67 Micro- and Nanotechnology and the Aging Spine
Introduction
The aging process presents a cascade of events that affect the health of the musculoskeletal system, in particular, the human spine. The maximum bone mineral density of an individual is reached between the ages of 18 to 20 years of age. As aging progresses, muscle size and strength begin to decrease, by as early as age 25. Accompanying these changes are reductions in hormone levels for both men and women, contributing to a decline in bone density and muscular strength. As we age, the musculoskeletal system experiences degenerative changes resulting in fibrosis, stiffening, and shrinkage of the soft tissue; bone loss; joint changes; and tissue desiccation due to a reduction in proteoglycans and a change in collagen type (i.e., intervertebral disc).10 With respect to the aging spine, this fibrosis and stiffening reduces the osmotic properties of the disc and the ability of the disc to obtain and/or maintain vital nutrients while eliminating noxious wastes. Disc desiccation initiates a cascade of progressive degenerative events leading to loss of disc height, degenerative facets, and compression of the neural structures resulting in pain. The degenerative process continues as the discs of the spine undergo these arthritic bony changes, resulting in altered loading patterns on the spine, further enhancing the patient’s pain and neurological deficits.
Spinal Etiologies12,13,21
Degenerative Disc and Congenital Disorders
The normal aging process results in degenerative disorders due to normal wear and tear of the joints and soft tissues. Although the process of aging results in disc desiccation, facet degeneration, osteophyte formation, and a cascade of mechanical and chemical events that lead to degenerative conditions that cause pain and neurological changes, the ability of the body to heal the tissues still occurs, although at a slower rate with progressive aging.
Arthritis affects approximately 80% of people over the age of 55 in the United States.1,12 It is often triggered by injury, a weakened immune system, and/or hereditary factors. Symptoms include inflammation, joint pain, and progressive deterioration of joint surfaces over time which may result in anatomical changes of the joint surface, and edema inside the joint accompanied by tissue debris. This condition demonstrates mechanical instability in the joint related to the wearing away of the cartilage that is responsible for friction-free motion of the joint. The debris causes an inflammatory response that can induce bone overgrowth and osteophyte formation that eventually interferes with joint mobility. Rheumatoid arthritis is a progressive form of arthritis that can be painfully destructive and may cause the interior joint tissues to swell and thicken, resulting in joint disintegration and eventual significant deformity.
Ankylosing spondylitis is a chronic hereitable disease characterized by progressive inflammation of the spine with early sacroiliac joint involvement, followed by hardening of the anulus fibrosus and surrounding connective tissue and arthritic changes in the facet joints.5; 6 The disease eventually results in a loss of segmental mobility and “stiffening” of the spinal tissues.
Osteoporosis
Osteoporosis is defined as the loss of bone mass and density due to a loss of calcium exchange, which significantly compromises the strength of the vertebral body.1 It is often detected during the later stages of bone loss and will weaken the mechanical integrity of the spinal column. Deformities may develop as the vertebral segments lose a great deal of the cancellous structure, and eventually lead to compression and crush fracture resulting in a kyphotic posture. Loss of bone strength may cause spontaneous fractures to occur, in which the patient’s own body weight alone may cause vertebrae to collapse leading to compressed nerves.
Nanomedicine and the Aging Spine
Drug Delivery Therapies7,19
Over the last few decades, considerable advances have been made toward drug delivery technologies. However, considerable challenges still exist. The continuous release of therapeutic agents over extended time periods following a preprogrammed temporal profile, local delivery of the drug at a constant rate to the diseased microenvironment to overcome systemic toxicity, improved ease of administration, increased patient compliance, minimized risk of side effects, reduced hospital stay, and independent application all pose significant challenges to the effectiveness of the delivered pharmaceutical. Injected or ingested drugs follow first-order kinetics with high blood levels of the drug immediately after initial dosing, followed by an exponential decay in blood concentration. The rapid rise in the drug can lead to toxicity, and the efficacy of the drug is diminished as the drug levels fall exponentially. A continuous drug release profile in a controlled manner for maintaining blood levels is an optimal release design. Currently, most controlled drug delivery systems are transdermal and subcutaneous in nature, with current research, focused on the development of implantable systems that will deliver therapeutic agents in a steady state manner, well underway. The treatment of certain diseases such as osteoporosis and arthritis that require the chronic administration of drugs could benefit from the presence of implantable devices. These devices have the capabilities to provide localized continuous delivery to the physiological site. The benefits of implantable drug delivery systems include the reduction of side effects with improved feedback in a manner that mimics the physiological release profiles of the immune system.
