAMP. However, intravenous infusion of this drug causes systemic hypotension, which often prevents the desired therapeutic effect from being achieved. Inhaled PGI2 is a selective pulmonary vasodilator, but its efficacy in PPHN remains to be firmly established. Milrinone, on the other hand, inhibits cAMP metabolism by interfering with PDE3 and has been used clinically as adjunct therapy to NO (McNamara et al., 2006).
Antagonizing vasoconstriction is another strategy for treating PPHN. ET-1, a potent vasoconstrictor produced by the endothelium, and NO interact in a feedback loop. For example, NO decreases ET-1 production and ET-1 increases superoxide release, which impairs NO-induced vasodilation (Abman, 2007). In addition to directly antagonizing ET-1, other strategies to interrupt vasoconstriction offer promise. For example, a GTPase, RhoA, and its protein ρ-kinase are important for regulation of vascular tone, especially for maintaining the high PVR present in utero. Thus, inhibitors of both ET-1 and ρ-kinase may be useful for the treatment of PPHN. Finally, dysfunction of the signaling pathways of VEGF may be important in the pathogenesis of PPHN. For example, VEGF releases NO, and inhibition of VEGF receptors down-regulates eNOS. Reduced levels of VEGF have been reported in infants with PPHN (Lassus et al., 2001).
Myocardial Ultrastructure
The fetal and adult myocardia contract and relax using the same basic processes—increases in the cytosolic calcium concentration to cause force generation and decreases to cause relaxation (Crick et al., 1998). At all stages of maturation, the ventricle must develop force against a varying resistance or load (contraction and ejection) and then relax (filling). Membranes present in the adult myocardium that control both calcium flux and the contractile system are also present in the fetal heart; however, there are qualitative and quantitative age-related differences in these membranes. Progression toward adult myocardial function involves developmental changes in these structures associated with the mechanics of force generation (e.g., the sarcomere and myofibril), those involved with controlling calcium flux (e.g., the sarcoplasmic reticulum, receptors, channels, exchangers, transporters, and pumps), those related to myocardial compliance (e.g., extracellular matrix and cytoskeleton), and sympathetic innervation. In part, age-related differences in the response to calcium and various pharmacologic agents are the result of the developmental state of myocardial anatomy and function.
Force Generation
Sarcomere
The immature myocardium develops less force against a load than that of the adult (Anderson et al., 1984). For example, the premature and neonatal hearts are unable to maintain output against an arterial pressure that would be considered low in the adult. In part, this reflects a smaller myocardial mass and a thinner left-ventricular wall. In addition, the velocity and quantity of sarcomere shortening is less in isolated immature myocytes than it is in adult myocytes (Nassar et al., 1987).
Both the number and size of myocytes increase before and after birth. The shape of the myocyte changes from a smooth, spherical structure to a more irregular, rectangular one. With this change in shape, the surface area-to-volume ratio decreases. This process occurs more rapidly in the left ventricle than in the right. The neonatal period is characterized by significant development of the left ventricle and a shift from right-ventricular to left-ventricular predominance. There also is a marked increase in left-ventricular work that is the result of a higher stroke volume, systolic arterial pressure, and wall tension. Right-ventricular systolic pressure and work decrease. Relative to body weight, left-ventricular mass increases and right-ventricular mass decreases. The increase in the number (cell division) and size (hypertrophy) of the myocytes is more pronounced in the left ventricle than in the right. By the second month of life, increased cell size, rather than increased cell number, becomes the predominant developmental phenomenon. This has major implications for patients with CHD, especially those with lesions that obstruct outflow from either ventricle (Rudolph, 2000). Control of postnatal development is not completely understood, but α-agonist stimulation, cortisol, thyroid hormone, and a variety of growth factors seem to be important (Brown et al., 1985; Rudolph, 2000).
Myofibrils
The number of myofibrils per unit of intracellular volume increases, and their arrangement becomes more highly organized during myocyte maturation after birth. In the fetus, the myofibrils appear somewhat chaotic. In the adult they are in long, parallel rows that alternate with rows of mitochondria (and sarcoplasmic reticulum). In the immature myocardium, myofibrils are arranged in thin layers, and groups of nuclei and mitochondria reside in the center of the cell. In part, this difference in myofibril arrangement may be the anatomic basis for the differences in control of the intracellular calcium concentration and in sensitivity to calcium channel blockers in the neonate as compared with the adult (see Sarcoplasmic Reticulum, p. 526). That is, transsarcolemmal movement of calcium from the outside to the inside of the cell causes contraction of the immature myocardium, whereas intracellular release of calcium is responsible for contraction of the adult myocardium. The irregular arrangement of mitochondria in the immature myocardium is likely to prevent the organized contractile activity seen in the adult heart, where the mitochondria alternate regularly within sarcomeres.
Calcium Flux
Sarcolemma
Sarcoplasmic reticulum
Caffeine, which releases calcium from the sarcoplasmic reticulum, has little effect on neonatal myocardial contractility. This suggests that extracellular, rather than intracellular, calcium controls contractility in the neonatal myocardium, probably through calcium entry via L-type channels of the sarcolemma. Also, extracellular calcium appears to more effectively activate myocardial contraction of the immature myocardium than of the adult myocardium. Compared with the adult heart, immature hearts are more sensitive to calcium-channel antagonists (Boucek et al., 1984). Furthermore, the newborn heart requires higher concentrations of extracellular calcium to achieve maximal contractility (Jarmakani et al., 1982). With maturation, the amount of sarcoplasmic reticulum and the number of specialized connections of sarcoplasmic reticulum with other membranes increases, as does the efficiency of pumping calcium. Finally, there is an increase in Ca+-ATPase activity, which has been linked to improved sarcoplasmic-reticulum function with maturation (Mahony and Jones, 1986; Mahony, 1988). Age-related increases in the amount and organization of the sarcoplasmic reticulum improve systolic and diastolic myocardial function with age (see Cardiovascular Function in the Newborn, p. 527).
Contractile proteins
Myocardial Compliance
Cytoskeleton
Sympathetic Innervation
The sympathetic nervous system modulates a wide range of critical events in the developing myocardium, including cell growth and differentiation and distribution of and sensitivity to calcium. With maturation of adrenergic innervation, the quantity of neurotransmitters and the number of receptors increase. At birth, adaptation to postnatal hemodynamics is mostly mediated by the α-adrenergic system. That is, an early high level of α-adrenoreceptors may be critical for left-ventricular growth in the early postnatal period. In fact, α-1-blockade interrupts the increase in protein synthesis in cultured myocytes exposed to α-stimulation (Robinson, 1996). For example, the increase in adrenergic fibers innervating the myocardium modulates widespread development and expression of the various contractile systems (e.g., expression of the contractile proteins, efficiency of the calcium channel, and expression of myosin ATPase isoforms). Finally, the activity of adenyl cyclase, an important enzyme involved in intracellular β-stimulation, increases in concert with the increase in catecholamine levels. The interaction of adrenergic innervation, catecholamine concentrations, and functional changes in multiple proteins that regulate responses to various agonists is complex and difficult to accurately extrapolate to specific clinical scenarios.
Cardiovascular Function in the Newborn
In spite of the intense age-related changes in myocardial physiology and function, the qualitative characteristics of the fetal and neonatal heart are similar to those of the adult in that blood pressure is directly proportional to CO and systemic vascular resistance (Table 17-3). The primary determinants of CO are heart rate and stroke volume. Preload, afterload, and myocardial contractility interact to determine ventricular performance. At all times, oxygen delivery and oxygen demands are interdependent; oxygen delivery (CO) is regulated by oxygen consumption (metabolism).
TABLE 17-3 Comparison of Neonatal and Adult Myocardial Functions
Neonate | Adult | |
Cardiac output | Rate dependent | Stroke volume and rate |
Contractility | Reduced | Normal response |
Starling response | Limited | Normal response |
Catecholamine | Reduced | Normal response |
The high collagen content and high ratio of Type I collagen to Type III collagen may be responsible for the relative noncompliance of the neonatal heart and its limited capacity to respond to volume loading (Klopfenstein and Rudolph,1978). This nondistensible heart has limited capacity to increase stroke volume and augment CO when faced with an increased preload. Thus, the Frank-Starling response appears to have a limited role, and heart rate is the primary means of increasing CO in the newborn. Over the first months of life, myocardial contractility gradually increases, which allows CO to be maintained over a wider range of preloads and afterloads. Similarly, the increase in contractile proteins and the shift in the expression of various isoforms; the development of sarcoplasmic reticulum and T tubules, adrenergic innervation, calcium recruitment and transport; and the overall response of the myocardium to stress or increased demands all contribute to complex changes in force development.
The newborn’s CO per unit weight is the highest of any age group (approximately 200 mL/kg per minute). Teitel (1985) hypothesized that the greater increase in myocardial performance with adrenergic stimulation in older lambs is to the result of a “lesser resting β-adrenergic tone” in the older animals. That is, the baseline state of the newborn myocardium is at higher level of “β-adrenergic tone,” and the high resting CO of the newborn limits its ability to further increase CO in response to increased demand or to adapt to wide variations in preload or afterload. Because the newborn’s normal heart rate is high, increasing the heart rate has limited effect on CO, but decreasing the heart rate dramatically decreases output.
