Chapter 46 Neonatal Respiratory Disease
Effective gas exchange within the lung requires both adequate ventilation and perfusion. Determinants of ventilation include the ventilatory “pump” (e.g., central drive, muscle strength, and chest wall recoil),1 compliance of the lung and chest wall, and resistance to airflow within the airways. Determinants of perfusion include the circulatory pump (right ventricular output) and pulmonary vascular resistance. A disorder in any one or a combination of these determinants can lead to respiratory insufficiency. Clearly then, a variety of disorders, either pulmonary or nonpulmonary, can lead to respiratory insufficiency.
Acute or Early-Onset Respiratory Disorders
Delayed Clearance of Fetal Lung Liquid
Fetal lung fluid, which is actively produced by the lung and is critical for normal fetal lung development,2 must be readily cleared from the airways to allow normal breathing after birth. On the basis of results from animal studies, the relative volume of liquid within potential airspaces, which remains constant in utero, is approximately 20 to 30 mL/kg near term and is the result of a balance between net accumulation within the lung (production minus reabsorption) and efflux out the trachea into the amniotic cavity.3 In the hours to days before delivery, net accumulation diminishes, and during labor, reabsorption predominates.4 As a result, extravascular lung liquid (i.e., liquid within the airspaces and interstitium) decreases. Any excess fluid remaining within the airspaces at the time of delivery is further removed as air entry into the lung displaces liquid from the airways into the interstitium. Residual liquid within the interstitium is then taken up into the circulation during the next several hours. Excessive extravascular liquid will lead to impairment of gas exchange, interstitial liquid pressure can compress small airways leading to atelectasis and gas trapping, and excess liquid within airspaces will impair alveolar gas exchange.5
Fetal lung liquid production and reabsorption are the result of active ion transport6 and are presumably hormonally regulated (Figure 46-1). Chloride ions enter the lung epithelial cell across the basolateral membrane via a Na/K/2CL cotransporter (the transporter on which furosemide acts). The mechanism of transepithelial movement of lung fluid at the time of birth is passive movement of sodium through epithelial sodium channels (ENaC), which are closed during fetal life; adrenergic stimulation during parturition activates these channels.7 Although β-adrenergic agents such as terbutaline and epinephrine enhance Na ions and thus liquid reabsorption,7,8 β-adrenergic blockade does not inhibit the reabsorption of lung liquid during spontaneous labor and delivery in animal studies.9,10 Other hormones of parturition, such as vasopressin, may be important as well.11,12
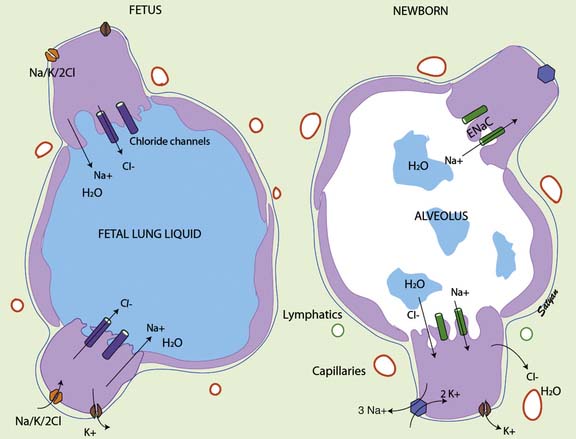
Figure 46–1 Mechanism of fetal and neonatal lung fluid transport.
(Modified from Guglani L, Lakshminrusimha S, Ryan RM: Transient tachypnea of the newborn, Pediatr Rev 29:e59-e65, 2008. Copyright Satyan Lakshminrusimha.)
The pulmonary circulation is also a key factor in fetal lung fluid clearance; not only does interstitial liquid drain directly into the circulation,13,14 but the dramatic increases in pulmonary blood flow seen after birth may enhance reabsorption of liquid from fetal airspaces.15 The onset of breathing not only increases the surface area for liquid reabsorption but also is associated with the opening of pores through which liquid can readily enter the interstitium.16 Drainage of interstitial liquid is generally complete by the end of neonatal transition (4 to 6 hours). Interstitial liquid appears to be directly absorbed into the microcirculation, and this process is governed by Starling forces; the contribution of lymphatic drainage is negligible.3
Interference with this process of liquid removal from the airspaces and interstitium leads to impaired gas exchange and respiratory distress with a variable clinical presentation. Excess liquid within the airspaces reduces compliance and increases intrapulmonary shunting, which results in tachypnea and mild to moderate hypoxemia. The chest radiograph may show opaque areas similar to neonatal pneumonia or surfactant-deficient respiratory distress syndrome (RDS). This picture is often described as “prolonged neonatal transition” or “delayed extrauterine adaptation.” Excess interstitial liquid reduces compliance and compresses small airways, which leads to the clinical signs of tachypnea and air trapping. The chest radiograph may show streaky densities within the lung, fluid collection within interlobar fissures, or even small pleural effusions; the lungs will also appear hyperinflated because of gas trapping. This clinical picture is often labeled retained fetal lung liquid or transient tachypnea of the newborn.5,17
Several additional points are worth noting. Infants who have been stressed in utero or whose mothers have been receiving β-mimetic agents are less likely to have retained lung liquid. Preterm infants with this condition may be mistakenly assumed to have surfactant-deficient RDS; supportive treatment is similar, although surfactant therapy has not been studied in infants with this condition. Because neonatal pneumonia also can present with tachypnea, an oxygen requirement, and a “wet” chest radiograph, infants with retained lung liquid who have symptoms often are treated with antibiotics. The clinical severity of this disorder can vary widely, and although most infants need only supplemental oxygen, others may require intubation and mechanical ventilation for 12 to 24 hours. Supplemental oxygen should be required for no more than 24 to 48 hours, but tachypnea may persist for several days.
It is of interest to note that infants born to mothers who have asthma are at increased risk of transient respiratory difficulty immediately after birth.18,19 In addition, reactive airway disease is more likely to develop later in life in newborns who present with transient respiratory distress.20,21
Pulmonary Air Leak Syndromes
Pulmonary air leak syndrome encompasses a spectrum of disease including pneumothorax, pneumomediastinum, pneumopericardium, subcutaneous emphysema, pneumoperitoneum, and pulmonary interstitial emphysema (PIE). As a group, pulmonary air leaks are more common during the neonatal period than at any other time of life. The two most common types of air leaks—pneumothorax and pneumomediastinum—occur spontaneously in 1% to 2% of term neonates.22 Preterm infants with surfactant-deficient respiratory distress had previously reported rates of air leaks in excess of 30%23; these rates fell rapidly with the advent of surfactant therapy in the 1980s but still remain around 5%.24 Infants with meconium aspiration or hypoplastic lungs have much higher rates of air leaks. Although techniques such as HFV and ECMO have markedly reduced the incidence of air leaks associated with these disorders, the rates still remain high, at 25% to 30%.25,26
The pathophysiologic cause of pulmonary air leaks has been hypothesized for many years as the uneven filling and redistribution of air in the lung; overdistension of more compliant air spaces leads to rupture. The alveoli most susceptible to this injury are those that border on arterioles and other structural elements of the lung; uniform protection by surrounding alveoli is lacking. After rupture of the alveolus or terminal airspace, air escapes into the lung interstitium and tracks along the vascular sheaths toward the hilum. For reasons that remain unclear, in the preterm infant the air may dissect within the interstitium (PIE).27 More commonly, the air breaks through the pleural reflections at the hilum (pneumomediastinum) and from there gains access to various potential compartments: the pleural cavity (pneumothorax), the pericardial reflection (pneumopericardium), and the soft tissue planes of the neck (subcutaneous emphysema), or across the diaphragmatic apertures and into the peritoneal space (pneumoperitoneum).
In the past, pulmonary air leaks most commonly occurred as a result of excessive ventilatory pressures due to either aggressive mechanical ventilation (barotrauma) or air trapping caused by partial airway obstruction with meconium or other debris (ball valving). Now, air leaks are more commonly seen during the recovery phase of acute respiratory disease, when lung compliance dramatically improves and pressure-limited ventilation leads to excessive tidal volumes (volutrauma). This phenomenon explains the clinical observations that air leaks tend to occur during the recovery phase of RDS28 and that the incidence of air leaks actually increased during early trials of surfactant therapy.29 Both observations underscore the need to closely monitor ventilatory volumes and wean pressure aggressively as compliance improves; these observations also suggest that volume-limited ventilation may be safer during the recovery phase of acute neonatal respiratory disease, although this theory has not been studied.
The approach to management of air leaks in infants is similar to that in older children. Pneumothorax, pneumopericardium, and occasionally pneumoperitoneum are acute, life-threatening emergencies because they can seriously impair cardiac output by decreasing venous return. A high degree of suspicion for air leaks in a patient who has a sudden cardiovascular deterioration for no apparent reason is critical for prompt diagnosis. Transillumination of the relatively translucent neonatal chest wall with an intensely focused light source is a quick and useful tool for diagnosing a large pneumothorax. Immediate aspiration of the air, preferentially with a large-bore angiocatheter, should be done without radiographic confirmation if the infant is severely compromised. Clinical signs of compromise, not the radiographic interpretation of percent size or extent, should dictate treatment, and many cases can be managed expectantly.30 An unstable or recurrent pneumothorax may require a thoracostomy tube; however, tube thoracostomy may have significant complications, including parenchymal lung injury, phrenic nerve paralysis, chylothorax, and hemorrhagic pericardial effusion.30 Penetration of the chest tube into the pulmonary parenchyma is a complication that often is not appreciated by chest radiographs; one study of autopsies of infants with pneumothorax reported a lung perforation rate of 25%.31 Cardiac tamponade resulting from a pneumopericardium may be suggested by distant heart tones and hypotensive shock with a normal-appearing electrocardiogram tracing (so-called electromechanical dissociation). Pneumomediastinum often is asymptomatic and rarely benefits from drainage, even in the presence of symptoms. PIE occurs predominantly in preterm infants and often leads to a vicious cycle of increasing ventilator delivery pressures to open alveoli compressed by extrinsic air, which in turn leads to more extravasation of air and further collapse. Conventional treatment of PIE, including positioning, selective mainstem intubation, and steroids, has been unsatisfactory.27,32 HFV, which may maintain alveolar patency while reducing inspiratory airway pressure, appears to be the ventilatory mode of choice in managing infants with PIE33 and refractory pneumothoraces.34
Pulmonary Hemorrhage
The term pulmonary hemorrhage is often misapplied in the neonatal intensive care setting. True pulmonary hemorrhage in a neonate is rare and almost alwaysresults in death. In most cases, what is called pulmonary hemorrhage is actually the most severe manifestation of pulmonary edema rather than vascular disruption.35 This distinction has been drawn by measuring the hematocrit of hemorrhagic fluid suctioned from the airway.36 The airway fluid generally will be 15 to 20 points lower than whole blood in the same patient at that time. Finding whole blood in the airway is rare and is usually a result of trauma from suctioning.
Similar to older children and adults, factors that alter Starling forces within the pulmonary microcirculation will predispose the lung to hemorrhagic pulmonary edema. These factors include increased perfusion pressure (e.g., left ventricular failure), increased blood flow (e.g., from a left-to-right shunt across a patent ductus arteriosus [PDA]), increased microvascular permeability (e.g., associated with sepsis or oxygen toxicity), and decreased oncotic pressure (e.g., protein malnutrition or water overload). Most infants who have pulmonary hemorrhage will have more than one risk factor present. In the neonate, the factors most commonly associated with hemorrhagic pulmonary edema are those that increase pulmonary blood flow, such as a left-to-right shunt or treatment with surfactants.35,37 Clinical features that increase the risk of hemorrhagic pulmonary edema or even frank pulmonary hemorrhage in neonates include extreme preterm gestation, asphyxia, hypothermia, certain types of congenital heart disease, and underlying coagulopathies.38 Pulmonary hemorrhage also has been associated with neurologic disorders, including seizures, stroke, subarachnoid hemorrhage, and massive intraventricular hemorrhage. This association has led some researchers to suggest that pulmonary hemorrhage may have a neurogenic origin and that neonates with known or suspected neurologic injury represent a particularly high risk group.39
Although the diagnosis of hemorrhagic pulmonary edema is relatively straightforward, management can be challenging. The most important approach to managing hemorrhagic pulmonary edema is to establish high positive end-expiratory pressure (PEEP); this not only effectively reduces alveolar flooding but also improves oxygenation and left ventricular function. Although the airway must be kept clear, frequent suctioning not only may be traumatic but also can aggravate the condition by reducing PEEP. High mean airway pressures, which can be safely achieved with HFV, can be effective in massive pulmonary hemorrhage with rapid improvement in oxygenation.40
Some persons have advocated administration of epinephrine or iced saline solution via the endotracheal tube, but the efficacy of this method is questionable, and epinephrine may worsen the condition by elevating pulmonary vascular pressures. Aggressive volume resuscitation also should be avoided for the same reason. Antibiotics should be considered if sepsis is clinically suspected, but the efficacy of prophylactic antibiotics to prevent bacterial contamination of the airways is unproven. If an underlying cause such as a PDA or coagulopathy is suspected, it should be treated accordingly. Additional therapies under investigation include surfactant41 and recombinant activated factor VII.42 Prevention also may be possible in some cases; in preterm infants at high risk of the development of hemodynamically significant PDA, indomethacin prophylaxis appears to reduce the incidence of severe hemorrhagic pulmonary edema.43 In a recent randomized trial of hemocoagulase given to preterm newborns requiring mechanical ventilation, the incidence of pulmonary hemorrhage (suspected or proven) was reduced by 70%44; however, in that study, the definition of “pulmonary hemorrhage” was rather broad and occurred in more than 40% of control infants, a much higher rate than is typically reported for this condition.
Even with aggressive management, the incidence of mortality from hemorrhagic pulmonary edema can exceed 25%, and surviving infants are at increased risk for neurodevelopmental impairment (e.g., cerebral palsy or cognitive delay) and neurosensory impairment (e.g., hearing loss requiring amplification or bilateral blindness).43
Pneumonia
The lungs represent the most commonly affected organ in neonates with sepsis. Bacterial or viral infection of the neonate may begin in utero, either by transplacental passage or, more commonly, ascending infection from the maternal genital tract. A delay from the time of rupture of the amniotic membranes until delivery increases the risk of an ascending infection, although some organisms may invade through intact membranes. Cervical bacterial colonization with group B streptococci or primary herpesviral cervical infection during pregnancy increases the risk of transmitting those diseases; however, routine cervical cultures taken during pregnancy often do not reliably predict the actual flora at the time of delivery. Also, infants born vaginally are invariably colonized with organisms from the vaginal canal and typically swallow organisms during vaginal passage. Cesarean delivery is not necessarily protective because fetuses may swallow contaminated amniotic fluid or aspirate organisms in utero. Infection occurring during the perinatal period may not present clinically for several days; thus infections congenitally acquired may be indistinguishable from infections postnatally acquired (i.e., nosocomial).
Organisms that cause perinatal pneumonias, then, typically are those found in the genital tract of the mother and include streptococci (groups A, B, and D), gram-negative rods (e.g., Escherichia coli and Klebsiella species), Listeria monocytogenes, Ureaplasma, genital hemophilus, and herpesvirus. Less commonly, maternal viral infections due to adenovirus, enteroviruses, or varicella can be vertically transmitted to the fetus. Although perinatal tuberculosis is rare compared with other causes of neonatal pneumonia, the increasing prevalence of this disease in women of child-bearing age increases the likelihood of new cases45; in congenitally acquired cases, the mother may be symptom free.46 Perinatal infection with other organisms, most typically chlamydia, may not present for several weeks.