Micro and nanoengineered delivery devices have the potential to improve drug delivery. Numerous materials that were once injected are now capable of being inhaled or swallowed through novel nanodelivery devices, thus improving patient compliance. However, the issues with fluctuating blood levels and drug maintenance still exist. Therefore, a variety of microfabricated devices such as microparticles, microneedles, microchips, nanoporous membranes, and micropumps have been employed in the development of drug delivery systems for the goal of long-term implantation. In essence, this is analogous to the behavior of the immune system. MEMS and NEMS have provided an alternative to current means of drug delivery. The technology allows for systems to be employed that will ease application of the drug and reduce the pain of delivery for injectable drugs. Microneedles of accurate, repeatable micron dimensions have been precisely designed in arrays with reproducible lumen dimensions that pierce tissue allowing for delivery of the drug in a localized manner, yet are small enough to avoid pain and significant tissue damage (Figure 67-1). Human nerves are insensitive to micro- or nanoscale needles, making microneedle arrays for drug delivery ideal for the elderly patient with fragile skin. Implantable nano-channeled needles for drug delivery can provide improvement of control and optimization of pharmocodynamics, by maintaining prolonged steady state of the drug performance and reducing the blood level fluctuation potential and toxicity risks associated with conventional drug delivery. The drug release rates can be accurately modeled and predicted due to the reproducibility of the microneedles.7 Eventually, these systems will be externally controlled by telemetric means where a small drug delivery “chip” is implanted directly to the diseased site and the chemical environment monitored and pharmaceutical agents delivered in response to a change in the chemistry, thus serving both as a diagnostic tool and therapeutic agent for diseased tissue.
Nanopore technology has been implemented in the development of microfabricated nanoporous membranes that are biologically, thermally, chemically, and mechanically stable once implanted into tissue and are also ideal for drug delivery systems and molecular sieves. These membranes are ideal biological sieves that have uniform pore size and very low thickness, making them ideally suited for drug delivery due to the ability of the membranes to have controlled diffusion and sustained release. The pore size, pore length, and pore density can all be highly controlled and serve as ideal diffusion barriers. A drug reservoir can also be fitted to the device for sustained delivery to the tissues.7,19
Micro- and Nanoscale Smart Polymer Technologies
There are numerous types of smart polymers or polymers that exhibit a sharp phase transition from hydrophilic to hydrophobic in response to an environmental stimulus such as pH, temperature, pressure, or strain. Numerous applications exist for these smart polymers or stimuli-responsive polymers, such as drug delivery and drug targeting systems, biodetectors, biosensors, and artificial muscles. Smart polymers are macromolecules capable of undergoing rapid, reversible phase transitions from a hydrophilic to a hydrophobic phase and are thermodynamic systems that undergo a phase transition for a certain range of parameters such as pressure, temperature, and pH.8,15–18,20 Furthermore, there are protein-based polymers that will adhere to surrounding tissue or behave as a barrier to scar tissue, as well as polymers that are electroactive in nature whose properties change drastically upon change of stimulus. Additionally, polymers that respond differently to changing mechanical properties such as strain rates have numerous potential uses in the musculoskeletal system in terms of replacement devices or shock absorbers in the human joints. Aging often results in degenerative joints that have less efficient shock-absorbing behavior, which is further amplified in the spine, where each intervertebral disc serves as a shock absorber to spinal motion. Fast strain rates imposed on these types of polymers would elicit a stiffening response, whereas slow strain rates would elicit a greater elastic zone with greater deformation. Finally, the physical state of a smart polymer coating on the surface of another material can switch from hydrophobic to hydrophilic, where in the hydrophobic state, the surfaces have been found to adhere to proteins and cells and can be patterned after human cells. In the hydrophilic state, these proteins would be released. Such a technology can be incorporated into applications toward engineering human tissue, such as an intervertebral disc or cancellous bone and can provide adhesive or barrier functions to the surrounding tissues.