Volume loading of the immature ventricle increases CO, but the effect is less than that that seen at older ages (Klopfenstein and Rudolph, 1978). Similarly, the tolerance of the immature myocardium to increases in afterload is less than it is at older ages. Thus, the impaired ventricular function of the fetus (i.e., preterm baby) and newborn are the result of fewer myofibrils; decreased sympathetic innervation; decreased β-adrenoreceptor concentration; immaturity of the structure and function of the sarcoplasmic reticulum; maturation-specific mechanisms for calcium uptake, release, and storage; and a specific spectra of expression of various isoforms of contractile and noncontractile proteins, channels, exchangers, and enzymes.
Cardiovascular Evaluation
Preoperative evaluation of the newborn should focus on understanding “normal,” because infants of the same gestational age can vary significantly in their baseline heart rates and blood pressures (Fig. 17-5). Acid-base status (electrolytes, pH, Paco2), urine output, and rates of fluid administration must be interpreted in the context of “normal” blood pressure and heart rate and the interventions required to maintain normalcy. Trends in these data over the hour, 6 hours, and 12 to 48 hours prior to examination help clarify the infant’s cardiac function. It is necessary to understand whether there were responses to fluid boluses and inotropic intervention, and if so, what those responses were with respect to maintenance of cardiovascular stability. This critical insight is important when developing a rational anesthetic plan. Finally, evaluation of the current level of sedation and the response to various agents also helps determine which drugs (e.g., morphine, fentanyl) and the amounts of each drug that are required to achieve the desired effect. This knowledge will guide the anesthesiologist in determining which drugs may be most effective during surgery.
The Central Nervous System
During the third trimester, cortical gray and white matter volumes increase fourfold to fivefold. Growth and differentiation of dendrites and axons, proliferation of glia, synaptogenesis, and myelination characterize this dramatic process. The proliferation in the cerebellum is even more rapid than the cerebral cortex and is characterized by intense migration of various populations of cells (Allin et al., 2001; Limperopoulos et al., 2005b; Limperopoulos and du Plessis, 2006).
In parallel with the intense growth of the parenchyma, the vascular network of the late-gestation brain also develops rapidly. The long and short penetrators lengthen and arborize, leading to a decrease in end- and border-zone blood flow. Despite this rapid growth, cerebral blood flow in late fetal life (8 to 15 mL/g per minute) is remarkably low compared with that of the adult. Blood flow to the white matter is even lower, approximately 25% of the flow to the cerebral cortex (Khwaja and Volpe, 2008). The presence of a pressure-passive cerebral blood flow may further predispose the newborn, especially the premature newborn, to ischemic injury (Greisen, 2005).
In addition to the overall patterns of intense brain growth in late gestation, the development of specific cellular elements defines the unique susceptibility of the preterm brain to hypoxic and ischemic insults. Microglia are macrophages that are prominent in the normal human brain during development and are concentrated in the cortical white matter. This population of cells peaks during the third trimester, the period of highest vulnerability to the white matter injury that is common in the premature infant. Although microglia have a critical function in normal development, activation of these cells precipitates release of cytokine and glutamate, producing reactive oxygen and nitrogen species. Preoligodendrocytes (precursors to myelinating oligodendrocytes) are especially susceptible to injury from oxidative and excitotoxic stress. Neuropathologic studies have documented that an intense inflammatory response in the white matter accompanies periventricular leukomalacia (PVL), and specifically, microgliosis characterizes diffuse, noncystic PVL. Diffuse white matter injury on MRI correlates with neurodevelopmental delay in expremature infants (Woodward et al., 2006; Glass et al., 2008).
Another unique population of cells that is prominent during development is the so-called “subplate neuron” group. The subplate is three times thicker than the cortical plate between 22 and 36 weeks’ gestation, but it is absent at term (McQuillen and Ferriero, 2005). These neurons express neurotransmitters and growth factors that seem to be critical in orchestrating the migration of cells from the germinal neuroepithelium to distant sites (e.g., the corpus callosum or thalamus). This layer receives synapses and organizes connections with distant structures, produces axons, and serves as a waiting zone for cells and axons as their final ultimate location in the cortex is defined (Leviton and Gressens, 2007). Injury to the subplate may disrupt the critical interaction and feedback between the cortex and thalamus, leading to long-term functional consequences. The connectivity among various distant structures during a time of critical brain development has been postulated to correlate with deficits identified in the expremature infant in the specific areas of behavior, cognition, and other complicated higher cortical functions (e.g., executive function) (Limperopoulos et al., 2005b; Boardman et al., 2006; Kapellou et al., 2006; Counsell et al., 2007).
Derangements of early brain development correlate with abnormalities in the two major structures of embryogenesis: the neural tube (future brain and spinal cord) and the prosencephalon (future forebrain) (Volpe, 2001). Primary development of the neural tube (3 to 4 weeks’ gestation) and prosencephalon (2 to 3 months’ gestation) are complete early in gestation. The term primary neural tube includes embryogenesis of both the brain and the spinal cord. The prosencephalon develops shortly after closure of the anterior neural tube and is related to both the telencephalon (future cerebral hemispheres) and the diencephalon (future thalamus and hypothalamus). Neural tube anomalies, such as anencephaly, craniorachischisis totalis, myeloschisis, and encephalocele, associated with these early events are dramatic and often fatal. Similarly, severe prosencephalic anomalies (holoprosencephalies) are often fatal, especially when they are associated with chromosomal abnormalities (e.g., trisomy 13-15 or trisomy/ring/deletion 18).
A less severe form of abnormal neural tube closure includes the lesions of spina bifida (incidence of 3 to 7 per 10,000 births). These neural tube anomalies are clinically important because affected infants often survive and have lifelong problems. The four primary types of spina bifida are categorized according to the severity of the defect. In the first type, spina bifida occulta, the divided vertebral arch, spinal cord, and meninges are covered with skin. Hair often protrudes from the skin overlying the defect, forming a sacral dimple. The presence of either hair or a dimple in this area should make anesthesiologists cautious of performing a caudal block without further information. In the second type, spina bifida cystica, neural tissue and its coverings protrude through the incompletely formed vertebral arch as a cystlike structure. In the third type, meningocele, the neural tube lies in its normal position but the meninges protrude through the defect; skin usually covers this lesion. In the fourth type, meningomyelocele, both the spinal cord and the meninges protrude, often without skin. These lesions can occur at any level of the spine. Hydrocephalus is a common accompaniment of myelomeningocele—60% in occipital, cervical, thoracic, or sacral lesions and about 90% in thoracolumbar, lumbar, and lumbosacral lesions (Lorber, 1961). In most infants, hydrocephalus is not evident before the meningomyelocele is closed, because cerebrospinal fluid leaks through the open lesion and decompresses the ventricles.
An Arnold-Chiari malformation is an abnormality of the hindbrain that occurs in most patients with meningomyelocele (Fig. 17-6). The medulla oblongata is flattened and elongated and, along with the fourth ventricle, protrudes into the spinal canal through the foramen magnum. The downward position of the medulla also elongates the lower pons and upper medulla and may compress brainstem nuclei and cranial nerves. If the cerebellar tonsils are displaced through the foramen magnum, aqueductal stenosis and hydrocephalus can occur. Whether it becomes necessary to decompress the posterior fossa depends on the severity of the anatomic abnormalities and the symptoms. For example, anomalies that are severe enough to cause apnea, vocal cord paralysis, or central and obstructive ventilatory disturbances require early correction (Kirk et al., 1999). Up to 20% of infants with myelomeningocele have sleep-disordered breathing, and some of these patients require a tracheostomy (Kirk et al., 2000). The anesthesiologist should be aware that a wide range of abnormalities associated with myelomeningocele exists beyond infancy, because this group of patients commonly requires ventriculoperitoneal shunt revision or urologic or orthopedic procedures.
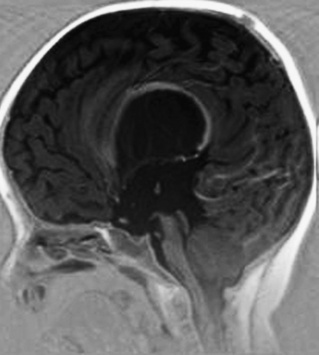
FIGURE 17-6 This MRI of Chiari’s malformation shows the brain stem being pushed down through the foramen magnum.
(Courtesy Jim Barkovich, MD, University of California, San Francisco.)
Other disorders, such as agenesis of the corpus callosum and septum pellucidum, are less commonly fatal, but they are often associated with abnormal neuronal migration and significant clinical abnormalities. Agenesis of the corpus callosum is usually associated with a syndrome (e.g., Aicardi’s or Andermann’s syndrome) or a chromosomal abnormality (e.g., 8, 11, 13, 15, or 18). As many as 80% of patients without a corpus callosum have other brain anomalies, as well as non-CNS malformations (Parrish et al., 1979; Jeret et al., 1987). Partial agenesis of the brain probably occurs later in development and is associated with clinical syndromes that have migrational and structural disorders. Agenesis of the septum pellucidum is never an isolated lesion. It is often occurs in conjunction with optic nerve hypoplasia (septooptic dysplasia).