Pneumonia as a nosocomial infection may develop in neonates, particularly those who require mechanical ventilation for other critical illness. Although reported rates vary widely, in part because of the lack of a diagnostic gold standard in this population, some authors have suggested that the incidence of ventilator-associated pneumonia may be as high as 30% in selected NICU populations.47,48 In addition to the presence of an endotracheal tube, risk factors for ventilator-associated pneumonias include low birth weight, prolonged mechanical ventilation, sedation with opiates, frequent endotracheal tube suctioning, and the crowding that is typical in some units.47,49 If a ventilator-associated pneumonia is suspected, typical nosocomial pathogens such as staphylococcus, Klebsiella and Pseudomonas species, and the pathogens previously listed for congenital pneumonias should be considered as possible causes.
Congenitally acquired pneumonia/sepsis can be a rapidly fatal disease, especially in the case of group B streptococcal or herpesviral infections, for which mortality rates as high as 50% have been reported.50,51 A high degree of suspicion is therefore important because prompt treatment may be lifesaving. Although antibiotics are routinely used in neonates with suspected pneumonia or sepsis, antiviral therapy should be considered if the infant has systemic signs such as shock or disseminated intravascular coagulation or is not responding to initial therapy.
In regard to group B streptococcus (GBS) infection, guidelines for antenatal screening and antepartum prophylaxis published by the Centers for Disease Control and Prevention in 1996, and revised in 2002, have been followed by a significant decrease in GBS-related neonatal morbidity and mortality in the United States.52 However, because of the inability of current microbiologic screening to identify all carriers, the failure in some cases to administer adequate intrapartum prophylaxis, and the need to deliver some infants prior to scheduled screening, GBS continues to be an important cause of early onset sepsis.53 Given the complications and potential limitations associated with maternal screening and intrapartum prophylaxis, vaccines may be the most effective means of preventing neonatal GBS disease and are currently under development.53
Meconium Aspiration Syndrome
Passage of meconium in utero is generally considered a sign of fetal distress. The stress can be acute, as in the case of cord compression during labor, or chronic, as in the case of preeclampsia. Moderate distress occurring during labor results in passage of meconium during the final stages of delivery (terminal meconium), whereas more severe or chronic distress results in passage in utero, with resultant staining of the amniotic fluid and fetus. The incidence of meconium staining as a more significant marker of fetal distress occurs in 10% to 20% of all deliveries and is most common in postmature infants.54 There also appears to be a maturational aspect to the ability to pass meconium because it is rarely observed in fetuses younger than 36 weeks’ gestation.54
Not only is meconium-stained amniotic fluid a sign of antenatal distress, but it also can cause subsequent difficulties in the neonate. The contaminated amniotic fluid may be aspirated by the fetus, either in utero or during passage through the birth canal, and lead to subsequent respiratory distress. Meconium is a lipid and protein-rich substance that is highly irritating to mucous membranes of the distal airways, resulting in a chemical pneumonitis.55 Dissolved meconium may travel down the respiratory tree and inactivate pulmonary surfactant; this inactivation leads to a functional surfactant deficiency.56,57 In addition, meconium induces a potent inflammatory response, further impairing lung function.58,59 As inflammatory markers improve, so too does pulmonary function.60 More particulate meconium will remain trapped in small airways, and this leads to a ball-valve type of gas trapping. In most cases the meconium is gradually removed from the respiratory tract through phagocytosis, and normal pulmonary function returns in 5 to 7 days. In more severe cases, meconium aspiration syndrome may lead to respiratory failure, and even death, despite aggressive intervention.
Infants with meconium aspiration are typically postmature, with elongated nails, peeling skin, and staining of the umbilical cord, skin, and nails. Respiratory distress develops soon after birth, although the infant’s respirations initially may be depressed if meconium passage occurred in response to a recent asphyxial episode in utero. Gas trapping may lead to a barrel-shaped appearance to the chest, and signs of respiratory distress may be severe. Chest radiographs often show characteristic patchy densities, hyperinflation, and areas of collapse. Air leaks are especially common. Aspiration of blood during delivery results in a similar clinical and radiographic picture; however, blood aspiration usually has a much milder course.61
An important step in the treatment of meconium aspiration syndrome is prevention. This has led to the routine practice of aggressive suctioning, beginning with clearing of the infant’s nose and mouth while still at the perineum and before delivery of the infant’s chest, followed by endotracheal intubation once the infant is delivered. A couple of large, randomized clinical trials have brought these practices into question and have even raised concerns that such interventions may produce harm, particularly in the vigorous infant.62,63 On the basis of these trials, current recommendations are that suctioning should be limited to infants who are not vigorous at delivery.64 Another intervention aimed at reducing disease as a result of meconium aspiration has been amnioinfusion, that is, the introduction of fluid transcervically during labor. The theoretical benefits of this approach include dilution of thick meconium and reducing cord compression by providing support to the umbilical cord. However, a recent large, randomized clinical trial has put this practice into question.65 As with tracheal suctioning, it can be argued that distressed fetuses may aspirate in utero, long before delivery, and that severe complications such as persistent pulmonary hypertension are more likely the result of the antecedent stress and are not due to meconium aspiration per se.
The treatment of meconium aspiration syndrome is to provide supportive care; infants are recognized as being at increased risk for having persistent pulmonary hypertension (see section on “Persistent Pulmonary Hypertension of the Neonate”). Supplemental oxygen support to maintain arterial oxygen saturation, endotracheal suctioning to clear remaining meconium, and ventilatory techniques to minimize gas trapping are techniques that are commonly used. HFV may be helpful in preventing subsequent air leaks. Antibiotics are commonly used because distinguishing the clinical and radiographic picture from sepsis may be difficult and because damage to the airways may predispose to subsequent bacterial infection. Surfactant therapy has been recently explored as an adjunctive therapy, following promising results from two small pilot studies.66,67 A recent systematic review of four clinical trials in term and near-term infants concluded that surfactant administration significantly reduced the need for extracorporeal life support, although the overall incidence of mortality was not affected68; in many centers, surfactant replacement has been added to other therapies routinely use in this condition, including high-frequency ventilation and inhaled nitric oxide.
A recent modification of surfactant replacement has been to lavage the lung with relatively large volumes of diluted surfactant to facilitate removal of meconium and improve surfactant function.69,70 While this approach has merit, more clinical work needs to be done to determine the optimal approach, because some infants do not tolerate the procedure.70
Despite the intense inflammatory nature of meconium aspiration, the utility of steroid administration remains unclear54; although a recent small, randomized, controlled trial showed some clinical improvements, the mortality rate remained high.71
Because meconium passage in utero and meconium aspiration syndrome per se often are associated with an hypoxic event, long-term outcome remains guarded, particularly with regard to neurodevelopment.72 In addition, a recent study has suggested that meconium aspiration during the perinatal period may be associated with increased risk of reactive airway disease in early childhood.73
Surfactant-Deficient Respiratory Distress Syndrome
Pulmonary surfactant disperses at the air-liquid interface on the inner surface of the alveolus, reduces surface tension at that interface, and prevents alveolar collapse at end-expiration. Surfactant is produced by type II alveolar epithelial cells and is a phospholipid and glycoprotein composite with phospholipid as the surface-active agent and glycoproteins aiding in surface adsorption, spreading, and metabolism of surfactant.74 Maturation of the pulmonary surfactant system is generally not complete until the latter part of the third trimester of fetal life, but it can be induced by intrauterine stress, by maternal steroid therapy, and after preterm delivery. The incidence of surfactant deficiency at birth is inversely related to gestational age; approximately 50% of infants born between 30 and 36 weeks’ gestation will be surfactant deficient, and virtually all infants born before 28 weeks’ gestation will be affected to some degree.75
Surfactant-deficient alveoli are more prone to collapse, and this leads to diffuse atelectasis, reduced ventilatory compliance, and intrapulmonary shunting. Infants with surfactant deficiency have stiff, noncompliant lungs and require significant distending pressure for the lungs to be ventilated. Neonates will have tachypnea, retractions, and expiratory grunting; these symptoms indicate RDS. Preterm infants with RDS have significant morbidity, although some complications may primarily be a result of prematurity per se. Short-term complications can be life-threatening and include pulmonary air leaks, pulmonary hemorrhage, and intracranial hemorrhage. Infants with RDS are at risk for later complications including necrotizing enterocolitis and chronic lung disease. Infants who require prolonged intubation and mechanical ventilation also are at risk for subglottic injury including subglottic stenosis and tracheomalacia. Before surfactant therapy, the mortality rate from RDS exceeded 20%76; now infants rarely succumb to RDS unless severe complications develop.77–79
Assessment of fetal lung maturity using phospholipid analysis of amniotic fluid and maternal steroid therapy, both introduced in the mid 1970s, have reduced the incidence of RDS in infants born before term. Treatment with surfactants in neonates with symptoms during the first few days of life has significantly reduced the clinical severity of RDS and improved survival.24
Unfortunately, although surfactant therapy has reduced some short-term complications such as pulmonary air leaks, the incidence of long-term morbidity remains unchanged, in part because of the increased survival rate of preterm infants with extremely low birth weight (<1000 g).78,79
Surfactant Protein B Deficiency
Pulmonary surfactant consists of surface-active phospholipid and a small amount (approximately 10% by weight) of protein.74 Various surfactant-associated proteins (SPs) have been identified. SP-A and SP-D are large molecular weight, hydrophilic glycoproteins. Although these larger proteins have no surface tension–lowering ability per se, SP-A regulates surfactant phospholipid synthesis, secretion, and recycling and blocks the inhibition of native surfactant by plasma proteins that may leak into the alveolus during lung injury. SP-D does not appear to be involved in surfactant function but does play a key role in host defense of the lung. Despite having little to do with surfactant function, a recent study found that certain SP haplotypes for SP-A and SP-D conferred protection against newborn RDS.80 In contrast to SP-A and SP-D, SP-B and SP-C are small, hydrophobic glycoproteins that promote spreading of surfactant across an air-liquid interface, which is an essential prerequisite for surfactant to function. A deficiency of either SP-B or SP-C markedly interferes with natural surfactant function in animal models.74
Congenital alveolar proteinosis is a rare disease entity with histopathologic similarities to alveolar proteinosis in older children and adults.81 The clinical course of congenital alveolar proteinosis, however, is markedly different, characterized by rapid progression to death within several hours to days. A clustering of cases within families has suggested a genetic basis for this disorder; the condition in older children and adults is thought to result from a nonspecific alveolar injury. Pulmonary lavage has been helpful in adults and some children with alveolar proteinosis, but it has not been studied in infants with congenital disease.82 Extracorporeal support has not altered the long-term prognosis of infants with this condition.83
In the early 1990s, several cases of congenital alveolar proteinosis were described in which SP-B and its messenger protein have been shown to be totally absent.84,85 Since then, more than 25 loss of function mutations have been identified in patients with SP-B deficiency, the most common being a single gene mutation (121 ins2).86 Adult humans heterozygous for this mutation have normal pulmonary function,87 whereas homozygous cases are uniformly lethal within days of life. Affected infants respond only transiently to exogenous surfactants that contain SP-B88; to date, the only survivors are those who have undergone a lung transplant. Molecular techniques can identify affected infants and even predict fetal outcome, allowing us to provide specific counseling for parents whose infants have this fatal disorder.89
Congenital Malformations of the Lung
Pulmonary Hypoplasia
Both static and dynamic expansion of the fetal lung appears to be an important determinant of normal fetal lung development.90 Static lung expansion occurs as a result of fetal lung liquid production. Epithelial cells within the lung actively secrete fluid into the lung lumen, distending the future airspaces. An intraluminal pressure gradient above amniotic fluid pressure is maintained by glottic regulation of fluid efflux from the trachea into the amniotic cavity, thus keeping the lungs expanded at a fluid volume that approximates postnatal functional residual capacity.91 Failure to maintain this distention, either by inadequate production or excessive drainage of fetal lung liquid, leads to developmental hypoplasia. Dynamic lung expansion occurs during fetal breathing movements, which are rhythmic in nature and occur with increasing frequency during the latter part of gestation.92 Absent or abnormal fetal breathing also appears to result in pulmonary hypoplasia.90
Pulmonary hypoplasia, unlike pulmonary agenesis or aplasia (discussed later in this chapter), can occur any time during gestation. Hypoplastic lungs are small in volume, and DNA content relative to body size have reduced numbers of alveoli, bronchioles, and arterioles per unit mass.90 Although the pathophysiologic origin is not well understood, pulmonary hypoplasia is thought to result from impairment of normal fetal lung expansion and generally occurs in conjunction with one of the following conditions: (1) space-occupying lesions within the hemithorax, such as a diaphragmatic hernia, or massive pleural effusions associated with fetal hydrops; (2) an inadequate thoracic cage, as in asphyxiating thoracic dystrophy or achondrogenesis; (3) a deficiency of amniotic fluid (oligohydramnios), either because of leakage (e.g., preterm rupture of fetal membranes) or underproduction (e.g., renal dysplasia) 25; (4) inadequate vascular supply to the developing lung, as may be seen with pulmonary artery atresia, hypoplastic right heart, or tetralogy of Fallot; (5) lack of the fetal breathing movements that normally occur throughout the latter part of gestation; and (6) chromosomal anomalies such as trisomy 13 or 18. Pulmonary hypoplasia may occur in the absence of any of these conditions, but such cases of primary isolated pulmonary hypoplasia are rare.90
Infants with pulmonary hypoplasia generally have signs of respiratory failure in the immediate newborn period. Reduced lung volumes impair ventilation and lead to hypercarbia, and decreased surface area for gas exchange (due to decreased alveoli) leads to hypoxemia. A decreased cross-sectional area of the vasculature makes these infants particularly susceptible to pulmonary hypertension, which further exacerbates the hypoxemia. The chest radiograph in infants with pulmonary hypoplasia should show low lung volumes but may otherwise be unremarkable. The severity of the respiratory distress depends on the degree of hypoplasia and the presence of associated problems such as fetal hydrops or cyanotic heart disease. The most common association is renal dysplasia or agenesis; in these cases infants have a history of moderate to severe oligohydramnios and have severe respiratory distress and compression deformities of the face and extremities (Potter’s syndrome).93
Congenital Diaphragmatic Hernia
Failure of the pleuroperitoneal canal to close at 6 to 8 weeks’ gestation results in a diaphragmatic defect that allows gastrointestinal structures to travel into the thoracic cavity as the intestines return from outside the fetus to the abdominal cavity.94 The resulting mass effect in the chest exerts a negative influence on ipsilateral lung growth, characterized by a quantitative reduction in airways and their associated preacinar arteries. Congenital diaphragmatic hernia (CDH) occurs in approximately 1 in 3000 births and is the most common cause of pulmonary hypoplasia in the neonate.95 The defect occurs on the left side in 80% to 85% of cases; the reason is that closure of the right pleuroperitoneal membrane normally precedes the left during fetal development. Because herniation often occurs before the tenth week of gestation when normal gut rotation occurs, malrotation is common. Nongastrointestinal anomalies are found in approximately 25% of cases; the most common involve the cardiovascular system, where virtually any kind of defect has been reported.94–96 Other associated anomalies include esophageal atresia, trisomies (13, 18, and 21), Turner’s syndrome, neural tube defects, and renal anomalies. While it has been assumed the pulmonary hypoplasia seen with CDH is secondary to mechanical forces, studies in newborns and animals have found that CDH may be associated with nutritional deficiencies.97,98 In the rat model of nitrofen-induced CDH, prenatal retinoic acid improves alveologenesis99; the experimental data regarding vitamin E supplementation has yielded conflicting results.100–102
Clinical presentation of CDH depends on the degree of pulmonary hypoplasia present (see section on “Pulmonary Hypoplasia”). In addition, the abdomen is often scaphoid because of a paucity of abdominal contents. As the infant cries and swallows air, the degree of lung compression may worsen, and an infant who appears healthy at delivery may undergo decompensation within minutes. The chest radiograph will show a cystic lesion in the lower lung field, often extending upward along the lateral chest wall. Initially, while the intestines remain fluid filled, the radiograph may be similar to that seen with pulmonary sequestrations or fluid-filled cysts (discussed later in this chapter); as the infant swallows more air, the radiographic findings can be confused with congenital emphysema or even a pneumothorax. Small or right-sided defects in infants may not present for weeks or even months; indeed, occasional cases have been diagnosed incidentally during childhood when chest radiographs are obtained for other reasons. Today, with the widespread use of antenatal sonography, most cases are diagnosed before birth, and significant confusion is avoided in the delivery room. In cases where sonographic findings are equivocal, prenatal magnetic resonance imaging may be particularly useful.103
Initial management focuses on stabilization, including immediate intubation and gastrointestinal decompression. Ventilation by bag and mask should be avoided because this intervention will only introduce more gas into the gastrointestinal tract. As with pulmonary hypoplasia, the clinical course is usually complicated by persistent pulmonary hypertension, which has accounted for mortality rates as high as 80%.104 With the advent of ECMO this number has dropped somewhat but is still significant.105 High-frequency ventilation appears to have particular merit for this condition and may improve mortality rates independent of bypass technology.96,106 In a lamb model of CDH the contralateral nonhypoplastic lung is functionally immature, leading some investigators to suggest that surfactant therapy may be beneficial,107 but this suggestion has not been borne out in a recent review of a large, national CDH registry.108 Attempts at intrauterine intervention, either to close the defect or to encourage lung growth through temporary blocking of the fetal lung liquid egress at the trachea, have been disappointing; fetal surgery is associated with an unacceptably high incidence of complications, including recurrence of the defect, preterm delivery, and miscarriage.96,109 In infants with significant pulmonary hypoplasia who require ECMO, distending the lung with perfluorochemical can promote lung growth, but further study is needed.110
Although early corrective surgery had been advocated in the past, recently a paradigm shift toward delayed repair has occurred, in large part because of the observation that respiratory function often worsens in the immediate postoperative period.95,96,111,112 Thus early, aggressive cardiorespiratory stabilization followed by surgery has become the recommended approach and is associated with improved outcome.95,113 An alternative to an initial period of attempted stabilization has been an ex-utero intrapartum therapy procedure, in which the delivering fetus is orally intubated and placed on a mechanical ventilator prior to umbilical cord ligation. In this approach, a brief trial of ventilation may be given; if oxygen saturation does not improve during the trial, the fetus is cannulated and ECMO is begun, followed by delivery of the infant. This approach may be helpful in selecting infants who are least likely to respond to initial stabilization in the NICU, and reported outcomes with this approach have been favorable, although the mortality rate for infants with this condition remains quite high.114
Cystic Adenomatoid Malformation
Cystic adenomatoid malformation (CAM) is a relatively infrequent lesion, estimated at 1 in 30,000 pregnancies.115 It results from abnormal mesenchymal proliferation and failure of maturation of bronchiolar structures early in gestation.116,117 The resultant adenomatous overgrowth leads to the development of cysts and suppression of alveolar growth. The cysts are almost always multiple, and in more than 95% of cases the cystic malformations lie within a single lobe. No lobar predilection exists. Histologically, the lesions are notable for the preponderance of elastic tissue and for a lack of cartilage. The cysts communicate directly with the tracheobronchial tree and with each other.