Nanocoatings
With the advent of spine arthroplasty implants for motion preservation, adherence of the implant to the surrounding bony interface is often challenging for long-term fixation. Biocompatible thermal spray coatings such as titanium plasma sprays and hydroxyapatite coatings (HA) are the conventional means for improving an implant’s fixation to the surrounding bone environment by promoting osseointegration at the interface. The HA coatings are generally 50 to 75 μm thick, and have a mean roughness of 7.5 to 9.5 μm, with a porosity of 1% to 10%, and a bond strength between 20 and 30 MPa. Titanium powders are generally sprayed and exhibit thickness of 350 μm to 600 μm, with a mean roughness of 30 μm and a porosity of 15% to 40%, with a bond strength of 25 MPa.4,14,14 However, the wide range in porosity and thickness can have limiting effects with respect to osseointegration.
Nanocoatings or nanostructured thermal spray coatings exhibit enhanced mechanical performance when compared to the conventional biomedical thermal spray coatings used on orthopedic implants, due to the uniformity and consistency in porous replication. Further characteristics such as higher wear resistance, higher bond strength with the substrate, higher resistance to delamination, higher toughness, and higher plasticity have also been observed with nanostructured coatings.4,9 These structures have been used extensively in the dental arena and tend to have a higher affinity for osteoblast proliferation and uniform bone formation at the interfaces. Improvement of these qualities using nanostructured technologies can lead to improved fixation of implants at the bone interface and the potential to increase the implant’s lifespan within compromised or osteoporotic bone of the aging spine.
Biosensors and Biochips
Chemical markers are macromolecules present in the membranes and fluids in the vicinity of the pathology. The ability to identify degenerative markers could lead to treatment strategies that could diagnose early spinal degeneration and provide treatments at an early stage, when the tissue is capable of healing and reversal of the disease is possible. Microfabricated biosensors can detect and target particular disease-related molecules and proteins. The current methods for protein detection involve labeling procedures that are time-consuming; also, proteins must be in high concentrations for detection, at a point that may not be reversible with respect to tissue healing. Implantable microsized biosensors provide a favorable approach that allows for real-time analysis in minuscule dosages to shorten the detection time and treatment options for the patient. Cantilever-based biosensors for diagnostics have become a promising tool for detection of biomolecular interactions with greater accuracy than conventional methods (Figure 67-2). A nanocantilever and/or microcantilever are devices that can act as physical, chemical, or biological sensors by detecting changes in cantilever bending or vibrational frequency. It is the miniaturized counterpart of a diving board that moves up and down at a regular interval, thus translating molecular or protein recognition into a mechanical motion, at either the nano- or microscale. The mechanical motion can be detected by an optical or piezoresistive readout detector system and, therefore, molecules adsorbed on a microcantilever can cause vibrational frequency changes and deflection of the microcantilever. Biomolecules that are immobilized on the surface of the cantilever beam relay a surface stress to a readout system. If this surface stress is disrupted by a variation in molecular mass or when a specific mass of a molecule is adsorbed on its surface, such as that of a diseased molecule, the readout will reflect the change and the diseased molecule can be detected at a very early time point and at small manageable dosages for successful treatment. Using a cantilever based on the detection of changes in vibrational frequency, the viscosity, density, and flow rate also can be measured and monitored.2
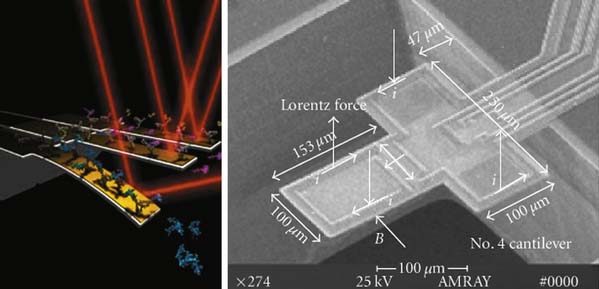
(Left image from http://www.eurekalert.org/features/doe/2001-10/drnl-cm061802.phpen. Right image from Li X, Yu H, Gan X, Xiaoyuan X, Pengcheng X, Jungang L, Liu M, and Yongxiang L: Integrated NEMS/MEMS resonant cantilevers for ultrasensitive biological detection, ed 2 Journal of Sensors (637734): 1-9, 2009.)
Biochips or Lab-on-a-chip (Figure 67-3)3 are microfluidic devices that can conduct several laboratory functions and fluid analyses at rapid speeds designed with microchannels smaller than a single cell, with large surface-area-to-volume ratio where liquids follow a laminar flow pathway. “Lab-on-a-chip” indicates generally the scaling of single or multiple lab processes down to chip-format. Conventional lab work conducted on patients’ blood samples are used to detect disease-related biochemical changes, and often take as long as one to two weeks to obtain diagnostic results. The biochip can conduct a continuous analysis on minuscule amounts of blood within seconds in multiple iterations, and provide more than just a snapshot of information. In doing so, earlier detection of degenerative disease is possible.
