CHAPTER 7 Membrane Structure and Dynamics
Membranes composed of lipids and proteins form the barrier between each cell and its environment. Membranes also partition the cytoplasm of eukaryotes into compartments, including the nucleus and membrane-bounded organelles. Each type of membrane is specialized for its various functions, but all biological membranes have much in common: a planar fluid bilayer of lipid molecules, integral membrane proteins that cross the lipid bilayer, and peripheral membrane proteins on both surfaces.
This chapter opens with a discussion of the lipid bilayer. It then considers examples of integral and peripheral membrane proteins before concluding with a discussion of the dynamics of both lipids and proteins. The following three chapters introduce three large families of membrane proteins: pumps, carriers, and channels. Chapter 11 explains how pumps, carriers, and channels cooperate in a variety of physiological processes. Chapters 24 and 30 cover plasma membrane receptor proteins.
Development of Ideas about Membrane Structure
Our current understanding of membrane structure began with E. Overton’s proposal in 1895 that cellular membranes consist of lipid bilayers (Fig. 7-1A). Biochemical experiments in the 1920s supported the bilayer hypothesis. It was found that the lipids extracted from the plasma membrane of red blood cells spread out in a monolayer on the surface of a tray of water to cover an area sufficient to surround the cell twice. (Actually, offsetting errors—incomplete lipid extraction and an underestimation of the membrane area—led to the correct answer!) X-ray diffraction experiments in the early 1970s established definitely that membrane lipids are arranged in a bilayer.
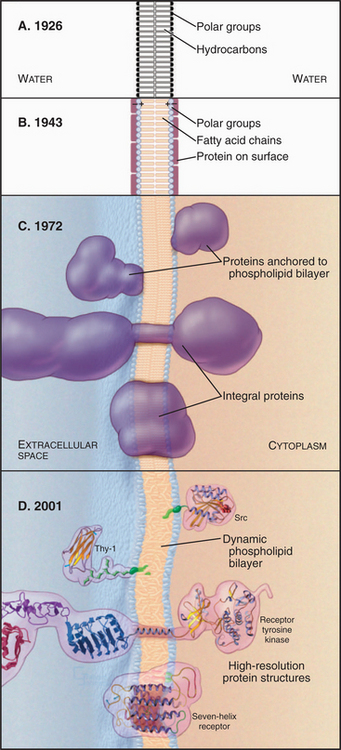
Figure 7-1 development of concepts in membrane structure. A, Gorder and Grendel model from 1926. B, Davson and Danielli model from 1943. C, Singer and Nicholson fluid mosaic model from 1972. D, Contemporary model with peripheral and integral membrane proteins. The lipid bilayer shown here and used throughout the book is based on an atomic model (Fig. 7-5).
During the 1930s, cell physiologists realized that a simple lipid bilayer could not explain the mechanical properties of the plasma membrane, so they postulated a surface coating of proteins to reinforce the bilayer (Fig. 7-1B). Early electron micrographs strengthened this view, since when viewed in cross sections, all membranes appeared as a pair of dark lines (interpreted as surface proteins and carbohydrates) separated by a lucent area (interpreted as the lipid bilayer). By the early 1970s, two complementary approaches showed that proteins cross the lipid bilayer. First, electron micrographs of membranes that are split in two while frozen (a technique called freeze-fracturing; see Fig. 6-4D) revealed protein particles embedded in the lipid bilayer. Later, chemical labeling showed that many membrane proteins traverse the bilayer, exposing different regions of the polypeptide to the aqueous phase on the two sides. Light microscopy with fluorescent tags demonstrated that membrane lipids and some membrane proteins diffuse in the plane of the membrane. Quantitative spectroscopic studies showed that lateral diffusion of lipids is a rapid process but that flipping from one side of a bilayer to the other is a slow one. The fluid mosaic model of membranes (Fig. 7-1C) incorporated this information, showing transmembrane proteins floating in a fluid lipid bilayer. Subsequent work revealed structures of many proteins that span the lipid bilayer, the existence of lipid anchors on some membrane proteins, and a network of cytoplasmic proteins that restricts the motion of many integral membrane proteins (Fig. 7-1D).