Once the structure of the neural tube and the prosencephelon are established (2 to 3 months of gestation), further development of the CNS is by proliferation and migration. Neurons proliferate from ventricular and subventricular regions at every level of the developing nervous system. From the second to the fourth month of gestation, some glia are formed, but most proliferating cells are neurons. From the fifth month of gestation into adulthood, glial multiplication is the primary process. Movement of cells from the ventricle to subventricular sites is important for our understanding of the pathophysiology of the preterm infant brain. For instance, the limited glial migration that occurs during early gestation is important for normal migration of other cells. Arterial and venous vessels develop at this same time. These developmental processes affect the prognosis of preterm infants, especially those born before 30 weeks’ gestation (see Chapter 22, Anesthesia for Neurosurgery). The two diagnoses most commonly associated with CNS injury in preterm infants are periventricular hemorrhagic infarct (PVHI) and PVL.
Intraventricular/Periventricular Hemorrhage-Germinal Matrix/Intraventricular Hemorrhage
The pathophysiology of intraventricular hemorrhage (IVH) is related to the structure of the immature brain (Fig. 17-7) (Volpe, 2001). Proliferating ventricular and subventricular areas of the developing nervous system are very cellular and vascularized; neuroblasts (cerebral precursors for both gray and white matter) and glioblasts originate from these sites between 10 and 20 weeks’ gestation. This region of the developing brain is gelatinous in midgestation, but the gelatinous nature of the area gradually decreases and is barely present in full-term infants. The dense, well-developed vascular network present in midgestation (which arises from the middle and anterior cerebral and anterior choroidal arteries) drains into a venous system that receives blood from the entire brain and terminates near the head of the caudate nucleus, where the veins join the Galen vein. The primary bleeding site in the premature brain is where veins and capillaries join, rather than where capillaries join to arteries or arterioles. Between 25 and 32 weeks’ gestation, the germinal matrix is most prominent at the head of the caudate nucleus, and this is the usual site of germinal matrix hemorrhage.
IVH has been divided into categories that correlate with the severity and extent of the initial injury and with the clinical outcome (Papile et al., 1978). Grade 1 is a subependymal hemorrhage with minimal or no IVH. Grade II is an IVH without distention of the ventricles. Grade III is IVH with enlargement of the ventricles by intraventricular blood. Grade IV, the worst category of all, includes IVH, ventricular dilation, and extension of the bleeding into the parenchyma of the brain. Volpe (2001) revised the grading as follows:
Echo density of the periventricular parenchyma falls into a separate classification.
The incidence and severity of IVH vary, but in general, they increase with decreasing gestational age and have an overall incidence of 7% to 23%. The incidence of grades III and IV IVH (10% to 12%) in VLBW infants has not changed during the last 10 to 15 years (Faranoff et al., 2003). In a group of ELBW infants, grade I IVH was present in 13%, grade II in 7%, grade III in 7%, and grade IV in 6% of neonates. The 13% incidence of grades III and IV IVH is similar to that reported by Fanaroff (Adams-Chapman et al., 2008). Mortality is high in infants with grade IV bleeds (at approximately 50%), but it is not increased in those with grades I and II bleeds (Vohr et al., 2000). While grades I and II IVH are not associated with severe neurologic sequelae, about 35% of survivors of grade III and 90% of grade IV IVH have poor neurodevelopmental outcomes (Whitelaw, 2001). Furthermore, posthemorrhagic hydrocephalus that is severe enough to require a ventriculoperitoneal shunt has the highest incidence of severe neurocognitive impairment in early childhood (78% in the group with grade III lesions and 92% in those with a grade IV IVH) (Adams-Chapman et al., 2008). Even though there is less significant neurodevelopmental delay in near-term infants with uncomplicated grade I or II IVH, they are reported to have lower developmental functioning, and their grey matter volumes are 16% smaller by MRI than predicted (Vasileiadias et al., 2004; Patra et al., 2006),
Periventricular Leukomalacia
This bilaterally symmetric, nonhemorrhagic lesion is the result of white matter necrosis that occurs dorsally and laterally to the external angles of the lateral ventricles (Fig. 17-8). In the past, this lesion was cystic and easily visualized on ultrasound. More recently, the most common form of PVL seen in ELBW infants on MRI consists of diffuse cerebral white matter injury without cyst formation. PVL is sometimes inappropriately thought to be a consequence of IVH, because both are commonly present on the same scan. However, PVL is a postischemic lesion, not a venous infarction (Takashima et al., 1986). While focal and tissue necrosis may cause cavitation and cyst formation, eventually the loss of oligodendrocytes and abnormal myelinization reduce the white-matter volume, causing ventriculomegaly. A consistent clinical correlate of PVL is spastic diplegia. More recently, a variety of neurodevelopmental and cognitive deficits have been associated with noncystic PVL in preterm infants.
Autoregulation of Cerebral Blood Flow
Critically ill newborns often require mechanical ventilation, airway suctioning, and intravenous fluid boluses, all of which can affect blood pressure, CO, heart rate, and cerebral blood flow. Hypoglycemia, hypercarbia, hypocarbia, hypoxia, hypo- or hypernatremia, and hypocalcemia can also dramatically affect the neonate’s hemodynamic status. Fluctuations in blood pressure and CO often affect the newborn’s CNS, since cerebral blood flow may change as a result of absent or attenuated cerebrovascular autoregulation. Cerebral blood flow autoregulation is present in both preterm and full-term newborns, but it is tenuous (Fig. 17-9). Also, the range of pressures over which blood flow is regulated is narrower for preterm infants. Cerebrovascular autoregulation is easily disrupted in neonates, and the normal blood pressure of preterm infants is close to the lower autoregulatory limit (Lou et al., 1979; Versmold et al., 1981; Tweed et al., 1983;Lou 1998). With decreasing gestational age, the lower limit approaches normal blood pressure. Similarly, the upper range of autoregulation may be approached during later gestational development (Papile et al., 1985). Furthermore, the normal range of autoregulation can be disturbed or disrupted by hypoxia, acidosis, seizures, and by the low diastolic blood pressures of patients with a PDA (Lou et al., 1979; Tweed et al., 1983; Lou, 1998). Rapid increases in arterial blood pressure can rupture the fragile vessels of the immature brain, while hypotension and low perfusion pressures may cause ischemia.
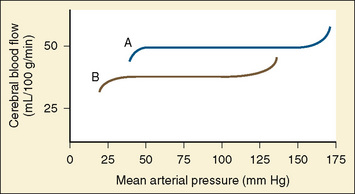
FIGURE 17-9 Autoregulation of cerebral circulation in neonates (curve B) and adults (curve A).
(Redrawn from Harris MM: Pediatric neuroanesthesia. In Berry FA, editor: Anesthetic management of difficult and routine pediatric patients, ed 2, New York, 1990, Churchill Livingstone. Data from Hernandez MJ, Brennan RW, Vannucci RC, Bowman GS: Cerebral blood flow and oxygen consumption in the newborn dog, Am J Physiol 234:R209, 1878).
Hypoxic Ischemic Injury
If the amount of cerebral blood-flow is insufficient for cerebral oxidative metabolic needs, severe, long-term neurologic injury may occur. Asphyxia has three biochemical features: hypoxemia, hypercapnia, and metabolic acidosis. Some authors have suggested that the normal events occurring during labor and delivery, including uterine contractions, cause a degree of asphyxia in all fetuses. Although severe asphyxia can cause hypoxic-ischemic encephalopathy (HIE) and injure other organs, HIE often develops without injury to other organs. HIE is defined as abnormal levels of consciousness, the presence of seizures, and a history of severe asphyxia (e.g., umbilical cord pH < 7.0, Apgar score of 0 to 3 for longer than 5 minutes, neurologic dysfunction) (Leuthner and Das, 2004). Neurologic abnormalities caused by infections or metabolic causes should not be labeled HIE. HIE occurs in approximately 4 to 5 per 1000 live births. One study demonstrated a decrease in HIE between 1990 to 2000 (Wu et al., 2004a).
Unfortunately, no specific clinical or biochemical markers of asphyxia accurately predict later neurologic function. Isolated low 1-minute Apgar scores (see Apgar Score), transient moderate acidosis, or other metabolic abnormalities, in the absence of encephalopathy, do not correlate well with long-term outcome. Neurodevelopmental outcome is best predicted by severity of the encephalopathy. Sarnat and Sarnat related encephalopathy to neurologic outcome. In stage 1 (first 24 hours), the infant is hyperalert with unimpaired Moro and sympathetic reflexes and an electroencephalograph (EEG) reading that is abnormal. Stage 2 includes obtundation, hypotonia, hyperreflexia, and seizures. In stage 3, infants are stuporous, flaccid, and have abnormal brain stem and autonomic reflexes (Sarnat and Sarnat, 1976). These investigators reported normal outcome in infants who were in stage 2 for less than 5 days, but when the symptoms persisted for more than 7 days, severe neurologic injury occurred. The presence of stage 3 at any time during the clinical course was predictive of injury or death. Although variations on this scheme have been described, the general trends indicate that a severe neurologic syndrome in the neonatal period correlates with high mortality or significant functional impairment. Miller and associates (2004) developed a simple, quantifiable neonatal encephalopathy score based on the criteria identified by Sarnat and Sarnat (1976). Miller et al.’s (2004) encephalopathy score includes feeding, alertness, tone, respiratory status, reflexes, and seizure activity. The score obtained on day 1 and the maximum score from the first 3 days of life were correlated with outcomes of patients (who had neonatal encephalopathy) at 30 months of age. Similar to earlier reports, severe encephalopathy and seizures during the first 3 days of life predicted poorer outcomes.