CAMs have been divided into three types, which vary both in anatomic and clinical characteristics. According to the traditional Stocker classification scheme, Type 1 CAM, which accounts for about half the cases, occurs as a few large (>2 cm) cysts, usually one to four in number, or a single large cyst surrounded by much smaller “satellite” cysts. Type 2 CAM, which accounts for about 40% to 45% of the cases, consists of multiple, small (<2 cm), evenly spaced cysts scattered throughout the affected area. Compared with type 1 CAM, type 2 cysts are associated with a much higher incidence (about 25%) of anomalies in other organs, particularly within the genitourinary tract (e.g., renal dysgenesis). Type 3 CAM, which accounts for less than 10% of cases, occurs as large collections of numerous tiny cysts; the affected area can be large, and this type often leads to early cardiovascular compromise, resulting in fetal hydrops or immediate postnatal complications. The Stocker classification scheme is based primarily on postnatal lung examinations; recent studies of fetal lung specimens suggests that a different classification scheme may be needed, particularly as this condition is now detected antenatally in most cases.118,119
Treatment of infants with symptoms may require positive pressure ventilation; in some infants PEEP may facilitate emptying of the cysts. Definitive treatment is surgical removal of the affected lobe, which can be done thoracoscopically in many cases.120 Even if an infant is asymptomatic, surgical resection is recommended by 3 to 6 months of age because of the high risk of expansion or infection if the cysts are left untreated.115,121
Prognosis depends on the type and extent of the CAM. The large type 3 lesions are more likely to cause immediate distress and carry a higher incidence of mortality, especially if associated with pulmonary hypoplasia or fetal hydrops.115,121 The prognosis in type 2 CAM depends on the presence and nature of associated anomalies. In addition, malignant transformation of CAM has been reported.94,116,121 Most cases of CAM, however, carry a good prognosis.115,117,121
Bronchogenic Cysts
Bronchogenic cysts occur as a result of anomalous budding of the ventral or tracheal diverticulum of the foregut during the sixth week of gestation, with subsequent separation from the normally developing bronchi by the sixteenth week of gestation.116,117 If separation occurs early (<12 weeks), the bronchogenic cyst tends to be located in the mediastinum (which is the most common type); if separation occurs later, it is more likely to occur in the peripheral pulmonary parenchyma. The cyst walls are cartilaginous and receive either systemic or pulmonary blood supply depending on their location. Bronchogenic cysts are more common in male infants, are usually singular, are more commonly right sided, and are generally less than 10 cm in diameter. They generally do not communicate with the airway and remain fluid filled, which differentiates them from pulmonary parenchymal cysts.
Chest radiographs can readily disclose most bronchogenic cysts.122 The cyst typically appears as a round or oval water-density mass, commonly in the mediastinal or perihilar area; if the cyst has been infected, an air-fluid level may be present. Mediastinal bronchogenic cysts usually appear just beneath the carina and extend to the right. Pulmonary bronchogenic cysts are usually sharply circumscribed and appear toward the periphery; two thirds of these cysts will be located in the lower lobes, with no right or left predilection. About 25% of bronchogenic cysts may be difficult to visualize with a chest radiograph; in these cases CT scans cannot only delineate the lesion but can also discern associated anomalies, such as a pulmonary sequestration (see later).116,122
Treatment may require ventilatory support for infants with symptoms. In all cases, surgical resection is indicated. Prognosis for infants with bronchogenic cysts, whether mediastinal or pulmonary, is good.123
Pulmonary Parenchymal Cysts
Pulmonary parenchymal cysts are thought to represent a disorder of bronchial growth,116,117 although they also may be acquired.124 Like adenomatoid malformations and bronchogenic cysts, congenital cysts arise early in fetal life; pulmonary parenchymal cysts are thought to develop at a time when completion of the terminal bronchioles and development of the alveoli are occurring. Pulmonary cysts are typically thin walled, singular, multilocular, and located in the periphery. Unlike bronchogenic cysts, some communication usually exists between the pulmonary cyst and the tracheobronchial tree, and thus approximately 75% will become filled with air. Like adenomatoid malformations, pulmonary cysts contain mostly elastic tissue and little or no cartilage.
Although pulmonary cysts are generally small (1 to 2 cm in diameter), they can expand dramatically and thus are much more likely to be symptomatic than are bronchogenic cysts. As with adenomatoid malformations, the lack of cartilaginous support leads to trapping of air. Unlike adenomatoid malformations, pulmonary cysts are rarely associated with other anomalies. Rupture of a peripheral cyst can result in a pneumothorax. Rarely, multiple cysts can occur and involve both lungs in an extensive fashion; these cases are generally fatal within the perinatal period.
Chest radiographs typically reveal thin-walled, round cysts with an air density. Often faint strands of lung tissue can be seen within the cysts. A large pulmonary cyst may be confused with congenital lobar emphysema; in this case a CT scan should easily distinguish the cystic nature of the former condition.122
Reports of spontaneous resolution of pulmonary cysts have been infrequent. As with other cystic lesions of the lung, however, surgical resection of the affected lobe is usually indicated.116,124
Pulmonary Sequestrations
Like bronchogenic cysts, pulmonary sequestrations are thought to result from an abnormal budding of the foregut, which retains its embryonic systemic arterial connections.116,117 Thus a sequestration is a mass of nonfunctioning, ectopic pulmonary tissue with its own blood supply. Pulmonary sequestrations are divided into two types, which are histologically similar. Extralobar sequestrations are surrounded by their own separate pleura, and intralobar sequestrations have no separate pleural covering.
Intralobar sequestrations are the more common type (75%); they are usually left sided (65% to 70%) and typically occur in the lower lobes. Unlike extralobar sequestrations, they are rarely associated with other anomalies.125 Most cases are asymptomatic and are found on chest radiographs obtained for other reasons. Symptomatic cases typically present in late childhood with recurrent infections.
Distinguishing between extralobar and intralobar sequestrations on chest radiographs may be difficult. Both can appear as either solid or cystic structures, although extralobar lesions are more often solid and intralobar lesions are more often cystic.122 Delineation of the vascular supply to the sequestration is important, not only to differentiate extralobar sequestrations from adenomatoid malformations, but to guide surgical management; in the past few years, magnetic resonance imaging has replaced arteriography for obtaining this information.122 Some authors recommend a study of the gastrointestinal tract, particularly if communication with the sequestration is suspected.
As with other cystic lesions of the lung, surgical removal is indicated and may be done thoracoscopically.126–128 Whereas extralobar sequestrations can be removed en bloc because of their separate pleural covering, intralobar sequestrations require lobectomy.94 Recently, success with a nonsurgical approach using transumbilical artery embolization during the newborn period has been reported.129
Congenital Lobar Emphysema
Congenital lobar emphysema is an unusual disorder characterized by overdistension of a pulmonary lobe caused by air trapping. The term emphysema is misapplied because usually no emphysematous destruction of the alveoli occurs; thus many authors favor the term congenital lobar overinflation.94,116,117 Although clinical and radiographic findings are typical of a ball-valve type of obstruction, evidence for this is found in less than 25% of cases. In most cases the cause for the air-trapping is unknown. Intrinsic bronchial obstruction may be the result of a deficiency of cartilaginous support or an intraluminal mass such as a mucous plug. Extrinsic bronchial obstruction usually results from an underlying cardiovascular abnormality, such as a vascular sling or, rarely, a PDA. Intrathoracic masses, such as an enlarged lymph node or a bronchogenic cyst, can lead to extrinsic obstruction as well.
Congenital lobar emphysema is more common in male infants and typically occurs in the upper lobes or the right middle lobe; fewer than 1% of cases occur in the lower lobes. Up to 20% of the cases have bilateral involvement. Associated cardiovascular anomalies are common; rib cage anomalies and aplasia/dysplasia of the kidneys have been reported in a small percentage of cases. In most infants with congenital lobar emphysema, the condition presents within the first month of life, and about one third of infants have symptoms within hours of birth. Symptoms relate directly to the degree of overinflation. Typically, infants have mild to moderate tachypnea, asymmetric inflation of the chest, and cyanosis. A chest radiograph reveals the overinflated lobe with ipsilateral atelectasis and flattening of the hemidiaphragm; also, a mediastinal shift away from the affected side may be observed. A CT scan may be helpful in identifying the cause of obstruction, if one is present. Lobectomy is the definitive treatment and may be done thoracoscopically.130 Surgery should be limited to symptomatic cases; favorable outcomes reported in asymptomatic or minimally symptomatic cases in which surgery was not conducted suggests that a less aggressive approach may be warranted.131
Pulmonary Agenesis and Aplasia
Pulmonary agenesis and aplasia, which are both rare and highly lethal disorders, have similar underlying causes that differ from those of pulmonary hypoplasia.117 Pulmonary agenesis and aplasia result from an arrest of development of the primitive lung during embryonic life. Obviously, the earlier in development that the arrest occurs, the more severe the defect. In pulmonary agenesis, the bronchial tree, pulmonary parenchyma, or pulmonary vasculature does not develop. In pulmonary aplasia there is a rudimentary bronchial pouch. The resulting lesion may involve one lobe or the entire lung; focal or bilateral defects are rare. Pulmonary agenesis or aplasia may be associated with other nonpulmonary anomalies including microphthalmia/anophthalmia, cleft palate, cardiac defects, congenital diaphragmatic hernia/eventration, and limb abnormalities.132
The clinical presentation is variable. If the defect is focal and isolated, the infant may not have any symptoms, but usually some mild respiratory distress is present. A chest radiograph reveals unilateral lung or lobar collapse with a shift of mediastinal structures, which leads to a suspicion of bronchial or bronchiolar obstruction. Misdiagnosis may subject the infant to the unnecessary risks of bronchoscopy when CT is readily diagnostic.133 Associated anomalies in the cardiovascular, gastrointestinal, genitourinary, central nervous, and musculoskeletal systems have all been described.117
If the defect is isolated to a single lobe, surgical resection will reduce symptoms and lessen the chance for infection.134 If the defect is extensive but the fetus is considered salvageable, an ex-utero intrapartum therapy procedure may be performed.
Prognosis depends on the degree of pulmonary involvement, a history of recurrent pulmonary infections, and the presence of associated anomalies.117 Bilateral defects are invariably lethal. If the defect is focal, the remaining normal lung tends to hypertrophy to compensate. Still, mortality rates exceed 50%, generally because of the presence of associated malformations, which are common. Right-sided defects have a poorer prognosis than left-sided lesions, partly because of a higher association with other anomalies and partly because of an increased risk for disseminating infection. It also has been suggested that right-sided lesions produce a greater mediastinal shift, distorting the trachea and great vessels.135,136 Repeated lower respiratory infections result in progressive pulmonary debilitation and also increase the risk for death.
Special Treatment Considerations for Acute Respiratory Failure
Surfactant Replacement
Exogenous surfactant therapy has significantly decreased the incidence, severity, and morbidity of RDS and improved the survival of low-birth-weight infants.137 Exogenous surfactants in current clinical use are considered “natural” because they are derived from either bovine or porcine lungs. Because of concerns for animal protein sensitization and for ease of standardization, newer surfactants under development and not yet commercially available contain no animal proteins but consist of surface active phospholipids and recombinant proteins that exhibit properties similar to the natural surfactant proteins, either B or C. While these surfactants may prove to have advantages over surfactants derived from animals, the earlier concerns regarding sensitization to foreign animal proteins have not been substantiated in more than two decades of clinical use. Human surfactant, purified and concentrated from pooled amniotic fluid, also has been studied clinically and is effective, but it is too expensive and sparse in supply to allow general use.
