Monitoring Systems, Catheters, and Devices in the Intensive Care Unit
Familiarity with the extensive monitoring facilities in the ICU relieves some of the apprehensions the physical therapist may have about practicing in this setting. Such monitoring is central to the standard of physical therapy care in the ICU to guide progressive mobilization and management overall.1 On introduction to the unit, the physical therapist is immediately struck by the high-tech environment. Quality care in this setting depends on harnessing the potential of high-tech monitoring equipment to optimize assessment, evaluate treatment parameters, and establish anticipated and actual effectiveness of intervention, as well as to reduce untoward risk for the patient.
Figure 16-1 illustrates a general view of a typical ICU. A view of a patient’s bedside area in the ICU shows life-support equipment and the various lines and catheters that are in place (Figure 16-2). A closer view of the patient demonstrates precisely where the various lines and catheters are positioned and identifies where caution must be observed. Treatments are modified according to the types and positions of the lines and catheters for each patient (Figure 16-3).
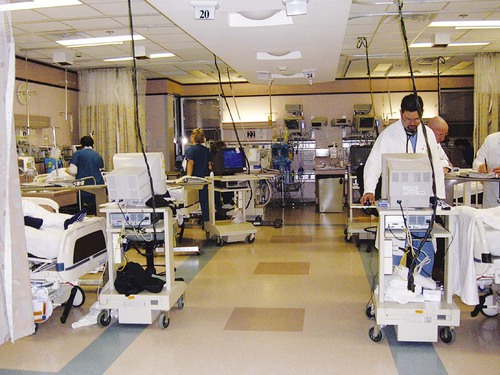
Fluid and Electrolyte Balance
Imbalances are reflected as excesses, deficits, or as an abnormal distribution of fluids within the body.2 Excesses result from increased intake and decreased loss of fluid and electrolytes. Deficits result from abnormal shifts of fluid and electrolytes among the intravascular and extravascular fluid compartments of the body. Excesses can occur with kidney dysfunction, promoting fluid retention, and with respiratory dysfunction, which promotes carbon dioxide retention. Deficits are commonly associated with reduced intake of fluids and nutrition. Diaphoresis and wounds can also contribute to major fluid loss. Diarrhea and vomiting drain the gastrointestinal tract of fluid. Hemorrhage always results in fluid and electrolyte loss. Deficits may be secondary to fluid entrapment and localized edema within the body (third-spacing), making this source of fluid unavailable for regulation of homeostasis.
Assessment of fluid and electrolyte balance is based on both subjective and objective findings (Table 16-1). At the bedside, the physical therapist must be alert to complaints of headache, thirst, and nausea, as well as changes in dyspnea, skin turgor, and muscle strength. More objective assessment is based on fluid intake, output, and body weight. Fluid balance is so critical to physical well-being and cardiovascular and pulmonary sufficiency that fluid input and output records are routinely maintained at bedside. These records also include fluid volume lost in urine and feces, wound drainage, and fluids aspirated from any body cavity (e.g., abdomen and pleural space).
Table 16-1
Assessment of Fluid and Electrolyte Imbalance
Area | Fluid Excess/Electrolyte Imbalance | Fluid Loss/Electrolyte Imbalance |
Head and neck | Distended neck veins, facial edema | Thirst, dry mucous membranes |
Extremities | Dependent edema “pitting,” discomfort from weight of bed covers | Muscle weakness, tingling, tetany |
Skin | Warm, moist, taut, cool feeling when edematous | Dry, decreased turgor |
Respiration | Dyspnea, orthopnea, productive cough, moist breath sounds | Changes in rate and depth of breathing |
Circulation | Hypertension, jugular pulse visible at 45-degree sitting angle, atrial dysrhythmias | Pulse rate irregularities, dysrhythmia, postural hypotension, sinus tachycardia |
Abdomen | Increased girth, fluid wave | Abdominal cramps |
Modified from Phipps WJ, Long BC, Woods NF, editors: Medical-surgical nursing: Concepts and clinical practice, ed 6, Philadelphia, 1999, Elsevier.