After an initial period of stabilization after neonatal resuscitation, searching for multiorgan dysfunction is a priority, especially if the neonate has HIE. For example, myocardial ischemia may manifest as tricuspid insufficiency or hypotension (decreased contractility). Pulmonary hypertension may evolve (at times associated with meconium aspiration) and require invasive monitoring and ventilator support. Clearly, inadequate cardiorespiratory function may exacerbate HIE if the CO is reduced or hypoxemia persists. Concern for gastrointestinal ischemia often delays feeding of premature infants for 3 to 7 days because it is thought that this reduces the risk of NEC. However, recent data suggest that this may not be true (Schurr and Perkins, 2008). Severe hepatic insults can cause clotting abnormalities or disseminated intravascular coagulation (DIC) and predispose the newborn to hemorrhage. Finally, renal insufficiency has been associated with CNS injury (67% of patients studied) and death (33%) if oliguria persists longer than 24 hours (Perlman and Tack, 1988).
Apgar Score
The Apgar score was initially proposed as a means of rapidly assessing the status of newborns at 1 minute after birth and as a means of determining whether a neonate required respiratory support (Apgar, 1953) (Table 17-4). As Casey and others (2001) recently suggested, “Every baby born in a modern hospital in the world is looked at first through the eyes of Virginia Apgar.” The Apgar score includes five variables with a range of scores from 0 to 2 (for a maximum of 10 points): heart rate, respiratory effort, muscle tone, reflex irritability, and color. Currently, the score is applied at 1 and 5 minutes, but in some cases the evaluation continues for as long as 20 minutes if continued resuscitative efforts are required.
The Apgar score has been demonstrated recently to be a predictor of mortality (Casey et al., 2001). In full-term infants, these authors found a mortality rate of 244 per 1000 (24.4%) for infants with 5-minute Apgar scores of 1 to 3, compared with 0.2 per 1000 (0.02%) for infants with 5-minute Apgar scores of 7 to 10. Similarly, in preterm infants of 26 to 36 weeks’ gestation, the mortality rate was 315 per 1000 (31.5%) for infants with 5-minute Apgar scores of 0 to 3 and 5 per 1000 (0.5%) for infants with 5-minute Apgar scores of 7 to 10. Thus, the incidence of neonatal death was highest when the 5-minute Apgar scores were 3 or lower, independent of gestational age. Neonatal death most commonly occurred during the first day of life, with the majority of infants dying before 3 days of age. These data indicate that the Apgar score is a valid predictor of neonatal mortality. In fact, the Apgar score better predicted outcome than umbilical-artery pH of 7.0 or less. Combining a 5-minute Apgar of 0 to 3 with an umbilical artery blood pH of 7.0 or less increased the risk of death in both preterm and full-term infants. An Apgar score of 0 for longer than 10 minutes suggests that resuscitative efforts should be suspended (Jain et al., 1991). Papile (2001) stated, “At present, there is no single measure of the fetal or neonatal condition that accurately predicts later neurodevelopmental disability…but, few will deny [the Apgar score’s] application at 1 minute of age accomplishes Dr. Apgar’s goal of focusing attention on the condition of the infant immediately after birth.” Although outcomes vary with gestational age, with the etiology of neonatal depression, and with other factors, effective resuscitation of infants with low Apgar scores resulted in survival of about 40% to 60% of the patients and, approximately two thirds of survivors had normal neurologic function (Leuthner and Das, 2004). In 1964, the Collaborative Study on Cerebral Palsy reported a stronger relationship between the 5-minute Apgar score and neonatal mortality than the 1-minute score (Drage et al., 1964). Controversy about the Apgar score arises when people try to use the Apgar score to predict neurologic outcome. Dr. Apgar did not intend that the score be used to establish the diagnosis of asphyxia, to measure the severity of perinatal asphyxia, or to predict long-term neurologic outcome. In fact, 75% of children with cerebral palsy had normal Apgar scores at 5 minutes (Nelson and Ellenberg, 1981).
Pathophysiology of Hypoxic-Ischemic Injury
Asphyxia-related insults follow a pattern of early energy depletion and failure of the Na+, K+-ATPase pump, which disrupts normal transmembrane ion gradients. This loss of these gradients causes the release of excitatory neurotransmitters, such as glutamate, dopamine, serotonin, and aspartate. Although glutamate is important for normal brain development, excessive excitation of glutamate receptors (e.g., N-methyl-d-aspartate [NMDA], α-3-amino-hydroxy-5-methyl-4-isoxazole propionic acid [AMPA], and kainite) leads to excessive intracellular calcium concentrations and activation of a variety of phospholipases and proteases that cause the release of arachadonic acid and other mediators. Downstream production of xanthine and prostaglandins generates free radicals, in part via the NO synthase system. Although this is a condensed and simplified version of some of the pathways for cellular injury, the basic schema of hypoxic-ischemic injury is clearly one of disruption of membrane and intracellular function (Volpe, 2001). The clinical correlates of this intense cellular injury include seizures, abnormal mental status, eventual cell death, and long-term CNS deficits.
Neuroprotection
Gonzalez and Ferriero (2008) emphasized that treatment of neonatal brain injury should focus on both neuroprotection and after injury treatment. Optimal therapy may require intervening at multiple pathways to both prevent cell death and to induce cell growth and differentiation (Gonzalez and Ferriero, 2008). In general, therapies under evaluation attempt to decrease oxidative stress (e.g., inhibition of NO, melatonin, allopurinol, or deferoxamine), inflammation (e.g., minocycline), and excitotoxicity pathways (e.g., magnesium, MK-801, topiramate, or memantine). Other strategies include administering various growth factors, such as erythropoietin, a glycoprotein that has diverse CNS functions. These include vasogenic and immune responses that may provide neuroprotection during hypoxia and ischemia and modulate neurogenesis and neural differentiation. Primarily studies of VEGF in animals have shown that VEGF regulates both angiogenesis and neurogenesis. Cortical neurons are the primary site of VEGF-A activity early in development, but later its primary effect is on glial cells. VEGF seems to enhance the survival of neurons after hypoxia and after exposure to glutamate. Generating new neurons could play a major role in recovery after injury that results from perinatal stroke or hypoxia and ischemia.
Hypothermia is being used more often as a method of treating brain injury. It is hypothesized that hypothermia modifies apoptosis and necrosis of injured neurons by reducing their metabolic demands and by decreasing the release of excitotoxic mediators. Several studies have shown a significant reduction in CNS injury with cooling to 33° to 34° C for about 72 hours. Preliminary results suggested decreased mortality and improved neurodevelopmental outcome, although the differences in neurodevelopment after cooling are not large (Shankaran et al., 2005; 2008). Hypothermia plus another form of therapy may have greater therapeutic effect than either treatment alone (Alkan et al., 2001).
Seizures
Seizures occur commonly in neonates, especially after asphyxia (Silverstein and Jensen, 2007). Neonates with encephalopathy, stroke, intracranial hemorrhage, CHD, inborn errors of metabolism, and some syndromes are prone to develop seizures. Without an EEG, it may be difficult to tell if a neonate is having a seizure, because non–seizure-induced, repetitive movements are common. Cerebral palsy, low intelligence quotient (IQ), and epilepsy often accompany seizures and receptor expression, and neonatal seizures alter synaptic plasticity (Holden et al., 1982; Mellits et al., 1982; Swann and Hablitz, 2000 Stafstrom et al., 2006). Phenobarbital, diazepam, phenytoin, and valproate are commonly used drugs for the treatment of seizures, but these drugs can increase apoptotic neuronal death (Pereira de Vasconcelos et al., 1990; Schroeder et al., 1995). Furthermore, they fail to block neonatal seizures in as many as 50% of patients (Sankar and Painter, 2005). Hypoglycemia and hypocalcemia are other causes of seizures, and correcting these abnormalities usually terminates the seizures. The neonate’s Paco2 should be maintained within the normal preoperative range (not reduced), because alkalosis lowers the seizure threshold and may induce a seizure. Anesthesia obscures overt seizure activity, especially when paralytic drugs are used.
Perinatal Stroke
Perinatal stroke has been more commonly recognized during the past few years because of improved neuroimaging. The incidence is 1:2300 to 1:5000 infants born at term and 7:1000 in those born prematurely (Raju et al., 2007; Benders et al., 2008). Infants with CHD have significantly more ischemic perinatal strokes than other neonates (Sherlock et al., 2009). Approximately 40% of neonates with transposition of the great vessels (TGV) show evidence of preoperative stroke; an additional one third has evidence of a new stroke after cardiac surgery. Many of the patients who had a stroke before surgery underwent balloon septostomy to improve oxygen—the incidence of stroke is high after this procedure (McQuillen et al., 2006). Strokes are either detected in the first few days of life or after 28 days of age. Those detected early are found because patients have seizures or undergo an MRI. Despite the evidence of a stroke, many neonates have no signs of neurologic dysfunction. They may not show signs of focal lesions or encephalopathy (Miller, 2000). Perinatal strokes are classically located within the boundary of the area perfused by the middle cerebral artery, usually the left cerebral artery (Schulzke et al., 2005). The incidence of arterial perinatal stroke is similar in preterm neonates to that of term infants (de Veber et al., 1998). Cerebral venous thrombosis is another cause of perinatal strokes and is usually detected when the neonate develops seizures (Wu et al., 2003). Venous infarctions often become hemorrhagic.