The three animal-derived surfactants are compositionally different from each other, even though two of them come from the same animal source. These differences are in large part due to the way in which the surface-active components are either extracted, or supplemented, in the different preparations. While most compositional differences are of little biophysical or clinical importance, differences in SP-B content appear to translate into clinical differences such as onset and duration of activity and resistance to inhibition.138 This difference is important because many other clinical disorders besides RDS can be associated with “functional” surfactant deficiency. Increasing evidence exists that conditions such as meconium aspiration, persistent pulmonary hypertension, hemorrhagic pulmonary edema, congenital diaphragmatic hernia, pulmonary hypoplasia, acute respiratory distress syndrome (ARDS), pneumonia, bronchiolitis, and asthma are associated with surfactant dysfunction and inactivation and that persons with these conditions might theoretically benefit from exogenous surfactant therapy 67,139–145 Treatment of these conditions may prove to be an appropriate niche for synthetic products that contain protein analogues.146
High-Frequency Ventilation
Two types of HFV are commonly used in neonates.34,147–150 The first type uses a highly pressurized intermittent jet of gas that is delivered at rates of 120 to 600 Hz and is called high-frequency jet ventilation. The second type uses an oscillating diaphragm or piston to provide active instillation and withdrawal of gas and is called high-frequency oscillatory ventilation (HFOV).
Although the effects of ventilator settings have been well studied in HFV, the actual mechanics of gas exchange during HFV are less well understood. Similar to conventional ventilation, oxygenation is affected primarily by inspired oxygen concentration and mean airway pressure. Although ventilation is affected by volume and frequency, the effect of volume is much more pronounced than with conventional ventilation, and the effect of frequency is contrary to what would be expected because it inversely affects tidal volume.34,147,150 In general practice, frequency is not a critical variable within the ranges afforded by the high-frequency ventilator and is not further adjusted during HFV use.
One key advantage to HFV of particular use in the neonate is the ability for high-end expiratory pressures to be used without the need for high-inspiratory pressures to maintain normal tidal volumes, allowing adjustment of mean airway pressure more or less independently of volume. This ability is particularly desirable when a significant V/Q mismatch exists, such as with the neonate who has idiopathic persistent pulmonary hypertension and whose lungs may be easy to ventilate but who is marginally oxygenated with 100% oxygen. Another advantage of HFV is the ability to use low, usually subphysio logic tidal volumes. The use of low volumes prevents overdistension and rupture of more compliant alveoli, thereby reducing the risk of pneumothorax. Furthermore, HFV reduces airflow across existing air leaks, not only promoting their closure but also enabling more effective ventilation in situations such as severe PIE or a bronchopleural fistula.34,147,148 Still, a specific advantage of HFV over conventional pressure ventilation in preterm infants with RDS, other than managing air leaks, has yet to be demonstrated.34,147,148 Rehospitalized NICU graduates with chronic lung disease frequently require mechanical ventilation following upper airway infection, particularly with organisms such as respiratory syncytial virus (RSV). The use of HFV may attenuate additional lung injury by allowing higher PEEP while minimizing volutrauma in these babies; however, this advantage is theoretical and not well studied to date.
Complications that have been reported with HFV use include hypotension, pulmonary hypertension, tracheobronchitis, and in preterm infants, intraventricular hemorrhage.34,147–150 Because the use of excessive airway pressures may impede cardiac return and increase pulmonary vascular resistance, hyperinflation must be avoided. Reports of necrotizing, sometimes lethal tracheobronchitis, a complication recognized during the early years of HFV use, are now rare, presumably because of better attention to humidification and avoidance of excessive mean airway pressures. While some studies have suggested that infants of very low birth weight (less than 1500 g) treated early with HFOV have a lower incidence of chronic lung disease,151 others have suggested that HFOV increases the risk of intracranial hemorrhage in preterm infants, perhaps because of alterations in cerebral blood flow and drainage.152–154 Researchers in several other clinical studies have found no such association,106,151,155–160 and researchers in two studies in animals found no differences in the effects of HFV and conventional ventilation on the cerebral circulation.161,162
Extracorporeal Membrane Oxygenation
ECMO evolved from classic bypass technology with the advent of membrane oxygenators that could operate for days without significantly disrupting blood cells and plasma proteins. However, when applied to premature neonates with RDS, ECMO initially was disappointing.163 ECMO did not reduce mortality rates in patients with ARDS and was associated with a high risk of intracranial hemorrhage in preterm infants. Nevertheless, subsequent use in term infants with cardiorespiratory failure, particularly those with persistent pulmonary hypertension and congenital diaphragmatic hernia for whom conventional therapies had failed, has led to its increasing application in this area, despite the absence of controlled clinical trials.164 In neonates with respiratory failure, compared with other indications for ECMO, RSV/lower airway disease has a far higher survival rate (96%) and very low rate of neurologic sequelae.165,166 Most causes of neonatal respiratory failure in the newborn are self-limited, and ECMO allows time for the lung to recover from the underlying disease process and for reversal of pulmonary hypertension, which frequently accompanies respiratory failure in the newborn.167 In addition, the use of ECMO in older children and adults also has received a resurgence of interest see Chapter 53. The technical description, aspects of clinical management, and potential complications of ECMO are covered elsewhere in this book and are not detailed here.
Nitric Oxide Inhalation
While nitric oxide inhalation has received much interest in the management of term infants with persistent pulmonary hypertension, other applications continue to be evaluated. Animal studies suggest that nitric oxide may have a significant role in the successful perinatal transition to breathing of air.168,169 Clinical studies suggest that inhaled nitric oxide may be beneficial in infants with surfactant-deficient RDS, particularly those who do not respond to exogenous surfactant therapy.170,171 The basis for these studies lies in the observation that pulmonary hypertension is not confined to term neonates but has been reported in preterm infants with RDS as well.172,173 In addition, recent work has shown that nitric oxide production modulates basal pulmonary vascular tone in preterm animals.174
Many questions regarding nitric oxide use remain to be answered before it can be approved for clinical use in preterm infants with RDS. Recognized clinical complications include methemoglobinemia (because of its high affinity for hemoglobin) and prolonged bleeding times175;the toxicity of nitric oxide metabolites includes the potential for injury to the pulmonary epithelium and surfactant system as well.176 In time, the intense amount of ongoing basic research in nitric oxide biochemistry and clinical trials in both adults and children will begin to answer important questions about dosing, safety, and efficacy.177,178
Liquid Ventilation
Liquid ventilation with perfluorochemicals, a complete departure from traditional ventilation with gases, is one of the latest advances in ventilatory management for the neonate. Specific advantages of liquid instead of gas expansion of the lungs were first demonstrated in the 1920s, but application to the clinical setting was hampered by the poor solubility of most liquids for oxygen and carbon dioxide and technical difficulties in achieving liquid tidal volume exchange. The introduction of perfluorochemicals, which have high solubility for respiratory gases, and the development of specific liquid ventilators enabled researchers to study liquid ventilation in animals in the 1970s.179 The demonstration that complete tidal liquid movement was not necessary to capitalize on the advantages of liquid ventilation180 made liquid ventilation a clinical reality in the 1990s. Partial liquid ventilation is the term applied to conventional gas tidal volume ventilation superimposed on liquid-filled alveoli (i.e., alveoli filled to functional residual capacity).
Though gas exchange can be impaired by too rapid liquid ventilation in the healthy lung in animal studies,181,182 it is clearly improved in many injured lung models. Possible mechanisms for this improvement includes reducing surface tension and maintaining alveolar stability, thus eliminating the need for alveolar surfactant by filling the alveoi with liquid and thereby removing the air-liquid interface normally present. Perfluorochemicals also may act as a mechanical PEEP, holding the alveoli open because of the higher density of the liquid. Therefore partial liquid ventilation offers the potential to manage infants with respiratory distress resulting from surfactant deficiency or dysfunction, in which exogenous surfactant replacement has failed or becomes impractical (e.g., heterogeneous lung disease such as ARDS). In animal models, perfluorochemicals also reduce neutrophil accumulation and inflammatory responses to lung injury183–186 and inhibit hydrogen peroxide and free radical production by macrophages.187 Studies in animals also show that partial liquid ventilation leads to marked improvements in respiratory mechanics compared with conventional ventilation strategies.188–192 In 13 premature infants with severe RDS for whom conventional mechanical ventilation had failed and who had a high predicted risk of mortality, 10 of 13 received partial liquid ventilation for 24 to 72 hours,193,194 resulting in dramatically improved oxygenation and compliance; 8 of 10 survived to 36 weeks gestational age without significant complications. Large randomized human trials have been limited by the dose-dependent risk of hypoxia and barotrauma associated with perfluorocarbon.195 No studies of perfluorochemical liquid ventilation have been conducted in term neonates with respiratory disease; however, other limited clinical trials and individual case reports have been encouraging.194,196–200
Chronic Pulmonary Disease
Chronic Lung Disease (Bronchopulmonary Dysplasia)
The first description of a chronic respiratory disease in neonates was in 1967 by Northway et al.,201 who reported long-term radiographic and clinical outcomes of 32 low-birth-weight infants with acute respiratory disease. The infants described by Northway and colleagues progressed from acute respiratory disease (typically, hyaline membrane disease) requiring mechanical ventilation to a chronic phase with persistent oxygen requirement and respiratory distress, often resulting in right ventricular failure and death. Northway et al. coined the term bronchopulmonary dysplasia (BPD) to describe the patho logic findings in these infants at autopsy.
Over the years, this term has been loosely applied to include a wider variety of infants with chronic lung disease, most of whom survive.202,203 Because of improvements in the management of severe RDS, pulmonary hypertension, and pulmonary hypoplasia and because of increased survival rates of infants born between 23 and 26 weeks’ gestation, the BPD characterized in the original description by Northway and colleagues is relatively rare. The pathophysiology of BPD has evolved to encompass a new population of infants with chronic lung disease: extremely-low-birth-weight infants (weighing less than 1000 g) who without today’s advancements in medicine and technology could not have survived at the time of the investigations by Northway et al. These infants typically have a relatively mild course of acute respiratory disease and usually wean to minimal ventilatory support, oxygen support, or both within the first 72 hours of life. The oxygen requirement, however, tends to persist for weeks, months, or even years, and these infants are at extreme risk for respiratory exacerbations. Although the incidence of mortality is rare, those with this “new” type of BPD who die typically do not show severe lung injury with marked fibrosis and cellular proliferation, as seen in infants with classic BPD. Instead, one finds arrested lung development, with evidence of both impaired vascular and alveolar growth.204–207
The pathophysiologic cause of chronic lung disease after relatively mild acute respiratory disease in these extremely-low-birth-weight infants may, in part, relate to an increased sensitivity and abnormal responsiveness of the immature airways to injury.205,206,208–219 It has been suggested that preterm birth during the late canalicular stage (24 to 27 weeks’ gestation) leads to an arrest of normal lung development213; this suggestion is consistent with the observation that “normal” preterm infants (i.e., those without clinical evidence of lung disease) have dysfunction of terminal respiratory units and higher elastic recoil than do term infants at comparable postmenstrual ages.212 This arrested development sets the stage for disordered repair when the immature lung is subjected to postnatal injury, perhaps by oxidants, infection, or ventilatory trauma.209 Inflammation clearly plays a role in the development of BPD210; indeed, evidence exists that it may begin in utero.213 Mediators of epithelial lung injury include a variety of cytokines, including interleukin-1β (IL-1β), IL-6, and IL-8218; in addition, impaired signaling of growth factors such as vascular endothelial growth factor has been implicated.208,215 Given these considerations, it is likely that the pathogenesis of BPD, particularly in the extremely-low-birth-weight infant, is multifactorial in nature.
Generally, the incidence of BPD increases with decreasing gestational age: at less than 28 weeks’ gestation the incidence is approximately 40%, and it approaches 90% for infants at less than 26 weeks’ gestation.202,203 Additional risk factors include maternal infection, neonatal sepsis, and PDA.219 Although better monitoring, improved ventilation strategies, and new technologies have all had a positive impact on reducing the incidence and severity of BPD in the more mature neonate, increased survival of extremely-low-birth-weight infants has increased the overall prevalence of this condition in NICU graduates.
Treatment of infants with BPD is generally supportive. The goal of treatment is to promote lung growth while simultaneously supporting respiratory needs and minimizing further injury to the lungs. Supplemental oxygen should be used for infants with oxygen saturations below 93% in room air. Bronchodilators may be helpful particularly during exacerbations because often there is a large airway component to BPD. Fluid restriction or diuretics may be helpful in infants with evidence of cor pulmonale or fluid intolerance. At initial presentation infants often receive a few days of antibiotics until infection is ruled out. Infants with pulmonary insufficiency have increased energy needs,211 making nutritional support particularly important, although it is often overlooked.220
An approach aimed at reducing the inflammation and ongoing fibrotic injury is the use of steroids. However, although earlier study results have shown that intravenous dexamethasone reduces the severity of BPD and improves respiratory outcome in older premature infants,221,222 concerns have been raised regarding long-term safety, particularly from studies that included younger and more immature infants.223–225 Because of these observations and a growing body of data that questions the safety of high-dose steroids in extremely premature animals, the Academy of Pediatrics Committee on the Fetus and Newborn has recommended that steroid use for infants with BPD be within the context of well-designed, randomized trials until issues of long-term efficacy and safety are more clear.226 Given the rapid and sustained response that many infants with BPD have to steroids, significant controversy exists regarding this recommendation, particularly as it applies to older or less premature infants with significant pulmonary disability.