Lipids
This chapter concentrates on major lipids found in biological membranes. After an introduction to their structures, the following section explains how the hydrophobic effect drives lipids to self-assemble stable bilayers. Membranes also contain hundreds of minor lipids, some of which might have important biological functions that are not yet appreciated. For example, during the 1980s, a minor class of lipids with phosphorylated inositol head groups first attracted attention when investigators found that they had a major role in signaling (see Fig. 26-7).
Phosphoglycerides
Phosphoglycerides (also called glycerolphospholipids) are the main constituents of membrane bilayers (Fig. 7-2). (These lipids are often called phospholipids, an imprecise term, as other lipids contain phosphate.) Phosphoglycerides have three parts: a three-carbon back-bone of glycerol, two long-chain fatty acids esterified to carbons 1 and 2 (C1 and C2) of the glycerol, and phosphoric acid esterified to C3 of the glycerol. Fatty acids have a carboxyl group at one end of an aliphatic chain of 13 to 19 additional carbons (Table 7-1). More than half of the fatty acids in membranes have one or more double bonds, which create a bend in the aliphatic chain. These bends contribute to the fluidity of the bilayer. Fatty acids and phosphoglycerides are amphiphilic, since they have both hydrophobic (fears water) and hydrophilic (loves water) parts. The aliphatic chains of fatty acids are hydrophobic. The carboxyl groups of fatty acids and the head groups of phosphoglycerides are hydrophilic. The cross-sectional areas of the head groups and the aliphatic tails are similar, so a phosphoglyceride is shaped approximately like a cylinder—an important factor in membrane structure. The hydrophobic effect (see Fig. 4-5) drives amphiphilic phosphoglycerides to assemble bilayers (see later).
Name | Carbons | Double Bonds (Positions) |
---|---|---|
Myristate | 14 | 0 |
Palmitate | 16 | 0 |
Palmitoleate | 16 | 1 (Δ9) |
Stearate | 18 | 0 |
Oleate | 18 | 1 (Δ9) |
Linoleate | 18 | 2 (Δ9, Δ12) |
Linolenate | 18 | 3 (Δ9, Δ12, Δ15) |
Arachidonate | 20 | 4 (Δ5, Δ8, Δ11, Δ14) |
The several head groups confer distinctive properties to the various phosphoglycerides. All have a negative charge on the phosphate esterified to glycerol. Neutral phosphoglycerides—PE and PC—have a positive charge on their nitrogens, giving them a net charge of zero. PS has extra positive and negative charges, giving it a net negative charge like the other acidic phosphoglycerides (PA, PG, and PI). PI can be modified by esterifying one to five phosphates to the hexane ring hydroxyls. These polyphosphoinositides are highly negatively charged.
Several minor membrane phospholipids are variations on this general theme. Plasmalogens have a fatty acid linked to carbon 1 of glycerol by an ether bond rather than an ester bond. They serve as sources of arachidonic acid for signaling reactions (see Fig. 26-9). Cardiolipin has two glycerols esterified to the phosphate of PA.
Sphingolipids
Most sugar-containing lipids of biological membranes are sphingolipids. Sphingolipids get their name from sphingosine, a nitrogen-containing base (Fig. 7-3) that is the structural counterpart of glycerol and one fatty acid of phosphoglycerides. Sphingosine carbons 1 to 3 have polar substituents. A double bond between C4 and C5 begins the hydrocarbon tail. Two variable features distinguish the various sphingolipids: the fatty acid (often lacking double bonds) attached by an amide bond to C2 and the nature of the polar head groups esterified to the hydroxyl on C1.
The head groups of glycosphingolipids consist of one or more sugars. Some are neutral; others are negatively charged. Note the absence of phosphate. Sugar head groups of some glycosphingolipids serve as receptors for viruses. Alternatively, a phosphate ester can link a base to C1. These so-called sphingomyelins have phosphorylcholine or phosphoethanolamine head groups just like PC and PE. Receptor-activated enzymes remove phosphorylcholine from sphingomyelin to produce the second messenger ceramide (see Fig. 26-11). Sphingolipids are much more abundant in the plasma membrane than in membranes inside cells. The hydrocarbon tails of sphingosine and the fatty acid contribute to the hydrophobic bilayer, and polar head groups are on the surface.
Sterols
Sterols are the third major class of membrane lipids. Cholesterol (Fig. 7-4) is the major sterol in animal plasma membranes, with lower concentrations in internal membranes. Plants, lower eukaryotes, and bacteria have other sterols in their membranes. The rigid four-ring structure of cholesterol is apolar, so it inserts into the core of bilayers with the hydroxyl on C3 oriented toward the surface.