The cause of a stroke in a given patient is almost never known. However, possible maternal factors include chorioamnionitis, prolonged rupture of the membranes, preeclampsia, and intrauterine growth retardation (Wu et al., 2004b). Twenty percent to 68% of infants with arterial ischemic stroke had prothrombotic states (Golomb et al., 2001). Polycythemia is also a cause of neonatal stroke, and suggested factors related to perinatal stroke include infection, inflammation, and hypoglycemia (Günther, et al., 2000; Benders et al., 2007).
Preoperative Neurologic Evaluation
There are some important questions to answer as part of the preoperative evaluation of the nervous system of neonates. Does the neonate breathe spontaneously and is the heart rate relatively stable and normal (120 to 160 beats per minute)? Is the fontanel normal (i.e., skin even with the outer table of the skull), bulging (from increased intracranial pressure), or sunken (from dehydration or volume depletions)? Is the muscle tone normal for the infant’s age? Differences in tone between the upper and lower extremities or between one side of the body or the other may suggest the presence of a neonatal stroke (Ibrahim et al., 2008). Does the infant bring his hands and arms together in front of the chest (Moro reflex) when the arms are raised and suddenly released? Is the Moro reflex equal bilaterally? Does the infant grasp the examiner’s finger when it is placed in a hand? When a finger is placed on the anterior sole of the foot, do the toes curl in a grasping motion? Is the Babinski reflex normal? Without experience it may be difficult to elicit deep tendon reflexes in neonates, especially preterm neonates. Do the eyes move normally when a flashlight is moved in different directions? The pupils should constrict to light, and there should be evidence of a gag reflex and a suck. A complete neurologic examination should be documented in the patient’s chart to note neurologic abnormalities that predate surgery and anesthesia.
Infants whose condition is stable except for ongoing neurologic problems (e.g., encephalopathy or seizures) require review of their vital signs, laboratory values, and medications, and they must have an appropriate physical examination. The hemodynamic responses to sedatives and analgesics and to painful procedures should be reviewed so the anesthesiologist can develop an anesthetic plan that will minimize wide variations in blood pressure. It should be determined whether the intravascular volume is adequate. Rapid administration of intravenous fluid during induction of anesthesia should be avoided because doing this may suddenly increase arterial and intracranial pressures, which can cause intracranial hemorrhage, especially in preterm neonates. The intracranial hypertension and increased intracranial volumes occur because cerebrovascular autoregulation is abnormal or absent. Both hypertension and hypotension can exacerbate neurologic injury. If available, near-infrared spectroscopy (NIRS) or EEG data should be reviewed. Do feeding, suctioning the trachea, medications (e.g., sedation), and fluid therapy alter cerebral oxygenation (Baserga et al., 2003)? If the patient is mechanically ventilated, understanding how oxygenation and ventilation are affected by various interventions may provide clues to the best ventilatory strategy to employ during surgery.
Postanesthetic Apnea
Preterm and expremature infants undergoing elective surgery are prone to develop postoperative apnea (Steward, 1982; Gregory and Steward, 1983). Apnea is usually defined as cessation of breathing for 20 seconds or more. The likelihood of life-threatening postoperative apnea increases if the patient had apneic spells in the NICU (Liu et al., 1983). Twenty percent to 30% of preterm infants have apnea with cyanosis and bradycardia during the first month of extrauterine life. Steward (1982) reported the presence of apnea in 18% of preterm infants (with a gestational age of fewer than 38 weeks or a postnatal age of 3 to 28 weeks) during the first 12 hours after surgery.
A prospective study by Liu et al. (1983) found that a history of apnea and a postconceptual age of fewer than 44 weeks often resulted in apnea after surgery. Kurth and LeBard (1991) reported that postanesthetic apnea (defined as cessation of breathing for more than 15 seconds, not longer than 20 seconds) occurred commonly in expremature infants whose postconceptual ages varied from 32 to 55 weeks. The initial episode of apnea occurred as late as 12 hours after the termination of anesthesia. These authors recommended that infants who whose postconceptual age was younger than 60 weeks and who developed apnea after surgery be continuously monitored in the hospital until they were apnea free for at least 12 hours.
Welborn et al. (1986) found no evidence of apnea in a group of healthy premature infants who had a postconceptual age of 44 weeks or more at the time of surgery and who underwent general anesthesia for herniorrhaphy. Although many of these patients did not have apnea, 63.6% of the them had periodic breathing, a common finding in premature infants that is characterized by repetitive apneas of short duration (fewer than 5 to 10 seconds) without oxygen desaturation or cyanosis and is usually harmless. Malviya et al. (1993) noted that expremature infants younger than a postconceptual age of 44 weeks had significantly more apnea than older infants. Coté et al. (1995), using data from 255 patients garnered from previously published studies, found an incidence of apnea of 1% in infants of 55 to 56 weeks’ postconceptual age. They also found an inverse relationship between postconceptional age and the incidence of apnea. Those who were 56 weeks’ postconceptual age or younger had the greatest risk for apnea. Anemia (Hct < 30%) was also an important risk factor for apnea (Fig. 17-10).
Nearly all patients in these studies of postoperative apnea in prematurely born infants in the past were anesthetized with halothane or isoflurane, drugs that have higher blood-gas solubility coefficients than newer inhaled anesthetics such as sevoflurane and desflurane. These less soluble agents have been associated with a lower incidence of apnea in patients who do not have a history of preoperative apnea (William et al., 2001).
Other factors that predispose infants, especially premature infants, to apnea include hypoglycemia, hypoxia, hyperoxia, sepsis, anemia, hypocalcemia, and changes in the environmental temperature (Schulte, 1977). Postoperative apnea may also be the result of the interaction of general anesthesia with an immature CNS (including the respiratory center). For example, in low concentrations, halothane depressed the chemoreceptor responses to hypoxia (Knill and Gelb, 1978). Inhaled anesthetics also depressed intercostal muscles function, thus reducing the FRC and increasing the risk of hypoxemia (Tusiewicz et al., 1977). These differences, coupled with an immature response to hypoxia and hypercarbia, predispose premature infants to erratic respiratory responses in the perioperative period (Rigatto et al., 1982).
Residual anesthesia in the postoperative period may also be an important factor in the development of apnea in preterm babies. This may be especially true when halothane, with its high lipid solubility, was the standard anesthetic in pediatric anesthesia. The cartilaginous upper airway of preterm infants may also predispose them to upper airway obstruction, and this obstruction may be made worse by the effects of residual anesthesia on pharyngeal muscles (Dransfield et al., 1983). Elective surgery should be delayed, if possible, until former preterm infants are beyond 44 weeks’ postconceptual age (Gregory and Steward, 1983). Infants younger than 44 weeks’ postconceptual age who require surgery must be individually evaluated. The type of surgery, the patient’s gestational and postconceptual age, hematocrit, current cardiorespiratory function (i.e., oxygen or diuretic dependence, CLD, neurologic status) are all factors that must be taken into consideration when developing a perioperative plan for the expremature infant. These patients should be admitted to hospital for 24 to 36 hours for postoperative monitoring.
Thermoregulation
Newborns abruptly arrive into an environment that is approximately 20° C cooler than the one they just left. Living in this new, colder environment markedly increases the number of calories expended to regulate body temperature. Once born, neonates lose heat by evaporation, convection, conduction, and radiation. Extremely preterm infants can lose 15 times more heat by transdermal water loss than full-term babies (Hammarlund et al., 1979). To compensate for increased heat losses, the sympathetic nervous system constricts skin blood vessels, which centralizes body heat (Asakura, 2004). Oxygen consumption and cell metabolism increase two- to threefold.
Many factors are responsible for heat loss, including cold environments (e.g., delivery rooms), a high ratio of surface area-to-body weight, reduced subcutaneous fat, and an underdeveloped ability to shiver in response to cold. Shivering is limited in part by the neonate’s much smaller muscle mass (25% vs. 45% in the adult). The major compensatory mechanism for cold stress in neonates is nonshivering thermogenesis (NST). NST is the result of stimulation of triglyceride and fatty acid metabolism in energy-rich brown fat by norepinephrine and thyroid hormone (Stern et al., 1965). Brown fat, which is mostly deposited during the third trimester of pregnancy, is found between the scapulae and around major abdominal organs. Infants born before the third trimester have less ability to initiate NST and are prone to hypothermia. SGA neonates have NST but to a far lesser degree than AGA term infants.
Hypothermia (body temperature of less than 36° C) increases oxygen consumption, metabolic acidosis, and pulmonary and peripheral vascular resistance while reducing CO (Brady and Ceruti, 1966). Babies admitted to the NICU with a temperature of 35.5° C have a Pao2 that is on average 18 mm Hg lower than neonates whose body temperatures are equal to 36.5° C. Warming cold infants to 36.5° C or higher increased Pao2 (Stephenson et al., 1970). Neonates should reside in a neutral thermal environment (i.e., the environmental temperature at which their oxygen consumption is minimal). Normally, this occurs when the skin temperature is 36° C and the environment temperature is between 32° to 34° C (see Chapter 6, Thermoregulation: Physiology and Perioperative Disturbances, Fig. 6-2) (Adamson and Towell, 1965). Prematurity, hypoglycemia, and general anesthesia exaggerate the neonate’s poor metabolic response to hypothermia (Swyer, 1975). Because preterm and asphyxiated or neurologically injured full-term neonates have limited ability to maintain normal body temperatures, their environmental temperature should be carefully controlled with overhead heaters, isolettes, and warm operating rooms.