Chest Tube Drainage and Fluid Collection Systems
Chest tubes are large catheters placed in the pleural cavity to evacuate fluid and air and to drain into a graduated collection reservoir at bedside.2 A typical chest tube drainage and collection system is shown in Figure 16-4, A. The removal of thick fluids such as blood and organized exudates by chest tubes is often indicated to prevent entrapment and loculation. Chest tubes are commonly inserted in the sixth intercostal space in the mid- or posterior axillary line. Chest tubes inserted into the pleural space are used to evacuate air or exudate. Chest tubes can also be inserted into the mediastinum to evacuate blood such as after open heart surgery (Figure 16-4, B).
Acid-Base Balance
Control of acid-base balance in the body is achieved by regulation of the hydrogen ion concentration in the body fluids.3,4 The pH of the body is normally maintained within a narrow range of 7.35 to 7.45 or slightly alkaline. When pH of the blood drops below 7.35, a state of acidosis exists; above 7.45, a state of alkalosis exists. Regulation of pH is vital because even slight deviations from the normal range cause marked changes in the rate of cellular chemical reactions. A pH below 6.8 or above 8 is incompatible with life.
Acid-base balance is controlled by several regulatory buffer systems, primarily the carbonic acid-bicarbonate, phosphate, and protein buffer systems. These systems act very quickly to prevent moment-to-moment changes in pH. In compensation, pH is returned to normal by altering the component not primarily affected. If the primary cause is respiratory, the compensating mechanism is metabolic. If the primary cause is metabolic, the compensating mechanism is respiratory. The lungs compensate for metabolic problems over hours, whereas the kidneys compensate for respiratory problems over days (see Chapter 10).
A guide to the clinical presentation of acid-base imbalances is shown in Table 16-2. Along with the major distinguishing characteristics of acid-base imbalance described in this chapter and elsewhere in this volume, potassium excess (hyperkalemia) is associated with both respiratory and metabolic acidosis, and neuromuscular hyperexcitability is associated with both respiratory and metabolic alkalosis.
Table 16-2
Signs and Symptoms of Common Acid-Base Disturbances
Respiratory Disturbances | Metabolic Disturbances | |
Acidosis |
Blood Gases
Analysis of the composition of arterial and mixed venous blood provides vital information about respiratory, cardiac, and metabolic function (see Chapter 10).5,6 For this reason, blood gases are usually analyzed in the ICU. In cases in which the patient’s condition is changing for better or worse over a short period of time or when a specific treatment response is of interest, blood gases may be analyzed several times daily. With an arterial line in place, frequent blood gas analysis is feasible and not traumatic for the patient. Should the patient be anemic, however, blood loss associated with repeated arterial blood sampling may mean that it is contraindicated. Thus requests for arterial blood gas analysis need to be particularly stringent in patients who are anemic.
Arterial saturation (SaO2), the proportion of hemoglobin combined with oxygen, can be readily monitored noninvasively by a pulse oximeter (SpO2). The earlobe or a finger is warmed by rubbing before the oximeter sensor is attached. Within seconds, the SpO2 can be read directly from the monitor. Pulse oximetry is a useful adjunct for routine evaluation of the effectiveness of mechanical ventilation, the effect of anesthesia, and the treatment response. Continuous estimation of SaO2 is particularly useful before, during, and after mobilization and exercise, position changes, and other therapeutic interventions. The SaO2 may appear to be reduced in patients who are anemic or jaundiced or those who have reduced cardiac output. The SpO2 reading may be inaccurate in patients with poor peripheral perfusion, who have cold extremities, or who have pigmented skin. The oxygenation of patients in the ICU varies considerably over time and even moment-to-moment, irrespective of sedation, high post end-expiratory pressure, or inverse ventilation.7
Mixed venous oxygen saturation () provides a useful index of oxygen delivery and utilization at the tissue level.8
is highly correlated to tissue oxygen extraction, and thus is a good index of the adequacy of oxygen delivery. The
is particularly useful as a significant warning sign, a guide to myocardial function, and a tool to titrate positive end-expiratory pressure support. The normal value of
is 75%. Concern is raised by