Cholesterol is vital to metabolism, being situated at the crossroads of several metabolic pathways, including those that synthesize steroid hormones (such as estrogen, testosterone, and cortisol), vitamin D, and bile salts secreted by the liver. Cholesterol itself is synthesized (see Fig. 20-13) from isopentyl (5-carbon) building blocks that form 10-carbon (geranyl), 15-carbon (farnesyl), and 20-carbon (geranylgeranyl) isoprenoids. As is described later, these isoprenoids are used as hydrocarbon anchors for many important membrane-associated proteins. Isoprenoids are also precursors of natural rubber and of cofactors present in visual pigments.
Glycolipids
Cells have three types of glycolipids: (1) sphingolipids (the predominant form), (2) glycerol glycolipids with sugar chains attached to the hydroxyl on C3 of diglycerides, and (3) glycosylphosphatidylinositols (GPI). Some glycosylphosphatidylinositols simply have a short carbohydrate chain on the hydroxyl of inositol C2. Others use a short sugar chain to link C6 of phosphatidylinositol to the C-terminus of a protein (Fig. 7-9C).
Triglycerides
Triglycerides are simply glycerol with fatty acids esterified to all three carbons. Lacking a polar head group, they are not incorporated into membrane bilayers. Instead, triglycerides form large, oily droplets in the cytoplasm that are a convenient way to store fatty acids as reserves of metabolic energy. In white adipose cells, specialized for lipid storage, the triglyceride droplet occupies most of the cytoplasm (see Fig. 28-6). Mitochondria oxidize fatty acids and convert the energy in their covalent bonds into ATP (see Fig. 19-4).
Physical Structure of the Fluid Membrane Bilayer
In an aqueous environment, amphiphilic lipids spontaneously self-assemble into ordered structures in microseconds. The cylindrical shapes and amphiphilic nature of phosphoglycerides and sphingolipids favor formation of lamellar bilayers, planar structures with fatty acid chains lined up more or less normal to the surface and polar head groups on the surfaces exposed to water (Fig. 7-1D). Bilayer formation is energetically favorable, owing to the increase in entropy when the hydrophobic acyl chains interact with each other and exclude water from the core of the bilayer. This hydrophobic effect increases the entropy of the system and drives the assembly process.
An atomic model of a phosphoglyceride bilayer (Fig. 7-5) has the hydrocarbon chains on the inside and polar head groups facing the surrounding water. The model accounts for the physical properties of biological membranes. It emphasizes the tremendous disorder of the lipid molecules, as expected for a liquid. Polar head groups vary widely in their orientation, and some protrude far into water. This makes the bilayer surface very rough at the nanometer level. The phosphorylcholine head groups are oriented nearly parallel to the bilayer rather than sticking out into water. Fatty acid chains undergo internal motions on a picosecond time scale, making them highly irregular, with about 25% of the bonds in the bent (gauche) configuration. The molecular density is lowest in the middle of the bilayer.
The model also accounts for the mechanical properties of membranes. Although bilayers neither stretch nor compress readily, they are very flexible, owing to rapid fluctuations in the arrangement of the lipids. Thus, one can also draw out a narrow tube of membrane by sucking gently on the surface of a cell. Little force is required to deform bilayers into the complex shapes observed for cell membranes. Both these features are illustrated by the response of a red blood cell plasma membrane to changes in volume (Fig. 7-6). Because the membrane area is constant, a reduction in volume throws the membrane into folds, whereas swelling distends it to a spherical shape until it eventually bursts. If osmotic forces rupture a lipid bilayer, it will reseal.
A variety of biophysical methods, including fluorescence recovery after photobleaching (Fig. 7-11), have shown that lipid molecules diffuse rapidly in the plane of a bilayer. A typical lateral diffusion coefficient (D) for a membrane lipid is approximately 1 mm2 s−1. Given that the rate of diffusion is 2(Dt) 1/2 (t = time), a lipid molecule moves laterally about 1 mm/s in the plane of the membrane. Thus, a diffusing lipid circumnavigates the membrane of a bacterium in a few seconds. Cholesterol flips between the two side of a bilayer on a second time scale. Rarely (about 10−5 s−1), a neutral phosphoglyceride, such as PC, flips unassisted from one side of a bilayer to the other. Charged phosphoglycerides are slower. Proteins can facilitate this flipping in cellular membranes (see Fig. 20-12).