There are several methods of maintaining a neonate’s body temperature, including covering the body with clear plastic, covering the head with a hat, placing a warming pad under the infant, increasing the environmental temperature, and wrapping the infant in warm blankets (see Chapter 6, Thermoregulation: Physiology and Perioperative Disturbances).
Maintenance of a normal body temperature is crucial, because hypothermia increases PVR, decreases pulmonary blood flow and causes right-to-left shunting of blood through the foramen ovale or PDA (see Transitional Circulation, p. 523, and Chapter 6, Thermoregulation: Physiology and Perioperative Disturbances, Fig. 6-8) (Brady and Ceruti, 1966; Stephenson et al., 1970).
Prolonged exposure to a hypothermic environment places demands on neonates that may exceed their ability compensate for these demands. If they cannot do so, hypoventilation, inadequate oxygen delivery, tissue acidosis, and cardiovascular collapse may occur (Adamsons et al., 1965).
The neonate’s temperature can be maintained by wrapping the trunk and extremities with plastic wrap, using stockinet caps, and caring for the child in incubators and overhead heaters (Roberts, 1981; Vora et al., 1999). Other than a cap, it is difficult to use any of these devices in the operating room because their use makes it difficult or impossible to do the operation.
Overhead heaters allow access to the patient and are commonly used during the first few days of life and in the operating room before the surgical drapes are applied. Beside allowing access to the patient, these devices effectively maintain the baby’s body temperature. Compared with incubators, overhead warmers increase insensible water loss by about 0.94 mL/kg per hour or 22.6 mL/kg per day (Flenady and Woodgate, 2003). More overhead warmer-treated patients had a serum sodium of greater than 150 mEq/L, compared with incubator-treated infants (Meyer et al., 2001). This increases the daily fluid requirements. The oxygen and energy consumption and the incidence of bradycardia are increased in overhead warmer-treated neonates (Long, 1980; Gorski et al., 1990; Hutchison, 1994). Nurses interact more often with infants who have been cared for in an overhead warmer than with those who were cared for in an incubator, which increases the opportunity for infections (Davenport, 1992). There are no reported differences in the rate of NEC, IVH, PVL, or death associated with overhead warmers and incubators.
Infants cared for in incubators have less insensible fluid loss, lower fluid intake, and lower body temperatures on the first 2 days of life. However, no difference in weight gain, maximum serum sodium, or serious complications (e.g., NEC, PVL, or ROP) was found in infants treated in the incubator vs. the overhead warmer (Meyer et al., 2001). More infants in the overhead-warmer group required phototherapy for hyperbilirubinemia. When evaluating neonates for surgery, anesthesiologists must be aware that patients cared for in overhead warmers may be hypovolemic more often than patients cared for in incubators and require correction of their intravascular volume preoperatively.
Liver
During the third to fourth week of gestation, epithelium of the posterior foregut forms an outpouching (liver bud) that invades the mesenchymal cells of the septum transversum of the diaphragm. Cells from both structures form hepatocellular tissue that is separated by sinusoids. The blood supply of the developing liver originates from the yolk sac. These vessels eventually become the hepatic and portal venous systems (Mitchell and Sharma, 2009). A connection between the hepatic bud and the duodenum forms the common bile duct, and an outgrowth from the common bile duct forms the gallbladder and the cystic duct. Endodermal cells occlude the extrahepatic bile ducts for the first 3 months of gestation. Failure to recannulate the ducts around this time results in extrahepatic biliary obstruction (i.e., biliary atresia), a common cause of liver failure during the first year of life. By the fourth week of gestation, hepatocytes produce and secrete some proteins, including α-fetoprotein and α1-antitrypsin (Diehl-Jones and Askin, 2002). By the fifth week of gestation, hematopoietic stem cells are present in the liver, and these cells are the primary source of hematopoiesis throughout the first two thirds of gestation; the bone marrow assumes this role during the third trimester. To accomplish hematopoiesis, the liver mass increases fortyfold, and in early gestation, hematopoietic cells outnumber hepatocytes. Early in gestation hepatic cells differentiate into type II hepatocytes and intrahepatic bile ducts. Failure to develop intrahepatic ducts is another major cause of liver failure during infancy. Formation of the Golgi apparatus permits synthesis and secretion of albumin and other proteins. By the second month of gestation, bile is secreted. Glycogen synthesis occurs by the tenth week of gestation, and acini are present by the third gestational month. The umbilical vein, not the portal vein, supplies most of the blood flow to the left (90%) and the right (60%) lobes of the liver in utero (Bloom and Fawcett, 1975).
Glucose Metabolism
The fetal supply of glucose is abruptly interrupted at birth, requiring neonates to meet their glucose needs by glucose intake, by converting glycogen (which is stored in the liver and heart during the third trimester of pregnancy) to glucose, and by glycogenolysis. Glycogen breakdown is under the control of catecholamines and glucagon. The liver of infants born at term has a greater store of glycogen than the adult (Kalhan and Parimi, 2000). Glycogenolysis allows full-term infants to maintain normal serum glucose concentrations during a 10 to 12 hour fast. Since glycogen storage and the capacity for degradation mostly occur during the last trimester of pregnancy, preterm infants are predisposed to hypoglycemia. Gluconeogenesis is not active in fetal liver, but a variety of hepatic enzymes that are important in gluconeogenesis (e.g., glucose-6-phosphatase) undergo rapid development after birth (Kalhan and Parimi, 2000; Kalhan et al., 2001).
Biotransformation
Cytochrome P450 (CYP) enzymes are responsible for phase I processes. More than 50 CYP enzymes have been identified, and they are classified according to their nucleotide sequence (Wilkinson, 2005). Although the full clinical relevance of the relative expression of these enzymes in fetus and neonates is yet to be defined, their primary expression is in the liver, and their developmental expression has a major role in the pharmacokinetics and pharmacodynamics of drugs, including those used during anesthesia. The CYP3A family is responsible for metabolism of about 50% of all drugs (Hines and McCarver, 2002). Multiple patterns of developmental expression have been reported (Figs. 17-11 and 17-12). For example, although CYP3A7 is present in utero, its expression is negligible 1 week after birth. On the other hand, the concentration of CYP3A4 is low during fetal life but is about 50% of adult levels by 6 months of age. CYP2D6 has a multitude of polymorphisms and may be of particular relevance in the metabolism of psychotropic (and anesthetic) drugs (Blake et al., 2005). Clearly, as the ontogeny of metabolizing enzymes and transporters is understood, the dosing of drugs will be better adjusted for age-related differences, toxicity may be avoided, and clinical effectiveness will be improved.
Fewer data are available concerning the ontogeny of phases II and III. However, the uridine glucuronosyltransferases (UGTs) function at much lower levels in neonates than they do in adults. For example, glucuronidation of morphine reaches adult levels only at 2 to 6 months of age (de Wildt et al., 1999). Other conjugating enzymes with major roles in drug metabolism have been less well characterized (glutathione S-transferases, N-acetyltransferases, glucuronosyl transferases), but they are likely to play important roles in the unique drug handling commonly seen in the newborn.
Although the clinical implications of the genetic variability and developmental changes of hepatic metabolizing processes are not clearly identified, examples of age-related differences in drug responses are well documented. For example, the age-related pharmacokinetics of various muscle relaxants and narcotics were described more than 20 years ago (Cook, 1981; Fisher et al., 1982; Gauntlett et al., 1988). Caffeine and theophylline are metabolized by CYP1A2, which is expressed at low levels in the newborn and requires age-related considerations for therapeutic dosing (Hakkola et al., 1994). More recently, both postnatal age and postconceptual age were correlated with decreased and variable propofol clearance in preterm and full-term infants. Of note, multiple cytochrome P450 enzymes are required for metabolism of this drug. Consequently, altered expression of phases I and II hepatic metabolic enzymes may be responsible for these pharmacokinetic findings (Allegaert et al., 2007).
Coagulation
The fetus produces coagulation factors because they inefficiently cross the placenta. Plasma levels of some coagulation proteins and factors and laboratory tests of clotting function (e.g., prothrombin time [PT] and activated partial thromboblastin time [APTT]) are markedly different in healthy full-term and preterm infants than in adults (Table 17-5).
TABLE 17-5 Normal Values for Clotting Tests Appropriate for Gestational Age (AGA), Small for Gestational Age (SGA), and Premature Infants During the First Day of Life
Plasma concentrations of vitamin K–dependent proteins (e.g., factors II, VII, IX, and X), as well as factors XI and XII, prekallikrein, and kininogen, are about 50% lower than those of adults, but the concentrations of fibrinogen, factor V, and factor VIII are similar to those of adults (Andrew et al., 1987; 1988).
Disturbances in clotting caused by liver dysfunction correlate with decreased synthesis of both clotting and fibrinolytic factors and with abnormal platelet function. Hepatocytes synthesize factors 1 (fibrinogen), II (prothrombin), V, VII, IX, and X, and abnormal production of these factors is reflected in a prolonged PT. The PT mostly reflects availability of factor VII. Thrombin levels of newborns are about 50% those of adults. APTT primarily reflects the amount of thrombin generated (Green et al., 1976).