What is clear is that infants who receive long courses of steroids for BPD usually show chemical evidence of adrenal suppression, which may last for several months;221 infants who receive steroids for more than several days also should receive supplementation at times of increased stress (e.g., surgery and infection). It also is apparent that the inhaled method of administering steroids offers no particular advantage over the intravenous route, except for ease of administration.227 Potential new therapies to prevent or reduce the severity of BPD include synchronous mode ventilation, HFV, antioxidants, nitric oxide, antiproteases, vitamin A, and antenatal therapy with steroids and thyrotropin-releasing hormone; however, to date, study results have been inconclusive.151,155,157,217,228–231
The long-term outcome for infants with classic BPD has improved over the years, although these infants remain at risk for significant morbidity and mortality.219,232 Infants typically require supplemental oxygen for the first several months of life, and some may require chronic diuretic and bronchodilator use. High-caloric formulas should be considered for infants who cannot tolerate fluid loading. Rehospitalizations as a result of respiratory exacerbations, which often are brought on by infection, are common during the first 2 years of life.233 Persons with relatively uncomplicated courses will gradually improve and by school age often have normal lung function.219
The long-term outcome for extremely-low-birth-weight infants with new BPD is less clear. Although these infants often require little or no respiratory support at the time of discharge from the NICU, fewer data exist on long-term outcome because extremely-low-birth-weight infants rarely survived to discharge from the hospital before the advent of surfactant replacement therapy in the 1980s. If these infants indeed have an arrest of lung epithelial and vascular development as a result of preterm birth, long-term cardiorespiratory function remains unclear. As with infants with classic BPD, these infants are at increased risk for rehospitalization as a result of pulmonary infection and reactive airway disease,234 but they also may be at increased risk for long-term pulmonary dysfunction.235–237
Mortality as a result of BPD has markedly improved during the past decade but remains high in infants with complicating conditions (such as cardiovascular disease) or in those with intercurrent respiratory infections, particularly RSV or adenovirus. Passive immunization against RSV is now possible and is recommended for high-risk premature neonates238; however, it is costly and is not uniformly protective.239,240 Minimizing exposure to environmental hazards, whether infectious or irritant (e.g., from kerosene burners or cigarette smoke), cannot be overemphasized.230
Congenital Defects of the Lymphatics
Chylothorax is not an uncommon entity encountered in the pediatric ICU because it can be a complication of cardiothoracic surgery; however, postoperative chylothorax has a different natural history and prognosis than does congenital chylothorax, which is thought to be due to a failure of peripheral and central lymphatic channels to fuse, or perhaps a rupture of inadequately fused channels at birth.241 Most affected infants have symptoms within hours of birth and require mechanical ventilation. Congenital chylothorax, in contrast to the postoperative variety, can be associated with chromosomal abnormalities and other malformations, as described by a recent case series of 11 newborns. Familial cases are especially common in babies with associated congenital pulmonary lymphangiectasis with bilateral chylothoraces.242 Progressive respiratory compromise develops as fluid accumulates in the hemithorax. Drainage is both diagnostic and therapeutic; initially the lymphocyte-rich fluid is clear, but it becomes opaque when milk feedings are introduced. Nutritional support is critical because of the tremendous loss of protein in the chylous drainage. Most cases self-resolve in 2 to 3 weeks; occasionally, a several-day course of a somatostatin-analog, which reduces chyle flow, or an attempt at surgical closure of the thoracic duct is indicated. Whether congenital or postoperative, common complications include nosocomial infection, hemodynamic disturbance, and protein loss.243 In the congenital variety, length of time to resolution is significantly affected by additional underlying problems.242
Pulmonary lymphangiectasia is a very rare condition that can be a primary condition or a secondary dilation of pulmonary lymphatics resulting from obstructed pulmonary venous flow.241,244 A primary lymphangiectasia can be isolated, which is termed congenital pulmonary lymphangiectasia, or it can be part of a generalized condition that includes intestinal lymphangiectasia, in which pulmonary involvement is less severe. Congenital pulmonary lymphangiectasia is thought to result from failure of connective tissue elements to normally regress during fetal lung development. In some cases, a hereditary pattern has been suggested. Affected infants usually have symptoms soon after birth; however, some infants may remain symptom free for several weeks. Affected infants are usually born at term and may appear normal except for mild tachypnea, or they may be more severely affected with cyanosis or be frankly hydropic245; infants born preterm may be mistaken as having surfactant-deficient RDS. Radiographs generally reveal streaky reticular densities as a result of engorged lymphatics, and occasionally a finer, “ground-glass” appearance may be confused with surfactant deficiency. Pleural effusions have been reported but are unusual. The condition is progressive and untreatable; most infants die within days, but an occasional survivor beyond infancy has been reported.
Nonpulmonary Conditions that Result in Respiratory Disease
Many nonpulmonary disorders may present with respiratory distress in the neonate (Box 46-1). Conditions that affect the control or mechanics of breathing, the patency or integrity of the upper airway, perfusion to and from the lung, or acid-base balance can present with increased respiratory effort or signs of respiratory insufficiency (i.e., respiratory acidosis or hypoxemia). The clinical and radiographic picture may be consistent with an underlying pulmonary pathologic condition, but nonpulmonary causes must be considered, particularly if an infant is gravely ill or not responding to conventional treatments. In many of these nonpulmonary conditions, a delay in diagnosis can lead to irreversible injury and death.
Box 46–1 Nonpulmonary Conditions that Cause Respiratory Distress in the Newborn
Apnea of Prematurity
Although apneic spells are a common cause of rehospitalization following NICU discharge, apnea of prematurity is a diagnosis of exclusion. Most infants with apnea of prematurity achieve complete resolution of symptoms by 34 to 36 weeks after conceptual age. Once apnea of prematurity has resolved, it does not come back,246 and pathologic causes such as hypoglycemia, hypoxemia, hypothermia or hyperthermia, infection, left-to-right shunt, and intracranial hemorrhage should be ruled out. Of particular concern is new-onset apnea in a previously symptom-free infant. Apnea also increases in response to less stressful stimuli, such as immunizations or an ophthalmoscopic examination. Apnea in a term or near-term infant is almost always pathologic.
Isolated apnea may be treated with simple tactile stimulation in many cases. Infants who remain apneic after stimulation may require blow-by oxygen, nasal cannula therapy, nasal continuous positive airway pressure, nasal intermittent mechanical ventilation, or even re-intubation and mechanical ventilation depending on the severity, recurrence, and presence of underlying pathology. Caffeine is the pharmacologic agent of choice to treat apnea because of a long plasma half-life and low toxicity. It is interesting to note that whereas high neonatal serum levels of magnesium resulting from maternal magnesium therapy have been associated with apnea, magnesium deficiency can also cause apnea. In one retrospective study of premature infants receiving supplemental magnesium because of low serum levels, reduction in apneic episodes was noted247; however, because of a narrow therapeutic index, using magnesium to treat preterm neonatal apnea would require frequent serum levels that are not necessary when using caffeine.
Choanal Atresia/Stenosis
Choanal obstruction resulting from failure of bony or membranous regression is the most common supralaryngeal congenital defect. It occurs in approximately 1 in 4000 live births. Choanal atresia is often associated with defects in other organs or with syndromes that include other craniofacial anomalies, especially CHARGE syndrome (i.e., coloboma of the eye, heart anomaly, choanal atresia, retardation, and genital and ear anomalies). Choanal atresia is usually unilateral, typically on the right side; a 2:1 excess of choanal atresia has been reported in girls, though a recent review suggests equal sex predilection.248 Unilateral choanal atresia occurs more frequently in isolated cases, whereas bilateral choanal atresia is an indication for further evaluation for other congenital anomalies. This phenomenon may be partly explained by the idea that genetic factors are more likely to result in symmetric errors of embryogenesis.249 Associated findings include a high-arched palate, thickening of the vomer, and medial bowing of the lateral wall of the nose. Clinical presentation depends on the degree of obstruction; bilateral choanal atresia or severe bilateral stenosis will present in the newborn period, whereas unilateral cases or mild stenosis may not present for weeks, months, or years. Infants with symptoms are typically distressed during times of sleep or feeding, when nasal breathing is preferential. Clinical presentation in infants with unilateral obstruction or mild stenosis may occur only when the nares become obstructed, as with the passage of a nasogastric tube or inflammation during an upper respiratory infection. With occlusion of the patent nares, an infant can suddenly decompensate, with signs of severe respiratory distress.
Tracheoesophageal Fistula
Tracheoesophageal fistula (TEF) occurs in approximately 1 in 4500 live births, making it one of the most common congenital malformations. Usually isolated, it can be associated with other anomalies including complex syndromes like VATER (vertebral defects, anal atresia, TEF, esophageal atresia, and renal anomalies), VACTERL (VATER plus cardiac and upper limb defects), and CHARGE (coloboma, heart defect, choanal atresia, mental retardation, genital hypoplasia, ear anomalies, and deafness). In the absence of a more generalized syndrome, TEF is also associated with isolated cardiac defects, which may present in up to 50% of cases. Whether as part of a syndrome or an isolated anomaly, TEF usually is associated with esophageal atresia; however, in 5% to 7% of cases there is no associated esophageal atresia (H-type TEF).
Congenital Anomalies of the Chest Wall
Thoracic cage abnormalities represent a group of uncommon but often overlooked causes of respiratory distress in the neonate and can be classified as either structural or functional. Structural abnormalities may be limited to the sternum or involve the entire thoracic cage. Sternal deformities include pectus excavatum, a relatively common but usually benign condition, and complete separation of the sternum, which usually leads to ectopia cordis and usually is lethal. Generalized structural abnormalities invariably involve some degree of thoracic restriction and pulmonary hypoplasia; many of these abnormalities are intrinsically lethal or are part of a more generalized lethal disorder. Some conditions, however, such as achondroplasia and Ellis-van Creveld syndrome, are compatible with normal life. Functional anomalies result from dysfunction of the chest wall musculature. Like structural defects, they can be isolated to the thoracic cage, but more often they are part of a systemic disorder, such as congenital muscular dystrophy, glycogen storage disease, or myasthenia gravis.
Persistent Pulmonary Hypertension of the Neonate
At birth, dramatic changes must occur within the lung if the fetus is to make a successful transition from placental to pulmonary gas exchange. Not only must liquid be removed from potential airspaces (see the section on Delayed Clearance of Fetal Lung Liquid in this chapter), but blood flow also must be redirected. After inflation of the lungs with air and dramatic increases in oxygen tension within the lungs, a marked increase in cyclic nucleotides occurs within the pulmonary vascular smooth muscle, leading to vasodilatation.250,251 The resultant drop in pulmonary vascular resistance leads to a tenfold increase in pulmonary blood flow,252 which causes left atrial pressure to exceed right atrial pressure, allowing the one-way flap across the foramen ovale to close. Flow across the ductus arteriosus reverses, and this, combined with the increase in oxygen tension in the blood, leads to gradual closure of the ductus over the first few hours of life.
In the syndrome of persistent pulmonary hypertension of the newborn (PPHN), this transition of the pulmonary circulation fails to occur normally (see Chapter 48). Pulmonary vascular resistance and pulmonary arterial pressure remain high and blood flow continues to bypass the lungs as in fetal life. (This is why the term persistent fetal circulation has been used, although it is not strictly correct because there is no longer any placental/umbilical circulation as in the fetus.) PPHN is associated with many neonatal disorders, including RDS, meconium aspiration, air leak syndromes, perinatal asphyxia, congenital sepsis, and structural lung disease such as pulmonary hypoplasia or alveolar-capillary dysplasia. PPHN can also be idiopathic. Infants with PPHN can be moderately or severely affected, depending on the degree of shunting. PPHN is often a self-limited disease, but this process may take several days; meanwhile, severe hypoxemia can lead to significant morbidity. The incidence of mortality can be as high as 50% to 60%; it has improved significantly with the advent of new treatment modalities such as HFV, inhaled nitric oxide, and extracorporeal bypass, which allow the infant more time to spontaneously recover.105,106,149,163,164,253–256 Because nitric oxide works by increasing cyclic guanosine monophosphate levels, there has been recent interest in using selective phosphodiesterase inhibitors such as sildenafil, particularly in infants with a prolonged course of PPHN, or in whom PPHN develops beyond the newborn period, either idiopathic or as a result of underlying cardiorespiratory disease. However, the long-term benefits and risks of sildenafil are unknown, and its use should still be considered experimental.
Congenital Heart Disease
In some types of congenital heart disease there is an overlap in presentation, and infants will appear cyanotic and have signs of respiratory distress such as tachypnea and perhaps even retractions. This type of presentation, which is common with hypoplastic left heart syndrome and total obstructed anomalous pulmonary venous return, may initially be confused with sepsis, pneumonia, meconium aspiration, or even RDS. Total anomalous pulmonary venous return (TAPVR) deserves particular mention because it cannot be detected in utero; it is often missed postnatally, even on repeated echocardiograms. In fact, this condition is the most common potentially correctable cardiac lesion for which neonates are mistakenly placed on ECMO. To diagnose TAPVR, one must keep a high index of suspicion, particularly in a term or near-term infant who has a clinical and radiographic picture of surfactant-deficient respiratory distress or group B streptococcal sepsis. If TAPVR is suspected and echocardiograms are not confirmatory, these infants must have a cardiac catheterization to make the diagnosis.
Metabolic Disorders
Several hundred inborn errors of metabolism (IEM) have been identified. Because they are a heterogeneous group, variable in presentation and relatively rare, they are frequently overlooked. Even with expanded newborn screening in many states, covering anywhere from 4 to 36 disease entities, results can be unavailable or difficult to interpret. An infant with an IEM may present with deep, labored respirations (Kussmaul breathing), if the underlying condition is associated with metabolic acidosis or increased intracranial pressure from cerebral edema. In many cases, this situation will rapidly progress to respiratory failure and apnea. Other manifestations of IEM result from either accumulation of a toxic product or deficiency of a necessary substrate. Although often present in the later course of the illness, many times these infants will not present with dysmorphic features, seizures, or vomiting. Rather, they display a pattern of worsening but nonspecific symptomatology that may not respond to routine intervention. It is important to maintain a high index of suspicion for IEMs, especially if there is no identified risk factor for respiratory distress, because a delay in diagnosis and treatment can be catastrophic.257
Intestinal and/or Renal Bicarbonate Wasting
Low plasma bicarbonate represents a metabolic acidosis that may present as tachypnea and respiratory distress in the neonate, although usually not in isolation. It is worth noting that metabolic acidosis with a normal anion gap may be a feature of neonatal diabetes or may be indicative of bicarbonate loss through the kidneys or intestines; gastroenteritis, renal tubular acidosis, and mineralocorticoid insufficiency are common causes.258 Differentiating the source of the acidosis is important because bicarbonate therapy may actually be harmful depending on the underlying problem.
References are available online at http://www.expertconsult.com.
1. Watchko J.F., Mayock D.E., Standaert T.A., et al. The ventilatory pump: neonatal and developmental issues. Adv Pediatr. 1991;38:109-134.
2. Alcorn D., Adamson T.M., Lambert T.F., et al. Morphological effects of chronic tracheal ligation and drainage in the fetal lamb lung. J Anat. 1977;123:649-660.
3. Bland R.D. Formation of fetal lung liquid and its removal near birth. In: Polin R.A., Fox W.W., editors. Fetal and neonatal physiology. ed 2. Philadelphia: WB Saunders; 1998:1047-1054.
4. Jain L. Alveolar fluid clearance in developing lungs and its role in neonatal transition. Clin Perinatol. 1999;26(3):585-599.
5. Avery M.E., Gatewood O.B., Brumley G. Transient tachypnea of the newborn. Am J Dis Child. 1966;111:380-385.
6. Olver R.E., Strang L.B. Ion fluxes across the pulmonary epithelium and the secretion of lung liquid in the foetal lamb. J Physiol (Lond). 1974;241:327-357.
7. Olver R.E., Ramsden C.A., Strang L.B., et al. The role of amiloride-blockable sodium transport in adrenaline-induced lung liquid reabsorption in the fetal lamb. J Physiol Lond. 1986;376:321-340.
8. Walters D.V., Olver R.E. The role of catecholamines in lung liquid absorption at birth. Pediatr Res. 1978;12:239-242.
9. Chapman D.L., Carlton D.P., Nielson D.W., et al. Changes in lung liquid during spontaneous labor in fetal sheep. J Appl Physiol. 1994;76:523-530.
10. McDonald J.V., Gonzales L.W., Ballard P.L., et al. Lung β-adrenoreceptor blockade affects perinatal surfactant release but not lung water. J Appl Physiol. 1986;60:1727-1733.
11. Cummings J.J., Carlton D.P., Poulain F.R., et al. Vasopressin effects on lung liquid volume in fetal sheep. Pediatr Res. 1995;38:30-35.