Despite all the lateral movement of the molecules, phospholipid bilayers are stable and impermeable to polar or charged compounds, even those as small as Na+ or Cl−. This poor electrical conductivity is essential for many biological processes (see Fig. 11-6). Small, uncharged molecules, such as water and glycerol, pass slowly across lipid bilayers and more rapidly through channels (see Figs. 10-14 and 10-15).
Biological membranes vary considerably in their lipid composition. In addition to phosphoglycerides, plasma membranes are about 35% cholesterol and over 10% sphingolipids (Fig. 7-7), while internal membranes have little of these lipids. Like bilayers of pure phosphatidylcholine cellular membranes have limited permeability to ions, high electrical resistance, and the ability to self-seal. The length of fatty acids and the presence of unsaturated bonds strongly influence the physical properties of membranes. Fatty acids with 18 or more carbons are solid at physiological temperatures unless they contain double bonds. Hence, phosphoglycerides in biological membranes usually contain C16 saturated fatty acids and longer-chain fatty acids with double bonds (C18 with one to three double bonds and C20 with four double bonds [Table 7-1]). Permanent bends created by double bonds contribute to bilayer fluidity by preventing tight packing of fatty acid tails in the middle of the bilayer. The presence of cholesterol in a bilayer makes the acyl chains pack more compactly. This allows lateral mobility of the lipids but restricts movement of small molecules across the bilayer.
With the exception of cholesterol, most lipids distribute asymmetrically between the two halves of biological membranes. In plasma membranes, glycosphingolipids are outside, while phosphatidylserine and phosphatidylinositol face the cytoplasm (Fig. 7-7). Phosphatidylserine asymmetry gives the cytoplasmic surface of the plasma membrane a net negative charge. Lipid asymmetry established during biosynthesis of membranes (see Chapter 20) is maintained, owing to the low rate of flipping of charged lipids from one side of a bilayer to the other. The lipid composition of prokaryotic membranes differs from that of eukaryotes. Bacterial membranes consist of phosphatidylethanolamine, phosphatidylglycerol, cardiolipin, and other lipids. Archaeal membranes have a mixture of glycolipids, neutral lipids, and ether-linked lipids, and some include single fatty acids.
Since cholesterol interacts favorably with sphingolipids, they have been proposed to form a separate phase in the outer leaflet of plasma membranes named rafts (Fig. 7-7B). It has been hard to pin down the size of such lipid domains and to determine the composition of the adjacent inner leaflet. A variety of indirect evidence is consistent with this idea, but these lipids might actually be dispersed in the outer leaflet of the plasma membrane (Fig. 7-7A), except for special invaginations called caveolae (see Fig. 22-6). Some transmembrane proteins, GPI-anchored proteins, and fatty acid–anchored proteins (Figs. 7-8 and 7-9) associate with sphingolipids and cholesterol in membrane extracts and in artificial bilayers. Consequently, establishing the degree of segregation of these lipids in membranes will also shed light on many membrane functions including signaling.
Membrane Proteins
Integral Membrane Proteins
Atomic structures of a growing number of integral membrane proteins and primary structures of thousands of others show how proteins associate with lipid bilayers (Fig. 7-8). Many integral membrane proteins have a single peptide segment that fulfills the energetic criteria (Box 7-1) for a membrane-spanning α-helix. Glycophorin from the red blood cell membrane was the first of these proteins to be characterized (Fig. 7-8A). Nuclear magnetic resonance experiments established that the single transmembrane segment of glycophorin is an α-helix. This helix interacts more favorably with lipid acyl chains than with water. By analogy with glycophorin, it is generally accepted that single, 25-residue hydrophobic segments of other transmembrane proteins fold into α-helices. In many cases, independent evidence has confirmed that the single segment crosses the bilayer. For example, proteolytic enzymes might cleave the peptide at the predicted membrane interface. Potential glycosylation sites might be located outside the cell. Chemical or antibody labeling might identify parts of the protein inside or outside the cells.