values of less than 60% or a drop of 10% for several minutes. Excessive
values above 80% are also cause for concern. High
values may occur in patients with left-to-right shunts in the heart, hyperoxia, hypothermia, cyanide toxicity, sepsis, anesthesia, and drug-induced paralysis. Despite its general clinical usefulness,
is a nonspecific indicator of the adequacy of oxygen transport (i.e., of the balance between oxygen supply and demand). Abnormal
values do not indicate precisely where the problem lies; so other hemodynamic variables need to be considered. Further,
has been reported to fluctuate as much as ±6% in patients in the ICU in response to routine activity and treatment.9 Maintaining normal
in patients with multiple trauma improves survival rates over those in whom oxygen transport values are maintained at above normal levels.10 Enhancing cardiac output and hemoglobin as well as
is essential to increase oxygen delivery (DO2) above its critical level and avoid tissue oxygen debt.11 Impaired extraction at the tissue level has been attributed to heterogeneity of oxygen delivery.12
Hypoxemia
In health, age and body position are factors that reduce arterial oxygen tension.13 Arterial oxygen levels diminish with age as a result of reduced alveolar surface area, pulmonary capillary blood volume, and diffusing capacity. Normal PaO2 levels expressed in mm Hg in older people should exceed the value of 110-0.5 (age). In a young adult, PaO2 ranges from 90 to 100 mm Hg in the upright seated position. In the supine position, this range is reduced to 85 to 95 mm Hg; in sleeping, to 70 to 85 mm Hg. These values are clinically significant because in older people, smokers, and people with pathology, these positional effects are accentuated. Despite sedation in patients who are critically ill, spontaneous variability of PaO2 is considerable, and factors unduly influencing it must be controlled when using PaO2 as an outcome.7
Hypoxemia refers to reduced oxygen tension in the blood. Some common signs and symptoms of various degrees of hypoxemia in adults appear in Table 16-3. Although the brain is protected by autoregulatory mechanisms, an arterial oxygen tension of 60 mm Hg produces signs of marked depression of the central nervous system, reflecting the extreme sensitivity of cerebral tissue to hypoxia.
Table 16-3
Signs and Symptoms of Hypoxemia
PaO2 | Signs and Symptoms |
80-100 mm Hg | Normal |
60-80 mm Hg | Moderate tachycardia, possible onset of respiratory distress |
50-60 mm Hg | Malaise |
Lightheadedness | |
Nausea | |
Vertigo | |
Impaired judgment | |
Incoordination | |
Restless | |
Increased minute ventilation | |
35-50 mm Hg | Marked confusion |
Cardiac dysrhythmias | |
Labored respiration | |
25-35 mm Hg | Cardiac arrest |
Decreased renal blood flow | |
Decreased urine output | |
Lactic acidosis | |
Poor oxygenation | |
Lethargy | |
Maximal minute ventilation | |
Loss of consciousness | |
<25 mm Hg | Decreased minute ventilation (secondary to depression of respiratory center) |
Monitoring oxygen kinetics is essential to understanding oxygen transport status in patients who are critically ill and thus ensuring early intervention.12,14 DO2 and and their constituents can be measured directly or indirectly with calorimetry. Patients who survive heart failure have distinct oxygen kinetic profiles.15 In response to improved cardiac index and DO2 in survivors,
did not change, whereas the oxygen extraction ratio (OER) decreased. In nonsurvivors,
increased and the OER did not change. Such profiles can assist in establishing baseline information, detecting changes early, and modifying physical therapy interventions.
ECG Monitoring
A single-channel ECG monitor with an oscilloscope, strip recorder, and digital heart rate display is typically located above the patient at bedside in the ICU (Figure 16-5). Often, the ECG can be observed at bedside and at a central monitoring console, where the ECGs of all patients in the ICU can be observed simultaneously.
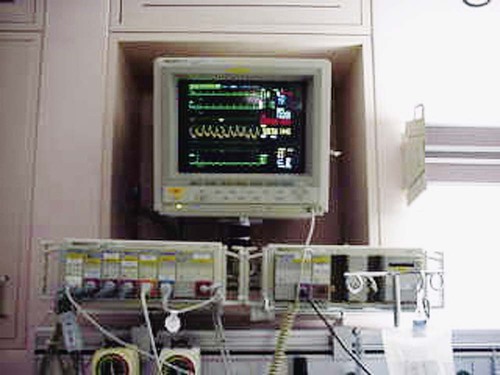
The characteristic features of common dysrhythmias are illustrated in Chapter 12. Physical therapists specializing in ICU management should be thoroughly familiar with ECG interpretation and the implications of the various dysrhythmias on patient management. For further elaboration of ECG application and interpretation, refer to Kinney and Packa16 and Dubin.17
The clinical picture associated with cardiac dysrhythmias depends on the nature of the dysrhythmia, the age and condition of the patient, the medications, and specifically, the absence or presence of underlying heart disease. The distinguishing clinical features of common atrial and ventricular dysrhythmias are outlined in Table 16-4.