12. Wallace M.J., Hooper S.B., Harding R. Regulation of lung liquid secretion by arginine vasopressin in fetal sheep. Am J Physiol. 1990;258(27):R104-R111.
13. Cummings J.J., Carlton D.P., Poulain F.R., et al. Hypoproteinemia slows lung liquid clearance in young lambs. J Appl Physiol. 1993;74(1):153-160.
14. Raj J.U., Bland R.D. Lung liquid clearance in newborn lambs: effect of pulmonary microvascular pressure elevation. Am Rev Respir Dis. 1986;134:305-310.
15. Cummings J.J. Simultaneous increase in pulmonary blood flow and decrease in lung liquid production in late gestation fetal lambs. Am Rev Respir Dis. 1993;147(4):A417.
16. Egan E.A., Dillon W.P., Zorn S. Fetal lung liquid absorption and alveolar epithelial solute permeability in surfactant deficient, breathing lambs. Pediatr Res. 1984;18:566-570.
17. Guglani L., Lakshminrusimha S., Ryan R.M. Transient tachypnea of the newborn. Pediatr Rev. 2008;29:E59-E65.
18. Demissie K., Marcella S., Breckenridge M., et al. Maternal asthma and transient tachypnea of the newborn. Pediatrics. 1998;102(1):84-90.
19. Schatz M., Zeiger R., Hoffman C., et al. Increased transient tachypnea of the newborn in infants of asthmatic mothers. Am J Dis Child. 1991;145(2):156-158.
20. Birnkrant D., Picone C., Markowitz W., et al. Association of transient tachypnea of the newborn and childhood asthma. Pediatr Pulmonol. 2006;41(10):978-984.
21. Schaubel D., Johansen H., Dutta M., et al. Neonatal characteristics as risk factors for preschool asthma. J Asthma. 1996;33(4):255-264.
22. Miller M.J., Fanaroff A.A., Martin R.J. The respiratory system: other pulmonary problems. In Fanaroff A.A., Martin R.J., editors: Neonatal-perinatal medicine, ed 5, St Louis: Mosby, 1992.
23. Ogata E.S., Gregory G.A., Kitterman J.A., et al. Pneumothorax in respiratory distress syndrome: incidence and effect on vital signs, blood gases and pH. Pediatrics. 1976;58:177-182.
24. Mercier C.E., Soll R.F. Clinical trials of natural surfactant extract in respiratory distress syndrome. Clin Perinatol. 1993;20(4):711-735.
25. Kilbride H.W., Thibeault D.W. Neonatal complications of preterm premature rupture of membranes: pathophysiology and management. Clin Perinatol. 2001;28(4):761-785.
26. Wiswell T.E. Advances in the treatment of the meconium aspiration syndrome. Acta Paediatrica. 2001;90(436):28-30.
27. Plenat F., Vert P., Didier F., et al. Pulmonary interstitial emphysema. Clin in Perinatol. 1978;5(2):351-371.
28. Madansky D.L., Lawson E.E., Chernick V., et al. Pneumothorax and other forms of pulmonary air leak in newborns. Am Rev Resp Dis. 1979;120:729-737.
29. Gormally S.M., Clarke T.A., Krishnan A., et al. Surfactant therapy in respiratory distress syndrome: the effect of a learning curve in improving outcome. Irish J Med Sci. 1993;162(11):458-461.
30. Litmanovitz I., Carlo W.A. Expectant management of pneumothorax in ventilated neonates. Pediatrics. 2008;122:E975-E979.
31. Moessinger A., Driscoll J.J., Wigger H. High incidence of lung perforation by chest tube in neonatal pneumothorax. J Pediatr. 1978;92(4):635-637.
32. Swingle H.M., Eggert L.D., Bucciarelli R.L. New approach to management of unilateral tension pulmonary interstitial emphysema in premature infants. Pediatrics. 1984;74(3):354-357.
33. Keszler M., Donn S.M., Bucciarelli R.L., et al. Multicenter controlled trial comparing high-frequency jet ventilation and conventional ventilation in newborn infants with pulmonary interstitial emphysema. J Pediatr. 1991;119:85-93.
34. Keszler M., Durand D.J. Neonatal high-frequency ventilation: past, present, and future. Clin Perinatol. 2001;28(3):579-607.
35. van Houten J., Long W., Mullett M., et al. Pulmonary hemorrhage in premature infants after treatment with synthetic surfactant: an autopsy evaluation. J Pediatr. 1992;120(2 Pt 2):S40-S44.
36. Cole V.A., Normand I.C.S., Reynolds E.O.R., et al. Pathogenesis of hemorrhagic pulmonary edema and massive pulmonary hemorrhage in the newborn. Pediatrics. 1973;51(2):175-186.
37. Kluckow M., Evans N. Ductal shunting, high pulmonary blood flow, and pulmonary hemorrhage. J Pediatr. 2000;137(1):68-72.
38. Berger T., Allred E., van Marter L. Antecedents of clinically significant pulmonary hemorrhage among newborn infants. J Perinatol. 2000;20(5):295-300.
39. Lodha A., Shah P.S., Hellmann J. Pulmonary haemorrhage associated with neonatal neurological disease. Heart Lung Circ. 2009;18:45-48.
40. Duval E.L., Markhorst D.G., Ramet J., et al. High-frequency oscillatory ventilation in severe lung haemorrhage: a case study of three centres. Respir Med CME. 2009;2:92-98.
41. Aziz A., Ohlsson A., Surfactant for pulmonary hemorrhage in neonates, Cochrane Database Sys 2008:Rev 2;CD005254
42. Brady K.M., Easley R.B., Tobias J.D. Recombinant activated factor VII (rFVIIa) treatment in infants with hemorrhage. Pediatr Anesthesia. 2006;16:1042-1046.
43. Alfaleh K., Smyth J.A., Roberts R.S., et al. Prevention and 18-month outcomes of serious pulmonary hemorrhage in extremely low birth weight infants: results from the Trial of Indomethacin Prophylaxis in Preterms, Pediatrics. 2008;121:E233-E238.
44. Shi Y., Zhao J., Tang S., et al. Effect of hemocoagulase for prevention of pulmonary hemorrhage in critical newborns on mechanical ventilation: a randomized controlled trial. Indian Pediatr. 2008;45:199-202.
45. Akinbami L.J., Selby D.M., Slonim A.D. Hepatosplenomegaly and pulmonary infiltrates in an infant. J Pediatr. 2001;139(1):124-129.
46. Correa A.G. Unique aspects of tuberculosis in the pediatric population. Clin Chest Med. 1997;18(1):89-98.
47. Foglia E., Meier M., Edward A. Ventilator-associated pneumonia in neonatal and pediatric intensive care unit patients. Clin Micro Rev. 2007;20(3):409-425.
48. Stover B., Shulman S., Bratcher D., et al. Nosocomial infection rates in US children’s hospitals’ network and pediatric intensive care units. Am J Infect Control. 2001;29:152-157.
49. Yuan T.M., Chen L.H., Yu H.M. Risk factors and outcomes for ventilator-associated pneumonia in neonatal intensive care unit patients. J Perinat Med. 2007;35:334-338.
50. Schuchat A., Oxtoby M., Cochi S., et al. Population-based risk factors for neonatal group B streptococcal disease: results of a cohort study in metropolitan Atlanta. J Infect Dis. 1990;162:672-677.
51. Whitley R., Arvin A., Prober C., et al. Predictors of morbidity and mortality in neonates with herpes simplex virus infections. N Engl J Med. 1991;324:450-454.
52. Pulver L.S., Hopfenbeck M.M., Young P.C., et al. Continued early onset group B streptococcal infections in the era of intrapartum prophylaxis. J Perinatol. 2009;29:20-25.
53. Koenig J.M., Keenan W.J., Group B. Streptococcus and early-onset sepsis in the era of maternal prophylaxis. Pediatr Clin North Am. 2009;56:689-708.
54. Greenough A. Meconium aspiration syndrome—prevention and treatment. Early Hum Dev. 1995;41(3):183-192.
55. Wiswell T.E., Bent R.C. Meconium staining and the meconium aspiration syndrome. Pediatr Clin North Am. 1993;40(5):955-981.
56. Herting E., Rauprich P., Stichtenoth G., et al. Resistance of different surfactant preparations to inactivation by meconium. Pediatr Res. 2001;50(1):44-49.
57. Lam B.C., Yeung C.Y., Fu K.H., et al. Surfactant tracheobronchial lavage for the management of a rabbit model of meconium aspiration syndrome. Biol Neonate. 2000;78:129-138.
58. Okazaki K., Kondo M., Kato M., et al. Serum cytokine and chemokine profiles in neonates with meconium aspiration syndrome. Pediatrics. 2008;121:E748-E753.
59. Salvesen B., Fung M., Saugstad O.D., et al. Role of complement and CD14 in meconium-induced cytokine formation. Pediatrics. 2008;121:E496-E505.
60. Cayabyab R.G., Kwong K., Jones G., et al. Lung inflammation and pulmonary function in infants with meconium aspiration syndrome. Pediatr Pulmonol. 2007;42:898-905.
61. Gordon E., South M., McDougall P.N., et al. Blood aspiration syndrome as a cause of respiratory distress in the newborn infant. J Pediatr. 2003;142:200-202.
62. Vain N., Szyld E., Prudent L., et al. Oropharyngeal and nasopharyngeal suctioning of meconium stained neonates before delivery of their shoulders: multicentre, randomized controlled trial. Lancet. 2004;362:560-561.
63. Wiswell T.E., Gannon C.M., Jacob J., et al. Delivery room management of the apparently vigorous meconium-stained neonate: results of the multicenter, international collaborative trial. Pediatrics. 2000;105(1):1-7.
64. Niermeyer S., Kattwinkel J., Reempts P.V., et al. International Guidelines for Neonatal Resuscitation: an excerpt from the guidelines 2000 for cardiopulmonary resuscitation and emergency cardiovascular care: international consensus on science. Pediatrics. 2000;106(3):E29.
65. Fraser W., Hofmeyr J., Lede R., et al. Amnioinfusion for the prevention of the meconium aspiration syndrome. N Engl J Med. 2005;353(9):909-917.
66. Auten R.L., Notter R.H., Kendig J.W., et al. Surfactant treatment of full-term newborns with respiratory failure. Pediatrics. 1991;87:101-110.
67. Lam B.C., Yeung C.Y. Surfactant for meconium aspiration syndrome: a pilot study. Pediatrics. 1999;103(5):1014-1018.
68. El Shahed A., Dargaville P., Ohlsson A., et al. Surfactant for meconium aspiration syndrome in full term/near term infants. Cochrane Database Sys Rev. (3):2007. CD002054
69. Hung H., Jim W., Hsu C., et al. Small versus large volume dilute surfactant lavage for meconium aspiration syndrome. Acta Paediatr Taiwan. 2006;47(4):181-186.
70. Dargaville A., Mills J., Copnell B., et al. Therapeutic lung lavage in meconium aspiration syndrome: a preliminary report. J Paediatr Child Health. 2007;43:539-545.
71. Tripathi S., Saili A. The effect of steroids on the clinical course and outcome of neonates with meconium aspiration syndrome. J Tropical Pediatr. 2006;53(1):8-12.
72. Stenson B.J., Jackson A.D. Management of meconium aspiration syndrome. Paediatr Child Health. 2008;19(4):174-177.
73. Nava F.V., Ramírez E.S., Núncio H.R.S., et al. Meconium aspiration syndrome, parental atopy and asthma symptoms in children under two years old. Revista Alergia México. 2006;53(4):130-135.
74. Holm B.A., Waring A.J. Designer surfactants: the next generation in surfactant replacement. Clin Perinatol. 1993;20(4):813-829.
75. Jobe A.H., Ikegami M. Surfactant metabolism. Clin Perinatol. 1993;20(4):683-696.
76. Krauss A.N. Recent advances in hyaline membrane disease. Pediatr Ann. 1983;12(1):24-30.
77. Clark R.H., Auten R.L., Peabody J. A comparison of the outcomes of neonates treated with two different natural surfactants. J Pediatr. 2001;139:828-831.
78. Lemons J.A., Bauer C.R., Oh W., et al. Very low birth weight outcomes of the National Institute of Child Health and Human Development Neonatal Research Network, January 1995 through December 1996. Pediatrics. 2001;107(1):E1-E8.
79. Malloy M.H., Freeman D.H. Respiratory distress syndrome mortality in the United States, 1987 to 1995. J Perinatol. 2000;20:414-420.
80. Thomas N.J., Fan R., DiAngelo S., et al. Haplotypes of the surfactant protein genes A and D as susceptibility factors for the development of respiratory distress syndrome. Acta Pædiatrica. 2007;96:985-989.
81. Coleman M., Dehner L.P., Sibley R.K., et al. Pulmonary alveolar proteinosis: an uncommon cause of chronic neonatal respiratory distress. Am Rev Respir Dis. 1980;121:583-586.
82. Mahut B., deBlic J., LeBourgeois M., et al. Partial and massive lung lavages in an infant with severe pulmonary alveolar proteinosis. Pediatr Pulmonol. 1992;13:50-53.
83. Moulton S.L., Krous H.F., Merritt T.A., et al. Congenital pulmonary alveolar proteinosis: failure of treatment with extracorporeal life support. J Pediatr. 1992;120:297-302.
84. deMello D.E., Nogee L.M., Heyman S., et al. Molecular and phenotypic variability in the congenital alveolar proteinosis syndrome associated with inherited surfactant protein B deficiency. J Pediatr. 1994;125:43-50.
85. Nogee L.M., deMello D.E., Dehner L.P., et al. Brief report: deficiency of pulmonary surfactant protein B in congenital alveolar proteinosis. N Eng J Med. 1993;328(6):406-410.
86. Nogee L.M., Garnier G., Singer L., et al. A mutation in the surfactant protein B gene responsible for fatal neonatal respiratory disease in multiple kindreds. J Clin Invest. 1994;93:1860-1863.
87. Yusen R.D., Cohen A.H., Hamvas A. Normal lung function in subjects heterozygous for surfactant protein-B deficiency. Am J Respir Crit Care Med. 1999;159:411-414.
88. Hamvas A., Cole F.S., deMello D.E., et al. Surfactant protein B deficiency: antenatal diagnosis and prospective treatment with surfactant replacement. J Pediatr. 1994;125:356-361.
89. Palomar L.M., Nogee L.M., Sweet S.C., et al. Long-term outcomes after infant lung transplantation for surfactant protein B deficiency related to other causes of respiratory failure. J Pediatr. 2006;149:548-553.
90. Sherer D.M., Davis J.M., Woods J.R. Pulmonary hypoplasia: a review. Obstet Gynecol Surv. 1990;45:792-803.
91. Fewell J.E., Hislop A.A., Kitterman J.A., et al. Effect of tracheostomy on lung development in fetal lambs. J Appl Physiol. 1983;55(4):1103-1108.
92. Rigatto H. Maturation of breathing control in the fetus and newborn infant. In: Beckerman R.C., Brouillette R.T., Hunt C.E., editors. Respiratory control disorders in infants and children. Baltimore, MD: Williams & Wilkins; 1992:61-75.
93. Thomas I.T., Smith D.W. Oligohydramnios, cause of the nonrenal features of Potter’s syndrome, including pulmonary hypoplasia. J Pediatr. 1974;84:811-817.