BOX 7-1 Amino Acid Sequences Identify Candidate Transmembrane Segments
Amino acid sequences of integral membrane proteins frequently provide important clues about segments of the polypeptide that cross the lipid bilayer. Each crossing segment must be long enough to span the bilayer with a minimum of charged or polar groups in contact with the lipid (Fig. 7-8). Polar backbone amide and carbonyl atoms are buried in α-helices or β-sheets to avoid contact with lipid. In many transmembrane segments, aromatic residues project into the lipid near the level where acyl chains are bonded to the lipid head groups (red side chains in Fig. 7-8). A helix of 20 to 25 residues or a β-strand of 10 residues is long enough (3.0 to 3.8 nm) to span a lipid bilayer.
Quantitative analysis of the side chain and backbone hydropathy (aversion to water) of the sequence of an integral membrane protein usually identifies one or more hydrophobic sequences long enough to cross a bilayer (see the legend for Fig. 7-8 for details). The approach works best for helices that are inserted directly in the lipid, like the single transmembrane helix of glycophorin A, which has mostly apolar side chains. If a protein has multiple transmembrane helices, some may escape detection by hydrophobicity analysis, because the helices may group together to surround a hydrophilic channel lined with charged and polar side chains. For example, two of seven transmembrane helices of bacteriorhodopsin contain charged residues facing the interior of the protein, so they are less hydrophobic than the other transmembrane helices. Transmembrane β-strands are more challenging, since only half of the side chains face the membrane lipids. None of the transmembrane strands of porin qualify in terms of hydrophobicity criteria. They are short, and many contain polar residues. Independent biochemical or structural data are required to confirm the identity of transmembrane polypeptides.
Transmembrane segments of integral membrane proteins that cross the bilayer more than once are folded into α-helices or β-strands. Hydrogen bonding of all backbone amides and carbonyls in the secondary structure minimizes the energy required to bury the backbone in the hydrophobic lipid bilayer. For the same reason, most amino acid side chains in contact with fatty acyl chains in the bilayer are hydrophobic. Chapter 21 considers how transmembrane proteins fold during their biosynthesis.
Integral membrane proteins with all α-helical transmembrane segments are the most common. Examples are bacteriorhodopsin (Fig. 7-8B; see also Fig. 24-2), pumps (see Figs. 8-3, 8-5, 8-7, and 8-9), carriers (see Fig. 9-3), channels (see Fig. 10-3), cytochrome oxidase (see Fig. 19-5), and photosynthetic reaction centers (see Fig. 19-9). All of these proteins have polar and charged residues in the plane of the bilayer, generally facing away from the lipid toward the interior of the protein, in contrast to the opposite arrangement in water-soluble proteins.
Many transmembrane proteins consist of multiple subunits that associate in the plane of the bilayer (Fig. 7-8). The transmembrane helix of glycophorin A has a strong tendency to form homodimers in the plane of the membrane. Dimers are favored because complementary surfaces on a pair of helices interact more precisely with each other than with lipids. The positive entropy change associated with dissociation of lipids from interacting protein surfaces (comparable to the hydrophobic effect in water) drives the reaction. Unconventional hydrogen bonds between backbone carbonyl oxygens and C-a hydrogens also stabilize dimers. Bacteriorhodopsin molecules self-associate in the plane of the membrane to form extended two-dimensional crystals. Many membrane channels form by association of four similar or identical subunits with a pore at their central interface (see Fig. 10-1). Acetylcholine receptors are pentamers of identical or related subunits. Together, they form a cation channel that opens transiently when the neurotransmitter acetylcholine binds to the two α-subunits (see Fig. 10-12). Bacterial cytochrome oxidase is an assembly of four different subunits with a total of 22 transmembrane helices (see Fig. 19-5). The purple bacterium photosynthetic reaction center consists of three unique helical subunits plus a peripheral cytochrome protein (see Fig. 19-9).
A minority of integral membrane proteins use β-strands to cross the lipid bilayer. Porins form channels for many substances, up to the size of proteins, to cross the outer membranes of gram-positive bacteria and their eukaryotic descendents, mitochondria and chloroplasts. Porins consist of an extended β-strand barrel with a hydrophobic exterior surrounding an aqueous pore (Fig. 7-8C). These subunits associate as trimers in the lipid bilayer.
In addition to transmembrane helices or strands, many integral membrane proteins have structural elements that pass partway across the bilayer. Porins have extended polypeptide loops inside the β-barrel. Many channel proteins have a short helices and loops that reverse in the middle of the membrane bilayer. These structural elements help to form pores specific for potassium (see Fig. 10-3), chloride (see Fig. 10-13), and water (see Fig. 10-15).