In spite of differences between newborn and adult liver function, clinically significant bleeding is uncommon in normal neonates who have adequate vitamin K levels. The association of vitamin K deficiency and hemorrhage in the newborn was initially described in 1894, is secondary to inadequate activity of vitamin K–dependent coagulation factors (i.e., II, VII, IX, and X), and is corrected by vitamin K replacement (Sutor et al., 1999). On the other hand, the newborn is predisposed to bleeding by infections and asphyxia, which may cause DIC. DIC is associated with prolonged PT and APTT that are caused by depletion of certain coagulation factors (e.g., fibrinogen and factors V and VIII), with increased fibrin degradation products, and thrombocytopenia.
Hyperbilirubinemia
Increased serum bilirubin concentrations are almost universal during the first postnatal week. (Kahlan and Parini, 2000). In most cases, hyperbilirubinemia is transient and has been labeled physiologic. The primary source of bilirubin is hemoglobin, but other heme-containing proteins (e.g., cytochromes, catalases, and myoglobin) also contribute. Infants produce more bilirubin per kg of body mass than older patients because they have higher red cell masses and their red blood cells have shorter life spans. Bilirubin is the end product of hemoglobin breakdown and has also been reported to be an antioxidant and a free-radical scavenger (Sedlak and Snyder, 2004).
Because of its large nonpolar structure, bilirubin is lipophilic and requires biotransformation for excretion. Binding of bilirubin to albumin facilitates its delivery to the liver, and uptake of bilirubin into hepatocytes for conjugation requires glucuronic acid (i.e., organic anion transporter, [OATP2]) (Cui et al., 2001). Uridine diphosphate glucuronic acid provides the glucuronic acid. Most bilirubin is excreted in bile as bilirubin diglucuronides. Upon entry into the intestinal lumen, a variety of pathways exist for further disposal of bilrubin. As occurs in adults, bacteria metabolize bilirubin to urobilinogens for excretion in feces. Since the neonatal gastrointestinal tract lacks the same bacterial flora as adults, bilirubin may be reabsorbed and raise the serum bilirubin concentration. Beta-glucuronidase is also important for bilirubin metabolism, because it deconjugates bilirubin. Although this enzyme clears unconjugated bilirubin from the placenta, it increases bilirubin reabsorption from the gut after birth. Breast milk contains high level of β-glucuronidase and is partly responsible for “breast-milk jaundice” (Gourley and Arend, 1986).
Even if significant hemolysis occurs in utero, the normal placenta efficiently clears the bilirubin, and the newborn is seldom jaundiced. However, after birth the same bilirubin production would cause jaundice in most infants. With increasing bilirubin concentrations, the skin becomes progressively yellow (icteric) in a cephalocaudad pattern (Knudsen, 1990). The term physiologic jaundice refers to the common, transient, indirect (unconjugated) hyperbilirubinemia in normal newborns. However, jaundice should be considered abnormal if it is present in the first 36 hours of life, persists beyond 10 days of life, is greater than 12 mg/dL, and the direct (conjugated) bilirubin is greater than 2 mg/dL or more than 30% of the total bilirubin concentration. Multiple disorders of increased bilirubin production (e.g., hemolysis secondary to maternal-fetal blood group incompatibility, other hemoglobinopathies, reabsorption of red blood cells from a hematoma, and polycythemia) or decreased excretion (e.g., as with genetic diseases, hypothyroidism, or infants of diabetic mothers) are recognized. Preterm infants more commonly develop elevated levels of bilirubin and are more vulnerable to bilirubin-induced CNS toxicity. CNS toxicity, or kernicterus, results from hyperbilirubinemia. Neuronal necrosis is the predominant feature and occurs mostly in the basal ganglia, brainstem, occulomotor nuclei, and cochlear nuclei.
Renal Function
Metabolic stability and electrolyte homeostasis are maintained in utero by the placenta; renal growth and development appear not to be linked or regulated by function during this time. Permanent kidneys appear during the fifth week of gestation, and nephrons appear during the eighth week, initially in the juxtamedullary region and cortex. A complex interaction of genes, such as Wilms’ tumor gene 1 (WT1), and growth factors such as neurotrophic factor, (GDNF) orchestrate this process (Taeusch et al., 2005; Dziarmaga et al., 2006). By 20 weeks’ gestation, one third of the final number of nephrons is present, and by 35 to 36 weeks’ gestation, the adult number of nephrons is present (see Chapter 5, Regulation of Fluids and Electrolytes) (McDonald and Emery, 1959). During the second half of gestation, kidney growth is in direct proportion to gestational age. Infants born prematurely develop new nephrons until about 34 to 35 weeks’ postconceptual age. Once the full complement of nephrons is present, further maturation of the kidneys occurs by increases in both glomerular and tubular size. Vascular growth and development parallel nephrogenesis. Anesthesiologists must understand renal development because of the kidneys’ importance in maintaining acid-base homeostasis and fluid and electrolyte balance (see Chapter 5, Regulation of Fluids and Electrolytes).
Among healthy full-term infants, the number of nephrons varies up to fivefold (Merlet-Bénichou et al., 1999). Recent studies suggest that having fewer nephrons at birth correlates with hypertension later in life (Brenner et al., 1988; Keller et al., 2003). These authors suggest that hypertrophied, overworked nephrons slowly sclerose, leading to progressive renal dysfunction. The reduced number of nephrons is thought to result from both genetic and environmental factors (Lelièvre-Pégorier and Merlet-Bénichou, 2000). Recently, polymorphism of the RET gene was shown to reduce the number of nephrons (Zhang et al., 2008). A common variant of another gene, PAX2, is also associated with having smaller kidneys at birth (Quinlan et al., 2007). Both prematurity and fetal growth retardation have negative effects on postnatal renal growth (Huang et al., 2007; Rakow et al., 2008). Finally, oxidative injury during the neonatal period has been associated with decreased capillary density and fewer nephrons in adult rats (Yzydorczyk et al., 2008).
Urine is formed by 10 weeks’ gestation, and urine production increases from about 2 to 5 mL/hour at 20 weeks’ gestation to 10 to 12 mL/hr at 30 weeks’, 12 to 16 mL/hr at 35 weeks’, and to 35 to 50 mL/hr at 40 weeks’ gestation (Rabinowitz et al., 1989). Because the fetal kidneys process large volumes of fluid, large quantities of hypotonic urine are produced. Fetal urine production is essential for the maintenance of normal amniotic fluid volumes, especially after 18 weeks’ gestation. Oliguria causes oligohydramnios, which is associated with a specific facies, clubfeet, limb contractures, and in some cases, pulmonary hypoplasia. In essence, high fetal urine volumes are necessary for normal development of organs besides the urinary tract.
Fetal urine is initially hypotonic (100 to 250 mOsm/kg). Its tonicity decreases throughout gestation. For example, urinary sodium is 120 mEq/L at midgestation and decreases to 50 mEq/L by 32 to 35 weeks’ gestation (Spitzer, 1996). Fetal and neonatal renal function is characterized by low renal blood flow, glomerular filtration rate (GFR), solid excretion, and concentrating power. Renal blood flow is low in utero because renal vascular resistance is high. After birth, renal blood flow increases markedly as a result of an increase in the arterial blood pressure and a decrease in renal vascular resistance. These changes allow more of the CO to be distributed to the kidneys (2% to 4% in utero, 10% at 1 week of age, 25% in the adult). Renal blood flow is about 20 mL/min per 1.73 m2 at 30 weeks’ gestation, 45 mL/min per 1.73 m2 at 35 weeks’ gestation, about 80 mL/kg per 1.73 m2 at term, 250 mL/min per 1.73 m2 at 8 days after birth, and 770 mL/min per 1.73 m2 at 5 months of age (Heisler, 1993). Similarly, fetal GFR increases rapidly in utero as the number of nephrons increases. Because fetal kidney growth begins deep in the medulla, the juxtamedullary nephrons are more mature than other nephrons at birth and have greater tubular length than outer and inner cortical nephrons. Since the glomerulus is uniform, a “tubular-glomerular” imbalance exists. This imbalance explains, at least in part, why more of the solids presented to the proximal tubules of newborns are not reabsorbed.
The GFR of preterm infants is a function of both gestational and postnatal age. During the first 24 hours of extrauterine life, the GFR of infants born before 25 weeks’ gestation may be as low as 2 mL/min per 1.73 m2. Infants born between 25 and 28 weeks’ gestation have a GFR of 10 to 13 mL/min per 1.73 m2, and those born after 34 weeks’ gestation have one of 20 to 25 mL/min per 1.73 m2, which is similar to that of full-term infants (Svenningsen and Aronson, 1974). Although GFR increases at a slower rate in ELBW infants, all neonates without acquired renal insufficiency double their GFR by 2 weeks of age and triple it by 3 months of age. Thereafter, GFR increases more slowly. Adult values for GFR are reached by 12 to 24 months of age. Because there is rapid renal maturation after birth, a 3-week old infant with 27 weeks’ gestation may have significantly more mature renal function than a healthy 6-hour-old full-term infant. Renal maturation apparently occurs in response to demand (separation from the placenta plus solute exposure). Renal filtration and concentrating ability increase when the kidneys are challenged.