Table 16-4
Clinical Picture of Common Dysrhythmias
Dysrhythmia | No Underlying Cardiovascular Disease | Underlying Cardiovascular Disease |
TACHYCARDIAS | ||
A. Supraventricular tachycardia | No symptoms Abrupt-onset palpitations, lightheadedness, nausea, fatigue |
May precipitate congestive heart failure, acute coronary insufficiency, myocardial infarction, pulmonary edema |
1. Sinus tachycardia | Awareness of the heart on exertion or with anxiety | Secondary to some precipitating factor, such as fever, electrolyte imbalance, anemia, blood and fluid loss, infection, persistent hypoxemia in COPD, acute MI, congestive heart failure, thyrotoxicosis |
2. Paroxysmal atrial tachycardia (PAT) | Prevalent, sudden onset, precipitated by coffee, smoking, and exhaustion | Common supraventricular tachycardia Spontaneous onset of regular palpitations that can last for several hours May be obscured by myocardial insufficiency and CHF in older patients Increased anxiety and report of fatigue |
3. Atrial flutter | Rare | Rapid regular-irregular rate |
May be difficult to distinguish from PAT | Suggests block at AV node | |
May be precipitated by alcohol, smoking, physical and emotional strain | Atrial flutter waves in jugular venous pulse | |
4. Atrial fibrillation | Rare, occasionally with alcohol excess in the young | Usually secondary to a variety of cardiac disorders |
5. Paroxysmal atrial tachycardia with block | Rare | Common arrhythmia seen with digitalis toxicity |
B. Subjunctional | Rare | Usually related to MI, pulmonary embolus, severe CHF Often unconscious, cyanotic; ineffective pulse, blood pressure, and respiration |
1. Ventricular tachycardia | Rare | Predisposed to ventricular fibrillation |
2. Ventricular fibrillation | Rare | Ineffective cardiac output, unconscious, dusky; cardiac arrest threat |
BRADYCARDIAS | ||
A. Sinus bradycardia | Physiological in very fit young adults | In older patients, may suggest sinus node and conduction system pathology; can produce syncope or CHF |
B. Heart block | Rare | Hypotension, dizziness, lightheadedness, syncope In chronic block with sustained bradycardia, CHF may be more frequent Most common dysrhythmia iatrogenically produced with digitalis excess Associated with numerous cardiac conditions; commonly in age-related degenerative disease in conducting system, inferior and occasionally anterior MIs |
Hemodynamic Monitoring
Intraarterial Lines
An arterial line is established by direct arterial puncture. It is usually in the radial artery; however, it can be seen in the femoral, axillary, or brachial artery (Figure 16-6, A, B). Blood pressure can be measured directly from this line. A digital monitor displays systolic and diastolic blood pressures above the patient at bedside. High and low blood pressure levels are set, above and below which the alarm will sound. Blood gas analysis can be performed routinely with an intraarterial line in place without repeating the puncture of a blood vessel.
Pulmonary Artery Balloon Flotation Catheter
The pulmonary artery balloon flotation (Swan-Ganz) catheter is designed to provide an accurate and convenient means of hemodynamic assessment in the ICU by monitoring intracardiac pressures and, in combination with other measures, assessing cardiac reserve, an important determinant of outcome following critical illness.18 The catheter is usually inserted into the internal jugular vein, the subclavian vein, or a large peripheral arm vein and is directed by the flow of blood into the right ventricle and pulmonary artery (see Figure 16-3, A). The catheter is carefully secured to prevent dislodging. Some of the complications that have been associated with pulmonary artery catheterization, however, include infection, venous thrombosis, myocardial irritation, air embolism, and pulmonary ischemia or infarct to segmental lung tissue.19
Complex catheters are available for monitoring a variety of parameters. In a two-lumen catheter, the first lumen is used to measure pulmonary artery pressure (PAP) and obtain mixed venous blood samples. The second lumen terminates in a balloon with a volume of less than 1 mL, which is inflated and deflated to obtain pulmonary artery occlusion or wedge pressure (PAOP or PAWP, respectively). The normal range of the systolic PAP is 20 to 30 mm Hg, and it normally reflects right ventricular pressure (RVP). The diastolic PAP ranges from 7 to 12 mm Hg and reflects left ventricular pressure in the absence of pulmonary disease. The average range of PAWP is 8 to 12 mm Hg and gives an estimation of mean left atrial pressure (LAP) and the pressure in the left ventricle (LVP). Figure 16-7 shows the normal cardiac pressures in each heart chamber. More elaborate catheters have pacing wires, thermistors for cardiac output determination, and sensors for arterial saturation.