Peripheral Membrane Proteins
Six strategies bind peripheral proteins to the surfaces of membranes (Fig. 7-9). One of three different types of acyl chains can anchor a protein to a membrane by inserting into the lipid bilayer. Other proteins bind electrostatically to membrane lipids, and some insert partially into the lipid bilayer. Many peripheral proteins bind directly or indirectly to integral membrane proteins.
Isoprenoid Tails
A 15-carbon isoprenoid (farnesyl) tail (see Fig. 20-13) is added posttranslationally to the side chain of a cysteine residue near the C-terminus of the guanosine triphosphatase (GTPase) Ras (see Fig. 4-6) and many other proteins. The enzyme making this modification recognizes the target cysteine followed by two aliphatic amino acids plus any other amino acid (a CAAX recognition site). Membrane attachment by this farnesyl chain is required for Ras to participate in growth factor signaling (see Fig. 27-6).
Myristoyl Tails
Myristate, a 14-carbon saturated fatty acid, anchors the tyrosine kinase Src (see Box 27-1) and other proteins involved in cellular signaling to the cytoplasmic face of the plasma membrane. Myristate is added to the amino group of an N-terminal glycine during the biosynthesis of these proteins. Insertion of this single fatty acyl chain into a lipid bilayer is so weak (Kd: ˜10−4 M) that additional electrostatic interactions between basic side chains of the protein and head groups of acidic phosphoglycerides are required to maintain attachment to the membrane. As a consequence, phosphorylation can dissociate some myristoylated proteins from membranes by competing with these secondary electrostatic interactions.
Glycosylphosphatidylinositol Tails
A short oligosaccharide-phosphoglyceride tail links a variety of proteins to the outer surface of the plasma membrane. The C-terminus of these proteins is attached covalently to the oligosaccharide, and the two fatty acyl chains of phosphatidylinositol anchor the link to the lipid bilayer. In animal cells, this glycosylphosphatidylinositol (GPI) anchors important plasma membrane proteins, including enzymes (acetylcholine esterase; see Fig. 11-8), adhesion proteins (T-cadherin; see Fig. 30-5), and cell surface antigens (Thy-1). The protozoan parasite Trypanosoma brucei covers itself with a high concentration of a GPI-anchored protein. If challenged by an antibody response from the host, the parasite sheds the protein by hydrolysis of the lipid anchor and expresses a variant protein to evade the immune system.
Electrostatic Interaction with Phospholipids
As was postulated in the 1930s (Fig. 7-1), a number of soluble cytoplasmic proteins bind the head groups of membrane lipids. The full range of these electrostatic interactions has yet to be explored, as the concept was largely neglected for two decades after the recognition of transmembrane proteins and the emergence of the fluid mosaic model of membranes. Annexins, a family of calcium-binding proteins implicated in membrane fusion reactions, bind tightly to phosphatidylserine. Myosin-I motor proteins (see Fig. 36-7) also bind strongly to acidic phosphoglycerides, a possible step in targeting to cellular membranes.
Partial Penetration of the Lipid Bilayer
For years, it was believed that no proteins penetrate the lipid bilayer only partially. It was thought that they either traverse the membrane fully one or more times or bind to the surface. However, some peptide venoms (such as bee venom mellitin) intercalate into half of a lipid bilayer. Hydrophobic α-helices of prostaglandin H2 synthase (see Fig. 26-9) are also postulated to anchor the enzyme to membranes by partially penetrating the lipid bilayer.
Association with Integral Proteins
Many peripheral proteins bind cytoplasmic domains of integral membrane proteins. For example, catenins bind transmembrane cell adhesion proteins called cadherins. These protein–protein interactions may provide more specificity and higher affinity than do the interactions of peripheral proteins with membrane lipids. Such protein–protein interactions anchor the cytoskeleton to transmembrane adhesion proteins (see Fig. 31-7) and guide the assembly of coated vesicles during endocytosis (see Fig. 22-11). Protein–protein interactions also provide a way to transmit information across a membrane. Ligand binding to the extracellular domain of a transmembrane receptor can change the conformation of its cytoplasmic domain, promoting interactions with cytoplasmic, signal-transducing proteins (see Chapter 24 and Fig. 46-17).