The serum creatinine concentration is commonly used as a measure of glomerular function. At birth, it reflects maternal values and is higher than that of normal 1- to 2-week-old full-term neonates (0.4 mg/dL). For the first 4 weeks of life, the plasma creatinine concentration of preterm infants exceeds that of full-term infants (Bueva and Guignard, 1994). Interestingly, the serum creatinine concentration was the same at birth in infants born before 27 weeks’ gestation as it was in those born at 31 to 32 weeks’ gestation. Serum creatinine concentrations increased in all groups over the first 3 days of life and then gradually decreased to less than 0.5 mg/dL (Gallini et al., 2000). However, the maximum creatinine concentration reported was higher and occurred later (day 3.5 vs. day 1) in the most immature neonates. Creatinine clearance increased in all groups, but it increased more slowly in the infants who had fewer than 27 weeks’ gestation. The variability in GFR and creatinine clearance, as a function of gestational and postnatal age, means that drugs primarily eliminated by the kidneys may be eliminated differently in different neonates.
Renal Tubular Function
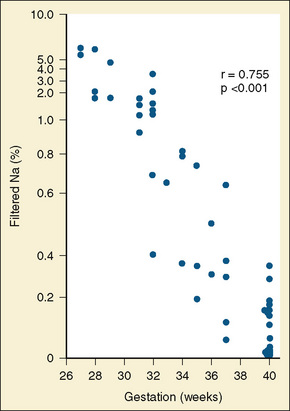
FIGURE 17-13 Scattergram demonstrating the inversion correlation between fractional sodium excretion and gestational age.
(From Siegel SR, et al.: Renal function as a marker of human renal fetal maturation, Acta Paediatr Scand 65:481, 1976.)
After birth, reabsorption of sodium in the proximal tubules increases five- to tenfold in response to increased Na+, K+ -ATPase activity (Holtbäck and Aperia, 2003). Glucocorticoid hormones increase messenger ribonucleic acid (mRNA) for both subunits of this enzyme, and prenatal administration of betamethasone to mature the lungs may also mature renal function. Similarly, developmental changes in the Na+–H+ exchanger (NHE) may be responsible for differences in acid-base balance among newborns, older children, and adults. The kidneys both reabsorb bicarbonate and excrete fixed acids. Bicarbonate reabsorption occurs primarily in the proximal tubules, but it also occurs in the loop of Henle and the collecting ducts. Hydrogen is actively secreted, and the secreted hydrogen reacts with bicarbonate to produce carbonic acid and carbon dioxide. These substances enter the tubular cells through the action of carbonic anhydrase. Bicarbonate again forms and exits the cell via another transport mechanism. Several isoforms of NHE have been identified, and impaired function of this transporter has been described (Rodríguez-Soriano, 2000; Holtbäck and Aperia, 2003). Immature carbonic anhydrase activity and impaired NHE function explain in part the normal metabolic acidosis found in newborns.
Distribution of total body water changes throughout fetal life (Friis-Hansen, 1983; Brans, 1986). At 16 weeks’ gestation, the total body water makes up 94% of the fetus’ weight; at about 32 weeks’ gestation it is 82%, and at term it is approximately 75%. The size of the extracellular compartment decreases from 65% at 16 weeks’ gestation to about 60% at 24 to 25 weeks’ gestation, to about 50% at term. At the same time the intracellular compartment increases from 34% in early gestation to 50% at term. Of note, most extracellular water is in the interstitial space (i.e., an extracellular compartment). This is exaggerated in preterm neonates, especially ELBW neonates, whose extracellular water compartments are very large.
During the first 3 to 7 days of extrauterine life, healthy term infants lose about 5% to 10% of their body weight, primarily through contraction of the extracellular water space (Fig. 17-14). Preterm infants follow a similar pattern, but they may lose more than 15% of their body weight; premature infants also take longer to establish steady growth than full-term infants do. Cardiorespiratory abnormalities and their treatment, infections and their side effects, and pharmacologic interventions so alter growth patterns that it is difficult to define normal growth patterns in preterm neonates.
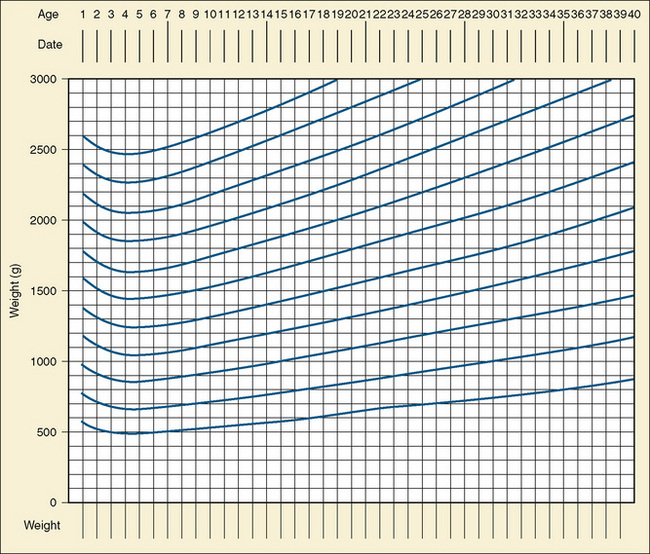
FIGURE 17-14 Extrauterine growth chart according to gestational age in weeks.
(Data from Schaffer SG, Quimiro CL, Anderson JR, Hall RT: Postnatal weight changes in low–birth-weight infants, Pediatrics 79:702, 1987; Gleason CA, Taeusch HW, Ballard RA, editors: Avery’s diseases of the newborn, ed 8, Philadelphia, 2005, Saunders, p. 1575.)
Hematology
Developmental hematopoiesis occurs in three stages and locations—the embryonic yolk sac, the fetal liver, and the bone marrow (Sacher and McPherson, 1986; Rappaport, 1997). Each stage is critical to survival.
Jopling et al. (2009) reported an increase in hemoglobin concentration from 14 g at 25 weeks’ gestation, 16 g at 30 weeks, 17 g at 35 weeks, to 18 g at term in AGA neonates. A similar increase occurred in the hematocrit. The increases of hemoglobin and hematocrit were linear and not affected by gender. SGA infants have higher hemoglobin concentrations than full-term babies 3 hours after birth, and the concentration decreases about 10% over the first week of life. The higher hemoglobin concentration of SGA neonates is thought to be compensation for intrauterine hypoxia and elevated levels of erythropoietin (Snijders et al., 1993). Preterm infants have lower hemoglobin concentrations at birth than SGA or AGA infants, and the decrease in hemoglobin concentration is greater in premature infants after birth (Table 17-7) (Obladen et al., 2000).
TABLE 17-7 Normal Hematologic Values for Appropriate for Gestational Age (AGA), Small for Gestational Age (SGA), and Premature Infants
The true hemoglobin of preterm infants may be difficult to determine, because many of these infants have intrauterine hypoxia before birth. This may stimulate hemoglobin production, as may steroid administration to the mother. The production of new erythrocytes decreased after birth as a result of lower concentrations of erythropoietin and fewer reticulocytes. Many preterm infants fail to respond to erythropoietin, because they have iron deficiency (Obladen et al., 2000). However, low iron concentrations may protect them from free-radical injury (Khawaja and Volpe, 2008).
The white blood count of neonates is variable at birth, especially in neonates who receive betamethasone or are infected in utero (Table 17-7) (Cohen et al., 1993). Some healthy infants have white blood cell (WBC) counts of 25,000 at birth. On the other hand, 21% of SGA neonates are neutropenic at birth but have normal cell counts by 7 days after birth (Ozyürek et al., 2006).
One third of SGA infants are thrombocytopenic on the first day of life, possibly because of consumption of platelets by placental infarcts. These infarcts may also be responsible for the polycythemia of SGA neonates. The platelet count of normal SGA infants is 102 to 292 × 109, and is usually greater than 250 × 109 after a few days (Table 17-7).
Preoperative Hematologic Evaluation
Several important questions must be answered during the preoperative evaluation. Is there evidence of bleeding? Are there petechiae or skin hemorrhages? Is there, or has there been, recent active bleeding into the bowels, lungs, or the brain? What is the platelet count, and has it decreased over the previous 24 hours? Were platelet transfusions required to maintain the desired platelet count, usually greater than 100,000/mm3? Are PT and PTT normal? If not, has the patient been treated with FFP? How many times? How much did FFP improve the PT, PTT, and international normalized ratio (INR)? For how long does it remain improved (normal)? When was FFP last administered? What is the WBC count? Is it increased or decreased from normal? Does an increase or decrease in the WBC count occur with sepsis? What are the patient’s hemoglobin concentration and hematocrit value? Are they high? If so, is this because of overtransfusion or dehydration? Is the hematocrit value low? If so, is the low hematocrit value caused by bleeding or by excessive blood drawing? Is blood available for the patient in the blood bank? How many units have been cross-matched against the child? How old is the blood? Blood that has been in the blood bank for more than a few days has an elevated potassium concentration, often in excess of 10 mEq/L. If this blood is administered rapidly to replace blood loss in neonates, a potassium-induced cardiac arrest may occur, which may be difficult to treat (Brown et al., 1990). Because many infants have potassium concentrations of 5 mEq/L or more, rapid addition of potassium during blood transfusion may be disastrous or lethal.