Despite the enormous benefits of direct invasive hemodynamic monitoring to patient assessment and management, the benefits of basic hemodynamic assessment are a fundamental part of the cardiovascular and pulmonary assessment, regardless of whether the patient has an invasive line inserted.20 Basic hemodynamic monitoring includes heart rate, ECG, blood pressure, and peripheral tissue perfusion. These are fundamental to the physical therapy assessment of all patients across settings. Even though ECG may not necessarily be monitored by the physical therapist directly in non-ICU settings, knowledge of its status is imperative in order to establish whether an individual is safe to treat and, if so, how treatment should be modified and what precautions should be taken.
Central Venous Pressure Monitoring
CVP is monitored by means of a venous line or catheter inserted into the subclavian, basilic, jugular, or femoral vein (see Figure 16-3, C). The catheter is advanced to the right atrium by way of the inferior or superior vena cava, depending on the site of insertion. Minimal risk for phlebitis or infection is associated with this procedure.
Intraaortic Balloon Counterpulsation Device
Intraaortic balloon counterpulsation (Figure 16-8, A) provides mechanical circulatory assistance by using an intraaortic balloon. The balloon is inserted into the femoral artery (see Figure 16-8, B). To maintain proper placement and good circulation, the patient’s leg must be extended. The presence of an intraaortic balloon must be taken into consideration whenever the patient is being treated and positioned. Inflation and deflation of the balloon with helium is correlated with the ECG. The intraaortic balloon is deflated during ventricular systole and assists the emptying of the aorta. Stroke volume is potentiated, afterload is reduced (hence reducing ventricular pressure), and myocardial oxygen delivery enhanced. The balloon is inflated during diastole, thereby restoring arterial pressure and coronary perfusion. Counterpulsation improves cardiac output, reduces evidence of myocardial ischemia, and reduces ST-segment elevation. Intraaortic balloon counterpulsation is commonly used after open-heart surgery and for CHF, medically refractory myocardial ischemia, ventricular septal defects, and left main coronary stenosis in patients who are in shock. The intraaortic balloon pump provides protection for the myocardium in many instances until surgery can be performed. Limb ischemia, the most common complication, occurs in 10% to 15% of patients.
Intracranial Pressure Monitoring
Changes in consciousness are the earliest and most sensitive indicators of increased ICP.21 Altered consciousness reflects herniation of the brainstem and compression of the midbrain. Compression of the oculomotor nerve and the pupilloconstrictor fibers results in abnormal pupillary reactions that are associated with brain damage.
Assessment of Arousal and Brain Activity
Patients in the ICU tend to have low arousal directly or indirectly related to underlying pathology, as well as from induced sedation and antianxiety medications.22 Although medication is often indicated to reduce arousal, a balance must be maintained by the medical staff to avoid under- or oversedating the patient. To benefit maximally from physical therapy and actively participate in treatment, patients need to be as consciously aware as possible. Tools such as the Richmond Agitation-Sedation Scale23 have been developed to quantify arousal state, which can be helpful in directing the course of treatment.
Neuromuscular Assessment
The neuromuscular assessment is a fundamental component of the assessment of the patient in the ICU. The patient may have been admitted for a primary neuromuscular condition with risk for respiratory failure. In addition, all patients in the ICU are at risk for ICU-acquired weakness. ICU-acquired weakness is defined as weakness developing in a patient who is critically ill with no identifiable cause other than nonspecific inflammation.24 It has been associated with the administration of steroids, neuromuscular blockade, hyperglycemia, sepsis, multiple organ failure, bedrest (recumbency), and restricted mobility. The terms critical illness polyneuropathy (CIP) and critical illness myopathy (CIM) are commonly used in the ICU. The neuromuscular manifestations of CIP and CIM are difficult to assess in a valid manner. Despite this, their early identification is essential in order to avoid significant weakness, irreversible deterioration, delayed recovery, and prolonged dependence on mechanical ventilation.25 Other causes need to be ruled out. Prognosis is good if ICU-acquired weakness is detected early and rehabilitation is instituted.