The Potential for Micro/Nanotechnology in the Aging Spine
The advancement of micro and nanotechnologies discussed in this section presents novel opportunities for increasing the lifespan and improving the quality of life for the aging population. Micro/nanomedicine has the potential to change the future of medicine and patient care. The areas of concentration for micro/nanomedicine are: (1) therapeutic delivery systems with the potential to deliver gene and pharmaceutical targeting specific cellular pathways, (2) development of novel biomaterials and tissue engineering to guide tissue regeneration, and (3) biosensors and biochips for diagnostic monitoring and therapeutic responses. For the aging patient with the degenerative spine, micro/nanomedicine provide the potential of reversing osteoporosis through nanomanipulation of molecules, gene therapy, localized drug delivery, and the development of nano- and microscaffolds that are osteoinductive and osteoconductive and can regenerate bone tissue. Biosensors and biochips will not only diagnose degenerative disorders of the spine, but provide the possibility of treating degenerative etiologies detected early in the cascade, to avoid the eventual fusion alternative, which has numerous challenges in the compromised spine of the aging individual.
1. http://www.spineuniverse.com/displayarticle.php/article65.html. Accessed February 17, 2010.
2. http://www.azonano.com/details.asp?ArticleID=1927. Accessed February 17, 2010.
3. http://en.wikipedia.org/wiki/Lab-on-a-chip. Accessed February 17, 2010.
4. Bansal P., Padture N.P., Vasiliev A. Improved interfacial mechanical properties of Al2O3-13wt% TiO2 Plasma-sprayed coatings derived from nanocrystalline powders. Acta Materialia. 2003;51:2959.
5. Benzel E., Ferrara L., Roy S., et al. Biomaterials and implantable devices: discoveries in the spine surgery arena. Clin. Neurosurg.. 2002;49:209-225.
6. Benzel E., Ferrara L., Roy S., Fleischman A. Micromachines in spine surgery. Spine. 2004;15(29):6-601.
7. Desai T., Bhatia S. Therapeutic micro/nano technology. Springer; 2006.
8. Galaev I.Y., Mattiasson B. ‘Smart’ polymers and what they could do in biotechnology and medicine. Trends Biotechnol. 1999;17:335-340.
9. Gell M. Development and implementation of plasma sprayed nanostructured ceramic coatings. Surface and Coatings Technology. 2001;48:146-147.
10. Guiot B.H., Fessler R.G. Molecular biology of degenerative disc disease. Neurosurgery. 2000;47:1034-1040.
11. Li X., Yu H., Gan X., Xiaoyuan X., Pengcheng X., Jungang L., Liu M., Yongxiang L: Integrated NEMS/MEMS resonant cantilevers for ultrasensitive biological detection, J. Sensors, 637734, 1-9, 2009.
12. Millenium Research Group: US markets for spinal implants, 2006, 5413, 2006.
13. Niosi C.A., Oxland T.R. Degenerative mechanics of the lumbar spine. Spine J. 2004;4(202S):208S.
14. Oosterbos C.J., Rahmy A.I., Tonino A.J. Hydroxyapatite coated hip prosthesis followed up for 5 years. Int. Orthop. 2001;25:17-21.
15. Peppas N.A., Bures P., Leobandung W., et al. Hydrogels in pharmaceutical formulations. Eur. J. Pharm. Biopharm. 2000;50:27-46.
16. Peppas N.A., Keys K.B., Torres-Lugo M., et al. Poly(ethylene glycol)-containing hydrogels in drug delivery. J. Control. Release. 1999;62:81-87.
17. Peppas N.A., Sahlin J.J. Hydrogels as mucoadhesive and bioadhesive materials. a review, Biomaterials. 1996;17:1553-1561.
18. Peppas N.A., Wood K.M., Blanchette J.O. Hydrogels for oral delivery of therapeutic proteins. Expert Opin. Biol. Ther. 2004;4:881-887.
19. Resiner D.E. Bionanotechnology; global prospects. CRC Press; 2008.
20. Uhrich K.E., Cannizzaro S.M., Langer R.S., et al. Polymeric systems for controlled drug release. Chem. Rev. 1999;99:3181-3198.
21. White A., Panjabi M. Clinical biomechanics of the spine, ed 2. Philadelphia: J.B. Lippincott Company; 1978.