The membrane skeleton on the cytoplasmic surface of the plasma membrane of human red blood cells (Fig. 7-10) provided the first insights regarding interaction of peripheral and integral membrane proteins. Two types of integral membrane proteins—an anion carrier called Band 3 and glycophorin—anchor a two-dimensional network of fibrous proteins to the membrane. The main component of this network is a long, flexible, tetrameric, actin-binding protein called spectrin (after its discovery in lysed red blood cells, “ghosts”; see Fig. 33-16). A linker protein called ankyrin binds tightly to both Band 3 and spectrin. About 35,000 nodes consisting of a short actin filament and associated proteins interconnect the elastic spectrin network. This membrane skeleton reinforces the bilayer, allowing a cell to recover its shape elastically after it is distorted by passage through the narrow lumen of blood capillaries.
Heterogeneous, Dynamic Behavior of Membrane Proteins
Several complementary methods can monitor the dynamic behavior of plasma membrane proteins (Fig. 7-11A). One approach—the one used originally—is to label proteins with a fluorescent dye, either by covalent modification or by attachment of an antibody with a bound fluorescent dye. After a spot of intense light irreversibly bleaches the fluorescent dyes in a small area of the membrane, one observes the fluorescence over time with a microscope. If the test protein is mobile, unbleached proteins from surrounding areas move into the bleached area. The rate and extent of fluorescence recovery after photobleaching (FRAP) revealed that a fraction of the population of most membrane proteins diffuses freely in two dimensions in the plane of the membrane but that a substantial fraction is immobilized, since the recovery from photobleaching is incomplete. The same photobleaching method is used to study the mobility of fluorescent fusion proteins targeted to any cellular membrane (see Fig. 6-3). The second approach is to label individual membrane proteins with antibodies or lectins (carbohydrate-binding proteins) attached to small particles of gold or plastic beads (Fig. 7-11B). High-contrast light microscopy can follow the motion of a particle attached to a membrane protein. Despite their size, the particles have minimal effects on diffusion of membrane proteins. The third method is an extension of single-particle tracking. Instead of merely watching spontaneous movements, the investigator can grab a particle in an optical trap created by focusing an infrared laser beam through the microscope objective (Fig. 7-11C). Manipulation of particles with an optical trap reveals what happens when force is applied to a membrane protein.
Membrane proteins exhibit a wide range of dynamic behaviors (Fig. 7-12). Some molecules diffuse freely. Others diffuse intermittently, alternating with periods of restricted movement. A substantial number of membrane proteins are immobilized, presumably by direct or indirect associations with the membrane skeleton or cytoskeleton. Others exhibit long-distance directed movements, presumably powered by motor proteins in the cytoplasm.
The population of a given type of membrane protein (e.g., a cell adhesion protein) may exhibit more than one class of dynamic behavior. For example, most proteins with GPI anchors diffuse freely, as is expected from their association with the lipid bilayer, but a fraction of any GPI-anchored protein has restricted mobility. Some transmembrane proteins also diffuse freely, but a fraction may become trapped or immobilized at any time. Diffusing proteins must be free of interactions with the membrane skeleton and with anchored membrane proteins. Cell adhesion proteins (cadherins; see Fig. 30-5) and nutrient receptors (transferrin receptors; see Fig. 22-14) are examples of transmembrane proteins that diffuse intermittently. They alternate between free diffusion and temporary trapping for 3 to 30 seconds in local domains measuring less than 0.5 mm in diameter. In some cases, trapping depends on the cytoplasmic tails of transmembrane proteins, which are thought to interact reversibly with the cytoskeleton or with immobilized membrane proteins. Tugs with an optical trap show that the cages that confine these particles are elastic, as expected for cytoskeletal networks. Extracellular domains of these proteins may also interact with adjacent immobilized proteins. Immobilized proteins do not diffuse freely, and particles attached to them resist displacement by optical traps. Remarkably, the lipid bilayer can flow past immobilized transmembrane elements without disrupting the membrane. If the plasma membrane of a red blood cell is sucked into a narrow pipette (Fig. 7-6), lipids of the fluid membrane bilayer extend uniformly over the protrusion, leaving behind the immobilized membrane proteins and the membrane skeleton.