Because many patients in the ICU are administered sedatives and muscle relaxants, placing them at increased risk for ICU-acquired weakness, the physical therapist needs to monitor level of arousal and sedation. The use of agitation and sedation scales can be useful in monitoring arousal and the patient’s capacity to cooperate with treatment.23,26 The physical therapist has an important role in minimizing the need for pharmacological sedation by optimizing pain control, relaxation, comfort, and the individual’s perception of control.
Peripheral-nerve electrical stimulation (e.g., of the median nerve) is used to monitor potential neuropathies in a patient in a coma or under neuromuscular blockade. Such stimulation is sufficient to activate the pathways of the ascending reticular activating system associated with consciousness and to hasten awaking from coma.27 In addition, patients score better on repeated assessment with the Glasgow Coma Scale initially and at 1 month after injury, and they have shorter ICU stays.
There is no specific treatment for ICU-acquired weakness. It is prudent to consider reducing patients’ exposure to the risk factors. Recent studies have demonstrated that the most promising management of ICU-acquired weakness is physical therapy, even while the patient is receiving life-and-organ support interventions.28,29
Cognitive Assessment
The ICU experience can contribute to cognitive impairment or worsen it in patients who are already impaired.30,31 These effects can extend for several months beyond the ICU stay.32 Risk factors include functional dependence and low body mass, as well as previous cognitive impairment.33 Preexisting cognitive impairment must be identified and changes in cognition should be monitored so they can be detected early. Strategies can be implemented to avoid cognitive impairment, including frequent orientation of the patient to time and place, surrounding the individual with familiar objects and family and friends, using familiar tape recordings (voices or music), taking time to talk to the patient, and being reassuring. Further, strategies to reduce anxiety, stress, restlessness, and negative mood states can reduce the duration of hospitalization in patients in the ICU.34
Acute distress disorder comparable to traumatic stress syndrome has been described recently in individuals after accidental injuries. In addition to the nature of the accident itself and the associated threat to life, factors contributing to an individual’s overall stress include hospitalization, length of the ICU stay, the ICU experience, and pain management.35,36 Thus optimizing the patient’s perception of care is important in preventing and offsetting symptoms of acute distress.
With improvement in the management of acute respiratory distress syndrome (ARDS), long-term studies have examined the relationship between cognitive performance, employability, and return to work in survivors. These patients may exhibit long-term cognitive deficits and impaired health status, which contribute to disability and marked reduction in health-related quality of life.37 An understanding of these potential consequences will help their prevention and the comprehensive management of ARDS.
Pain Assessment
Pain has been described as one of the vital signs. Assessing pain in the ICU is particularly challenging, given that patients cannot readily communicate their discomfort and pain. Physical therapists need to monitor discomfort and pain as part of the ongoing assessment to ensure not only that the patient is comfortable but also that therapeutic interventions do not contribute to compromised oxygen transport by increasing distress and that they do not interfere with sleep and recovery. Further, noninvasive interventions should be used as much as possible to reduce the necessity of pharmacological agents that can compromise the patient’s capacity to cooperate with treatment. Optimal management can be achieved only with accurate assessment and ongoing evaluation as the basis for progressing treatment. Pain assessment tools include the McGill Pain Questionnaire, a pain analog scale, the Wong-Baker FACES pain scale, and others.38 The therapist should choose the format to which the patient can most readily respond.
Long-Term Outcomes Related to ICU Care
The study of outcomes of patients who have experienced ICU stays has become a focus of research. In previous decades, if a patient survived the ICU stay, this was viewed as the primary outcome. These days, most patients survive the ICU; however, outcomes related to their return to a full and active life may be less favorable.39 Focusing on long-term outcomes can be integrated into the physical therapy care in the ICU. This includes assessment and evaluation of some aspects of functional status, as well as a premorbid evaluation based on the patient’s self-report, if possible, or that of family members. In addition, the practice of physical therapy needs to extend from the ICU to the ward and then to the home, with judicious follow up after the patient has returned to the community. Depending on the age of the patient, the reason for ICU admission, and comorbidity, return to a maximal level of functioning could take a year or more.