94. Devine P.C., Malone F.D. Noncardiac thoracic anomalies. Clin Perinatol. 2000;27(4):865-900.
95. Lally K.P. Congenital diaphragmatic hernia. Curr Opin Pediatr. 2002;14(4):486-490.
96. Bohn D. Congenital diaphragmatic hernia. Am J Respir Crit Care Med. 2002;166:911-915.
97. Major D., Cadenas M., Fournier L., et al. Retinol status of newborn infants with congenital diaphragmatic hernia. Pediatr Surg Int. 1998;13:547-549.
98. Yang W., Shaw G., Carmichael S., et al. Nutrient intakes in women and congenital diaphragmatic hernia in their offspring. Birth Defects Res A Clin Mol Teratol. 2008;82(3):131-138.
99. Montedonico S., Sugimoto K., Felle P., et al. Prenatal treatment with retinoic acid promotes pulmonary alveologenesis in the nitrofen model of congenital diaphragmatic hernia. J Pediatr Surg. 2008;43:500-507.
100. Beckman D., Cummings J., Katwa L., et al. Can maternal vitamin E supplementation prevent lung hypoplasia in the nitrofen-induced rat model of congenital diaphragmatic hernia? Pediatr Res. 2005;57(3):392-395.
101. Gonzalez-Reyes S., Alvarez L., Diez-Pardo J., et al. Prenatal vitamin E improves lung and heart hypoplasia in experimental diaphragmatic hernia. Pediatr Surg Int. 2003;19:331-334.
102. Islam S., Narra V., Cote G., et al. Prenatal vitamin E treatment improves lung growth in fetal rats with congenital diaphragmatic hernia. J Pediatr Surg. 1999;34:172-176.
103. Leung J.W.T., Coakley F.V., Hricak H., et al. Prenatal MR imaging of congenital diaphragmatic hernia. Am J Roentgenol. 2000;174:1607-1612.
104. Cullen M.L., Klein M.D., Philippart A.I. Congenital diaphragmatic hernia. Surg Clin North Am. 1985;65(5):1115-1137.
105. Van Meurs K.P., Robbins S.T., Reed V.L., et al. Congenital diaphragmatic hernia: long-term outcome in neonates treated with extracorporeal membrane oxygenation. J Pediatr. 1993;122:893-899.
106. Clark R.H., Yoder B.A., Sell M.S. Prospective, randomized comparison of high-frequency oscillation and conventional ventilation in candidates for extracorporeal membrane oxygenation. J Pediatr. 1994;124(3):447-454.
107. Wilcox D.T., Glick P.L., Karamanoukian H., et al. Pathophysiology of congenital diaphragmatic hernia. v. effect of exogenous surfactant therapy on gas exchange and lung mechanics in the lamb congenital diaphragmatic hernia model. J Pediatr. 1994;124:289-293.
108. Van Meurs K., the Congenital Diaphragmatic Hernia Study Group. Is surfactant therapy beneficial in the treatment of the term newborn infant with congenital diaphragmatic hernia? J Pediatr. 2004;145:312-316.
109. Harrison M.R., Adzick N.S., Longaker M.T., et al. Successful repair in utero of a fetal diaphragmatic hernia after removal of herniated viscera from the left thorax. N Engl J Med. 1990;322:1582-1589.
110. Walker G.M., Kasem K.F., O’Toole S.J., et al. Early perfluorodecalin lung distension in infants with congenital diaphragmatic hernia. J Pediatr Surg. 2003;38(1):17-20.
111. Moyer V., Moya F., Tibboel R., et al. Late versus early surgical correction for congenital diaphragmatic hernia in newborn infants. Cochrane Database Sys Rev. 2002;3:CD001695.
112. Sakai H., Tamura M., Hosokawa Y., et al. Effect of surgical repair on respiratory mechanics in congenital diaphragmatic hernia. J Pediatr. 1987;111:432-438.
113. Nakayama D.K., Motoyama E.K., Tagge E.M. Effect of preoperative stabilization on respiratory system compliance and outcome in newborn infants with congenital diaphragmatic hernia. J Pediatr. 1991;118:793-799.
114. Kunisaki S.M., Barnewolt C.E., Estroff J.A., et al. Ex utero intrapartum treatment with extracorporeal membrane oxygenation for severe congenital diaphragmatic hernia. J Pediatr Surg. 2007;42:98-106.
115. Laberge J.M., Flageole H., Pukash D., et al. Outcome of the prenatally diagnosed congenital cystic adenomatoid lung malformation: a Canadian experience. Fetal Diagn Ther. 2001;16(3):178-186.
116. Hernanz-Schulman M. Cysts and cystlike lesions of the lung. Radiol Clin North Am. 1993;31:631-649.
117. Kravitz R.M. Congenital malformations of the lung. Pediatr Clin North Am. 1994;41:453-472.
118. Cha I., Adzick N., Harrison M., et al. Fetal congenital cystic adenomatoid malformations of the lung: a clinicopathologic study of eleven cases. Am J Surg Pathol. 1997;21:537-544.
119. Kreiger P.A., Ruchelli E.D., Mahboubi S., et al. Fetal pulmonary malformations: defining histopathology. Am J Surg Pathol. 2006;30(5):643-649.
120. Vu L.T., Farmer D.L., Nobuhara K.K., et al. Thoracoscopic versus open resection for congenital cystic adenomatoid malformations of the lung. J Pediatr Surg. 2008;43:35-39.
121. Nagata K., Masumoto K., Tesiba R., et al. Outcome and treatment in an antenatally diagnosed congenital cystic adenomatoid malformation of the lung. Pediatr Surg Int. 2009;25:753-757.
122. Williams H.J., Johnson K.J. Imaging of congenital cystic lung lesions. Paediatr Respir Rev. 2002;3(2):120-127.
123. Ryckman F.C., Rosenkrantz J.G. Thoracic surgical problems in infancy and childhood. Surg Clin North Am. 1985;65:1423-1454.
124. Kugelman A., Weinger-Abend M., Miselevich I., et al. Acquired pulmonary cysts in the newborn infant. Pediatr Pulmonol. 1997;24(4):298-301.
125. Zeidan S., Hery G., Lacroix F., et al. Intralobar sequestration associated with cystic adenomatoid malformation: diagnostic and thoracoscopic pitfalls. Surg Endosc. 2009;23:1750-1753.
126. Jesch N.K., Leonhardt J., Sumpelmann R., et al. Thoracoscopic resection of intra- and extralobar pulmonary sequestration in the first 3 months of life. J Pediatr Surg. 2005;40:1404-1406.
127. Kestenholz P.B., Schneiter D., Hillinger S., et al. Thoracoscopic treatment of pulmonary sequestration. Eur J Cardiothorac Surg. 2006;29:815-818.
128. Rothenberg S.S. First decade’s experience with thoracoscopic lobectomy in infants and children. J Pediatr Surg. 2008;43:40-45.
129. Lee B.S., Kim J.T., Kim E.A., et al. Neonatal pulmonary sequestration: clinical experience with transumbilical arterial embolization. Pediatr Pulmonol. 2008;43:404-413.
130. Rahman N., Lakhoo K. Comparison between open and thoracoscopic resection of congenital lung lesions. J Pediatr Surg. 2009;44:333-336.
131. Meir-Zahav M., Konen O., Manson D., et al. Is congenital lobar emphysema a surgical disease? J Pediatr Surg. 2006;41(6):1058-1061.
132. Chitayat D., Sroka H., Keating S., et al. The PDAC syndrome (pulmonary hypoplasia/agenesis, diaphragmatic hernia/eventration, anophthalmia/microphthalmia, and cardiac defect) (Spear syndrome, Matthew-Wood syndrome): report of eight cases including a living child and further evidence for autosomal recessive inheritance. Am J Med Genet Part A. 2007;143A:1268-1281.
133. Bentsianov B.L., Goldstein N.A., Giuste R., et al. Unilateral pulmonary agenesis presenting as an airway lesion. Arch Otolaryngol. 2000;126(11):1386-1389.
134. Aspirot A., Puligandla P.S., Bouchard S., et al. A contemporary evaluation of surgical outcome in neonates and infants undergoing lung resection. J Pediatr Surg. 2008;43:508-512.
135. Bromley B., Benacerraf B.R. Unilateral lung hypoplasia: report of three cases. J Ultrasound Med. 1997;16(9):599-601.
136. Shaw T.R. Displacement of the heart caused by pulmonary agenesis. Heart. 2002;88(3):277.
137. Turell D.C. Advances with surfactant. Emerg Med Clin North Am. 2008;26(4):921-928.
138. Cummings J.J. Surfactant therapy in neonates and beyond. J Respir Care Pract. 1999;6(7):77-84.
139. Khammash H., Perlman M., Wojtulewicz J., et al. Surfactant therapy in full-term infants with severe respiratory failure. Pediatrics. 1993;92:135-139.
140. Notter R.H., Holm B.A., Willson D., et al. Surfactant therapy and its potential use with other agents in term neonates, children, and adults with acute lung injury. Perspect Neonatol. 2000;1(4):4-20.
141. Tibby S.M., Hatherill M., Wright S.M., et al. Exogenous surfactant supplementation in infants with respiratory syncytial virus bronchiolitis. Am J Respir Crit Care Med. 2000;162:1251-1256.
142. Golombek S.G., Truog W.E. Effects of surfactant treatment on gas exchange and clinical course in near-term newborns with RDS. J Perinat Med. 2000;28:436-442.
143. Marraro G.A., Luchetti M., Galassini E.M., et al. Natural surfactant supplementation in ARDS in paediatric age. Minerva Anestesiol. 1999;65(Suppl 1):92-97.
144. Willson D.F., Zaritsky A., Bauman L.A., et al. Instillation of calf lung surfactant extract (calfactant) is beneficial in pediatric acute hypoxemic respiratory failure. Crit Care. 1999;27:188-195.
145. Lotze A., Mitchell B.R., Bulas D.I., et al. Multicenter study of surfactant (beractant) use in the treatment of term infants with severe respiratory failure. J Pediatr. 1998;132:40-47.
146. McDonald C., Ainsworth S. An update on the use of surfactant in neonates. Curr Paed. 2004;14(4):284-289.
147. Ringer S.A. High-frequency ventilation: the realization of the potential. Perspect Neonatol. 2000;1(5):1-16.
148. Cotten M., Clark R.H. The science of neonatal high-frequency ventilation. Respir Care Clin North Am. 2001;7(4):611-631.
149. Frantz III ID: High-frequency ventilation, Neonatal Respir Dis 5:1–11.
150. Clark R.H. High-frequency ventilation. J Pediatr. 1994;124(5):661-670.
151. Plavka R., Kopecky P., Sebron V., et al. A prospective randomized comparison of conventional ventilation and very early high frequency oscillatory ventilation in extremely premature newborns with respiratory distress syndrome. Intensive Care Med. 1999;25(1):68-75.
152. HIFO Study Group. Randomized study of high-frequency oscillatory ventilation in infants with severe respiratory distress syndrome. J Pediatr. 1993;122:609-619.
153. Moriette G., Paris-Llado J., Walti H., et al. Prospective randomized multicenter comparison of high-frequency oscillatory ventilation and conventional ventilation in preterm infants of less than 30 weeks with respiratory distress syndrome. Pediatrics. 2001;107(2):363-372.
154. Wiswell T.E., Graziani L.J., Kornhauser M.S., et al. High-frequency jet ventilation in the early management of respiratory distress syndrome is associated with a greater risk for adverse outcomes. Pediatrics. 1996;98:1035-1043.
155. Courtney S.E., Durand D.J., Asselin J.M., et al. High-frequency oscillatory ventilation versus conventional mechanical ventilation for very-low-birth-weight infants. N Engl J Med. 2002;347(9):643-652.
156. Gertsmann D.R., Minton S.D., Stoddard R.A., et al. The Provo multicenter early high-frequency oscillatory ventilation trial: improved pulmonary and clinical outcome in respiratory distress syndrome. Pediatrics. 1996;98:1044-1057.
157. Johnson A.H., Peacock J.L., Greenough A., et al. High-frequency oscillatory ventilation for the prevention of chronic lung disease of prematurity. N Engl J Med. 2002;347(9):633-642.
158. Keszler M., Modanlou H.D., Brudno D.S., et al. Multi-center controlled clinical trial of high-frequency jet ventilation in preterm infants with uncomplicated respiratory distress syndrome. Pediatrics. 1997;100:593-599.
159. Ogawa Y., Miyasaka K., Kawano T., et al. A multicenter randomized trial of high frequency oscillatory ventilation as compared with conventional mechanical ventilation in preterm infants with respiratory failure. Early Hum Dev. 1993;32:1-10.
160. The NINOS Study Group. Inhaled nitric oxide for near-term infants with respiratory failure. N Engl J Med. 1997;336:602-605.
161. Todd M.M., Toutant S.M., Shapiro H.M. The effects of high-frequency ventilation on intracranial pressure and brain surface movement in cats. Anesthesiology. 1981;54:496-504.
162. Walker A.M., Brodecky V.A., Preu N.D., et al. High frequency oscillatory ventilation compared with conventional mechanical ventilation in newborn lambs: effects of increasing airway pressures on intracranial pressures. Pediatr Pulmonol. 1992;12(1):11-16.
163. Klein M.D., Whittlesey G.C. Extracorporeal membrane oxygenation. Pediatr Clin North Am. 1994;41(2):365-384.
164. Kanto W.P. A decade of experience with neonatal extracorporeal membrane oxygenation. J Pediatr. 1994;124(3):335-347.
165. Meyer T.A., Warner B.A. Extracorporeal life support for the treatment of viral pneumonia: collective experience from the ELSO registry, J Pediatr Surg. 1997;32(2):232-236.
166. Steinhorn R.H., Isham-Schopf B., Smith C., et al. Complications of neonatal extracorporeal membrane oxygenation. J Pediatr. 1990;116(6):1006-1007.
167. Bahrami K.R., Van Meurs K.P. ECMO for neonatal respiratory failure. Semin Perinatol. 2005;29(1):15-23.
168. Cummings J.J. Nitric oxide decreases lung liquid production in fetal lambs. J Appl Physiol. 1997;83:1538-1544.
169. Abman S.H., Kinsella J.P., Parker T.P., et al. Physiologic roles of nitric oxide in the perinatal pulmonary circulation. In: Weir E.K., Archer S.L., Reeves J.T., editors. Fetal and neonatal pulmonary circulations. New York: Futura; 1999:239-260.
170. Kinsella J.P., Walsh W.F., Bose C., et al. Randomized controlled trial of inhaled nitric oxide in premature neonates with severe hypoxemic respiratory failure. Lancet. 1999;354:1061-1065.
171. Van Meurs K.P., Rhine W.D., Asselin J.M., et al. Response of premature infants with severe respiratory failure to inhaled NO. Pediatr Pulmonol. 1997;24:319-323.
172. Watchko J.F. Persistent pulmonary hypertension in a very low birthweight preterm infant. Clin Pediatr. 1985;24(10):592-595.
173. Walther F.J., Benders M.J., Leighton J.O. Persistent pulmonary hypertension in premature neonates with severe respiratory distress syndrome. Pediatrics. 1992;90(6):899-904.
174. Kinsella J.P., Ivy D.D., Abman S.H. Inhaled nitric oxide improves gas exchange and lowers pulmonary vascular resistance in severe experimental hyaline membrane disease. Pediatr Res. 1994;36(3):402-408.