Some membrane proteins undergo long-distance translational movements in relatively straight lines. Diffusion cannot account for these linear movements, so they must be powered by motor proteins attached to cytoplasmic domains. Because disruption of cytoplasmic actin filaments by drugs impedes these movements, myosins (see Fig. 36-7) are the most likely, but still unproved, motors for these movements. In some instances, members of the integrin family of adhesion proteins (see Fig. 30-9) use this transport system.
Movement of membrane proteins in the plane of the membrane is essential for many cellular functions. During receptor-mediated endocytosis, receptors are concentrated in coated pits before internalization (see Fig. 22-11). Similarly, transduction of many signals from outside the cell depends on the formation of receptor dimers or trimers (see Figs. 24-5, 24-7, 24-8, 24-9, 24-10, 24-11, and 46-17). Some freely diffusing receptor subunits may be brought together by binding extracellular ligands. In other cases, ligand binding changes the conformation of preexisting dimers in the membrane. In both cases, juxtaposition of the cytoplasmic domains of receptor subunits activates downstream signaling mechanisms, such as protein kinases. Similarly, clustering of adhesion receptors, allowed by movements in the plane of the plasma membrane, enhances binding of cells to their neighbors or to the extracellular matrix (see Figs. 30-6 and 30-11).
ACKNOWLEDGMENTS
Thanks go to Michael Edidin and Donald Engelman for their suggestions on this chapter.
Bijlmakers M-J, Marsh M. The on-off story of protein palmitoylation. Trends Cell Biol. 2003;13:32-42.
Casey PJ, Seabra MC. Protein prenyltransferases. J Biol Chem. 1996;271:5289-5292.
Curran AR, Engelman DM. Sequence motifs, polar interactions and conformational changes in helical membrane proteins. Curr Opin Struct Biol. 2003;13:412-417.
Dowhan W. Molecular basis for membrane phospholipid diversity: Why are there so many lipids? Annu Rev Biochem. 1997;66:199-232.
Edidin M. The state of lipid rafts: From model membranes to cells. Annu Rev Biophys Biomol Struct. 2003;32:257-283.
Edwards PA, Ericsson J. Sterols and isoprenoids: Signaling molecules derived from the cholesterol biosynthesis pathway. Annu Rev Biochem. 1999;68:157-186.
Engelman DM. Lipid bilayer structure in the membrane of Mycoplasma laidlawii. [Bilayer structure established by x-ray diffraction.] J Mol Biol. 1971;58:153-165.
Gahmberg GG, Tolvanen M. Why mammalian surface proteins are glycoproteins. Trends Biochem Sci. 1996;21:308-311.
Jakobsson E. Computer simulation studies of biological membranes: Progress, promise and pitfalls. Trends Biochem Sci. 1997;22:339-344.
Jayasinghe S, Hristova K, White SH. Energetics, stability, and prediction of transmembrane helices. J Mol Biol. 2001;312:927-934.
McNeil PL, Steinhardt RA. Plasma membrane disruption: Repair, prevention, adaptation. Annu Rev Cell Devel Biol. 2003;19:697-731.
Munro S. Lipid rafts: Elusive or illusive? Cell. 2003;115:377-388.
Robertson JD. Membrane structure. [Historical perspective.] J Cell Biol. 1981;91:1895-2045.
Sachs JN, Engelman DM. Introduction to the membrane protein reviews: The interplay of structure, dynamics, and environment in membrane protein function. Annu Rev Biophys Biomol Struct. 2006;35:707-712.
Senes A, Engel DE, DeGrado WF. Folding of helical membrane proteins: The role of polar, GxxxG-like and proline motifs. Curr Opin Struct Biol. 2004;14:465-479.
Simons K, Vaz WLC. Model systems, lipid rafts and cell membranes. Annu Rev Biophys Biomol Struct. 2004;33:269-295.
Stoeckenius W, Engelman DM. Current models for the structure of biological membranes. [Historical perspective.] J Cell Biol. 1969;42:613-646.
Torres J, Stevens TJ, Samsó M. Membrane proteins: The “Wild West” of structural biology. Trends Biochem Sci. 2003;28:137-144.
White SH. The progress of membrane protein structure determination. Protein Sci. 2004;13:1948-1949.
White SH, Wimley WC. Membrane protein folding and stability: Physical principles. Annu Rev Biophys Biomol Struct. 1999;28:319-365.
Zhang FL, Casey PJ. Protein prenylation: Molecular mechanisms and functional consequences. Annu Rev Biochem. 1996;65:241-270.