175. Zapol W.M., Rimar S., Gillis N., et al. Nitric oxide and the lung. Am J Respir Crit Care Med. 1994;149:1375-1380.
176. Abman S.H., Kinsella J.P. Nitric oxide in the pathophysiology and treatment of neonatal pulmonary hypertension. Neonatal Respir Dis. 1994;4:1-11.
177. Abman S.H., Kinsella J.P. Is there a role for nitric oxide therapy in premature neonates? Perspect Neonatol. 2001;2:4-16.
178. Kinsella J.P. Inhaled nitric oxide: current and future uses in neonates. Semin Perinatol. 2000;24(6):387-395.
179. Greenspan J., Wolfson M., Shaffer T. Liquid ventilation. Semin Perinatol. 2000;24:396-405.
180. Fuhrman B.P., Paczan P.R., DeFrancisis M. Perfluorocarbon associated gas exchange. Crit Care Med. 1991;19(5):712-723.
181. Burkhardt W., Proquitte H., Krause S., et al. Cerebral oxygenation is affected by filing mode and perfluorochemical volume in partial liquid ventilation in healthy piglets. Biol Neonate. 2002;82(4):250-256.
182. Tutuncu A.S., Houmes R.J., Bos J.A., et al. Evaluation of lung function after intratracheal perfluorocarbon administration in healthy animals. Crit Care Med. 1996;24(2):274-279.
183. Colton D.M., Till G.O., Johnson K.J., et al. Neutrophil accumulation is reduced during partial liquid ventilation. Crit Care Med. 1998;26(10):1716-1724.
184. Haeberle H.A., Nesti N., Dieterich H.J., et al. Perflubron reduces lung inflammation in respiratory syncytial virus infection by inhibiting chemokine expression and nuclear factor-kappa B activation. Am J Resp Crit Care Med. 2002;165(10):1433-1438.
185. Merz U., Klosterhalfen B., Hausler M., et al. Partial liquid ventilation reduces release of leukotriene B4 and interleukin-6 in bronchoalveolar lavage surfactant-depleted newborn pigs. Pediatr Res. 2002;51(2):183-189.
186. Rotta A.T., Steinhorn D.M. Partial liquid ventilation reduces pulmonary neutrophil accumulation in an experimental model of systemic endotoxemia and acute lung injury. Crit Care Med. 1998;26(10):1707-1715.
187. Steinhorn D.M., Papo M.C., Rotta A.T., et al. Liquid ventilation attenuates pulmonary oxidative damage. J Crit Care. 1999;14(1):20-28.
188. Degraeuwe P.L., Thunnissen F.B., Jansen N.J., et al. Conventional gas ventilation, liquid-assisted high-frequency oscillatory ventilation, and tidal liquid ventilation in surfactant-treated preterm lambs. Int J Artificial Organ. 2000;23(11):754-764.
189. Jeng M., Kou Y.R., Sheu C.C., et al. Effects of partial liquid ventilation with FC-77 on acute lung injury in newborn piglets. Pediatr Pulmonol. 2002;33(1):12-21.
190. Leach C.L., Fuhrman B.P. Perfluorocarbon associated gas exchange (PAGE) in surfactant deficiency. Am Rev Respir Dis. 1992;145:A454.
191. Lewis D.A., Colton D., Johnson K., et al. Prevention of ventilator-induced lung injury with partial liquid ventilation. J Pediatr Surg. 2001;36(9):1333-1336.
192. Vazquez de Anda G.F., Lachmann R.A., Verbrugge S.J., et al. Partial liquid ventilation improves lung function in ventilation-induced lung injury. Eur Resp J. 2001;18(1):93-99.
193. Kallas H.J. Non-conventional respiratory support modalities applicable in the older child: high frequency ventilation and liquid ventilation. Crit Care Clin. 1998;14(4):655-683.
194. Leach C.L., Greenspan J.S., Rubenstein S.D., et al. Partial liquid ventilation with perflubron in premature infants with severe respiratory distress syndrome. N Engl J Med. 1996;335(11):761-767.
195. Ricard J., Lemiare F. Liquid ventilation. Curr Opin Crit Care. 2001;7:8-14.
196. Dani C., Reali M.F., Bertini G., et al. Liquid ventilation in an infant with persistent interstitial pulmonary emphysema. J Perinatal Med. 2001;29(2):158-162.
197. Fedora M., Nekvasil R., Seda M., et al. Partial liquid ventilation in the therapy of pediatric acute respiratory distress syndrome. Bratislavske Lekarske Listy. 1999;100(9):481-485.
198. Hirschl R.B., Conrad S., Kaiser R., et al. Partial liquid ventilation in adult patients with ARDS: a multicenter phase I-II trial. Ann Surg. 1998;228(5):692-700.
199. Hirschl R.B., Croce M., Gore D., et al. Prospective, randomized, controlled pilot study of partial liquid ventilation in adult respiratory distress syndrome. Am J Resp Crit Care Med. 165(6), 2002. 787
200. Sands T.L., Miller K.J., Fischer J. Liquid ventilation: a case study. Am J Crit Care. 2000;9(6):397-402.
201. Northway W.H., Rosan R.C., Porter D.Y. Pulmonary disease following respiratory therapy of hyaline membrane disease: bronchopulmonary dysplasia. N Engl J Med. 1967;276:357-368.
202. Bancalari E., Gerhardt T. Bronchopulmonary dysplasia. Pediatr Clin North Am. 1986;33(1):1-23.
203. O’Brodovich H.M., Mellins R.B. Bronchopulmonary dysplasia: unresolved neonatal acute lung injury. Am Rev Respir Dis. 1985;132:694-709.
204. Baraldi E., Fillipone M. Chronic lung disease after premature birth. N Engl J Med. 2007;357(19):1946-1955.
205. Bland R.D. Neonatal chronic lung disease in the post-surfactant era. Biol Neonate. 2005;88(3):181-191.
206. Bland R.D., Coalson J.J., editors. Chronic lung disease of infancy. New York: Dekker, 2000.
207. Jobe A.J. The new BPD: an arrest of lung development. Pediatr Res. 1999;46(6):641-643.
208. Bhatt A.J., Pryhuber G.S., Huyck H., et al. Disrupted pulmonary vasculature and decreased vascular endothelial growth factor, Flt-1, and TIE-2 in human infants dying with bronchopulmonary dysplasia. Am J Respir Crit Care Med. 2001;164:1971-1980.
209. Clark R.H., Gerstmann D.R., Jobe A.H., et al. Lung injury in neonates: causes, strategies for prevention, and long-term consequences. J Pediatr. 2001;139(4):478-486.
210. Copland I.B., Post M. Understanding the mechanisms of infant respiratory distress and chronic lung disease. Am J Resp Cell Mol Biol. 2002;26:261-265.
211. Denne S.C. Energy expenditure in infants with pulmonary insufficiency: is there evidence for increased energy needs? J Nutr. 2001;131:S935-S937.
212. Hjalmarson O., Sandberg K. Abnormal lung function in healthy preterm infants. Am J Respir Crit Care Med. 2002;165:83-87.
213. Jobe A.H., Ikegami M. Mechanisms initiating lung injury in the preterm. Early Hum Dev. 1998;53:81-94.
214. Kennedy K.A. Epidemiology of acute and chronic lung injury. Semin Perinatol. 1993;117(4):247-252.
215. Lassus P., Turanlahti M., Heikkilä P., et al. Pulmonary vascular endothelial growth factor and Flt-1 in fetuses, in acute and chronic lung disease, and in persistent pulmonary hypertension of the newborn. Am J Respir Crit Care Med. 2001;164:1981-1987.
216. Poets C.F. When do infants need additional inspired oxygen? A review of the current literature. Pediatr Pulmonol. 1998;26:424-428.
217. Rozycki H.J. Oxygen, oxygen radicals, and inflammation in the development of chronic lung disease. Neonatal Respir Dis. 2002:1-8.
218. Speer C.P. New insights into the pathogenesis of pulmonary inflammation in preterm infants. Biol Neonate. 2001;79:205-209.
219. Vaucher Y.E. Bronchopulmonary dysplasia: an enduring challenge. Pediatr Rev. 2002;23(10):349-358.
220. Bancalari E., Sosenko I. Pathogenesis and prevention of neonatal chronic lung disease: recent developments. Pediatr Pulmonol. 1990;8:109-116.
221. Cummings J.J., D’Eugenio D.B., Gross S.J. A controlled trial of dexamethasone in preterm infants at high risk for bronchopulmonary dysplasia. N Engl J Med. 1989;320:1505-1510.
222. Harkavy K.L., Scanlon J.W., Chowdry P.K., et al. Dexamethasone therapy for chronic lung disease in ventilator- and oxygen-dependent infants: a controlled trial. J Pediatr. 1989;115:979-983.
223. Garland J.S., Alex C.P., Pauly T.H., et al. A three-day course of dexamethasone therapy to prevent chronic lung disease in ventilated neonates: a randomized trial. Pediatrics. 1999;104(1):91-99.
224. O’Shea T.M., Kothadia J.M., Klinepeter K.L., et al. Randomized placebo-controlled trial of a 42-day tapering course of dexamethasone to reduce the duration of ventilator dependency in very low birth weight infants: outcome of study participants at 1-year adjusted age. Pediatrics. 1999;104(1):15-21.
225. Shinwell E.S., Karplus M., Reich D., et al. Early postnatal dexamethasone treatment and increased incidence of cerebral palsy. Arch Dis Child Fetal Neonatal Educ. 2000:F177-F181. 83
226. Committee on Fetus and Newborn. Postnatal corticosteroids to treat or prevent chronic lung disease in preterm infants. Pediatrics. 2002;109(2):330-338.
227. Suchomski S.J., Cummings J.J. A randomized trial of inhaled versus intravenous steroids in ventilator-dependent preterm infants. J Perinatol. 2002;22:196-203.
228. Jankov R.P., Negus A., Tanswell A.K. Antioxidants as therapy in the newborn: some words of caution. Pediatr Res. 2001;50:681-687.
229. Mazursky J.E., Klein J.M. Techniques to counter neonatal pulmonary disease. Contemp OB GYN Dec. 1994:11-27.
230. Rush M.G., Hazinski T.A. Current therapy of bronchopulmonary dysplasia. Clin Perinatol. 1992;19(3):563-590.
231. Truog W.E., Jackson J.C. Alternative modes of ventilation in the prevention and treatment of bronchopulmonary dysplasia. Clin Perinatol. 1992;19(3):621-647.
232. Jobe A.H., Bancalari E. Bronchopulmonary dysplasia: a NICHD/NHLBI/ORD workshop summary. Am J Respir Crit Care Med. 2001;163:1723-1729.
233. Furman L., Bayley J., Borawski-Clark E., et al. Hospitalization as a measure of morbidity among very low birth weight infants with chronic lung disease. J Pediatr. 1995;128(4):447-452.
234. Evans M., Palta M., Sadek M., et al. Associations between family history of asthma, bronchopulmonary dysplasia, and childhood asthma in very low birth weight children. Am J Epidemiol. 1998;148:460-466.
235. Gross S.J., Iannuzzi D.M., Kveselis D.A., et al. Effect of preterm birth on pulmonary function at school age: a prospective controlled study. J Pediatr. 1998;133:188-192.
236. Hofhuis W., Huysman M.W.A., Wiel E.C.vd., et al. Worsening of VmaxFRC in infants with chronic lung disease in the first year of life. Am J Respir Crit Care Med. 2002;166:1539-1543.
237. Subhedar N.V., Shaw N.J. Changes in pulmonary arterial pressure in preterm infants with chronic lung disease, Arch Dis Child Fetal Neonatal Educ. 2000;82:F243-F247.
238. Committee on Infectious Diseases and Committee on Fetus and Newborn. Revised indications for the use of palivizumab and respiratory syncytial virus immune globulin intravenous for the prevention of respiratory syncytial virus infections. Pediatrics. 2003;112:1447-1452.
239. Carter B.S. Palivizumab (synagis): counting “costs” and values. Pediatrics. 2000;106(5):1168-1169.
240. Lee S.L., Robinson J.L. Questions about palivizumab (Synagis) [comment]. Pediatrics. 1999;103(2):535.
241. Huber A., Schranz D., Blaha I., et al. Congenital pulmonary lymphangiectasia. Pediatr Pulmonol. 1991;10:310-313.
242. Ergaz Z., Bar-Oz B., Yatsiv I., et al. Congenital chylothorax: clinical course and prognostic significance. Pediatr Pulmonol. 2009;44(8):806-811.
243. Lumbreras Fernandez J., Sanchez Diaz J.I. Chylothorax: experience in a pediatric intensive care unit [in Spanish]. An Pediatr (Barc). 2009;70(3):223-229.
244. Scully R.E., Mark E.J., McNeely W.F., et al. Case records of the Massachusetts General Hospital case 13-1992. N Engl J Med. 1992;326(13):875-884.
245. Mettauer N., Agarwal S., Pierce C., et al. Outcome of children with pulmonary lymphangiectasis. Pediatr Pulmonol. 2009;44(4):351-357.
246. Bakewell-Sachs S., Medoff-Cooper B., Escobar G.J., et al. Infant functional status: the timing of physiologic maturation of premature infants. Pediatrics. 2009;123(5):E878-E886.
247. Caddell J.L. Magnesium therapy in infants with postneonatal apnea. Magnesium. 1988;7(2):103-116.
248. Harris J., Robert E., Kallen B.Epidemiology of choanal atresia with special reference to CHARGE association Pediatrics 1997:99;363-367
249. Burrow T.A., Saal H.M., de Alarcon A., et al. Characterization of congenital anomalies in individuals with choanal atresia. Arch Otolaryngol Head Neck Surg. 2009;135(6):543-547.
250. Cornfield D.N., Chatfield B.A., McQueston J.A., et al. Effects of birth-related stimuli on L-arginine-dependent pulmonary vasodilation in ovine fetus. Am J Physiol. 1992;262(31):H1474-H1481.
251. Morin F.C.III, Tiktinsky M.H. Oxygenation increases fetal pulmonary blood flow via endothelium dependent vasodilation. Pediatr Res. 1992;31:1405A.
252. Heymann M.A. Control of the pulmonary circulation in the perinatal period. J Develop Physiol. 1984;6:281-290.
253. Clark D.A., Nieman G.F., Thompson J.E., et al. Surfactant displacement by meconium free fatty acids: an alternative explanation for atelectasis in meconium aspiration syndrome. J Pediatr. 1987;110:765-770.
254. Durand D.J., Asselin J.M., Courtney S.E., et al. Randomized study of high-frequency oscillatory ventilation in infants with severe respiratory distress syndrome. J Pediatr. 1993;122:609-619.
255. Perez-Benavides F., Boynton B., Desai N.S. Persistent pulmonary hypertension (PPHN): comparison of conventional treatment vs. extracorporeal membrane oxygenation (ECMO) in neonates fulfilling Bartlett’s criteria. Clin Res. 1990;38(1):A65A.
256. Subramanian K.N.S., Keszler M., Hoy G. ECMO for severe neonatal respiratory failure. Cont Obstet Gynecol. 1986;28:21-37.
257. Colletti J.E., Homme J.L., Woodridge D.P. Unsuspected neonatal killers in emergency medicine. Emerg Med Clin North Am. 2004;22(4):929-960.
258. Kwon K., Tsai W. Metabolic emergencies. Emerg Med Clin North Am. 2007;25(4):1041-1060.