CHAPTER 10 Membrane Channels
C hannels are integral membrane proteins with transmembrane pores that allow particular ions or small molecules to cross a lipid bilayer. Some channels are open constitutively, but most open just part time. Each time a channel opens, thousands to millions of ions diffuse down their electrochemical gradient across the membrane. Carriers and pumps are orders of magnitude slower, since they use rate-limiting conformational changes to transport each ion (see Chapters 8 and 9).
The ability to control diffusion across membranes allows channels to perform three essential functions (Fig. 10-1). First, certain channels cooperate with pumps and carriers to transport water and ions across cell membranes. This is required to regulate cellular volume and for secretion and absorption of fluid, as in salivary glands, kidney, inner ear, and plant guard cells. Second, ion channels regulate the electrical potential across membranes. The sign and magnitude of the membrane potential depend on ion gradients created by pumps and carriers and the relative permeabilities of various channels (Appendix 10-2). Open channels allow unpaired ions to diffuse down concentration gradients across a membrane, separating electrical charges and producing a membrane potential. Coordinated opening and closing of channels change the membrane potential and produce an electrical signal that spreads rapidly over the surface of a cell. Nerve and muscle cells use these action potentials (see Fig. 11-6) for high-speed communication. Third, other channels admit Ca2+ from outside the cell or from the endoplasmic reticulum into the cytoplasm, where it triggers a variety of processes (see Fig. 26-12), including secretion (see Fig. 21-19) and muscle contraction (see Fig. 39-16).
Channels are important in medicine. Ion channels are targets of powerful drugs and toxins, including curare, tetrodotoxin (“voodoo toxin”), paralytic shellfish toxins, cobra toxin, local anesthetics, antiarrhythmic agents, and probably general anesthetics (Table 10-1). Defects in ion channel genes cause many inherited disorders, including some cardiac arrhythmias and kidney stones. In the human autoimmune disorder myasthenia gravis antibodies target ion channels.
Table 10-1 EXAMPLES OF CHANNEL-BLOCKING AGENTS
Compound (Chemical Class) | Source | Physiological Effect |
---|---|---|
Sodium Channel Blockers | ||
Tetrodotoxin (alkaloid) | Japanese puffer fish | Paralyzes skeletal muscle |
Saxitoxin (alkaloid) | Dinoflagellates | Paralyzes skeletal muscle |
μ-Conotoxins (peptide) | Maine snails | Paralyzes skeletal muscle |
Batrachotoxin (alkaloid) | Arrow poison frogs | Opens Na-channels, paralyzes skeletal muscle |
Lidocaine | Chemical synthesis | Reduces cardiac and nerve excitability |
Potassium Channel Blockers | ||
Quaternary amino alkanes | Chemical synthesis | Blocks K-currents, increases nerve excitability |
Scorpion toxin | Scorpions | Blocks K-currents, increases nerve excitability |
Calcium Channel Blockers | ||
Dihydropyridines | Chemical synthesis | Reduces excitability of L-type channels of striated muscles |
ω-conotoxin (peptide) | Pacific cone snail | Inhibits nervous system N-type channels; blocks synaptic transmission |
Nicotinic Acetylcholine Receptor | ||
α-Bungarotoxin (peptide) | Snake, Bungaris multicinctus | Blocks neuromuscular transmission; paralyzes skeletal muscle |
α-Cobra toxin | Cobra | Blocks neuromuscular transmission; paralyzes skeletal muscle |
Curare | Plant, strychnos toxifera | Blocks neuromuscular transmission; paralyzes skeletal muscle |
This chapter covers 12 large families of plasma membrane channels. Other chapters discuss cystic fibrosis transmembrane regulator Cl− channels (see Fig. 11-4), gap junction channels used for communication between adjacent cells (see Fig. 31-6), and intracellular Ca2+ release channels that participate in signal transduction (see Figs. 26-13 and 39-15). Understanding channels requires not only information about their structure and activity but also some knowledge of electrical phenomena. Appendixes 10-1 to 10-3 contain essential material about electrophysiology.
A new channel can be characterized by expressing its cDNA in a test cell and then making electrical recordings of ion currents from the cell or patches of its membrane (Appendix 10-1). If expression of a single-channel protein fails to reproduce the channel activity observed in the cell of origin, auxiliary subunits are probably required. Historically, investigation of channel functions has relied on toxins and drugs that inhibit particular channels more or less specifically (Table 10-1). This approach is often limited by a lack of specificity. Mutations, including those in human disease, provide definitive tests for physiological functions and have yielded some surprising results.
Channel Diversity and Evolution
Humans have about 400 genes that encode channel proteins. The historical channel nomenclature based variously on the ion transported, mode of regulation, physiological role, or drug sensitivity is often ambiguous. Fortunately, knowledge of channel protein structures clarified evolutionary relationships and provided a framework to classify most plasma membrane channels into a few large families (Fig. 10-2).
Channels are integral membrane proteins, usually with two or more a-helices crossing the lipid bilayer. Porins are an exception; they are built from transmembrane β-strands (see Fig. 7-8C). Channels generally consist of two to six subunits, but some are single, large polypeptides. The transmembrane pores for conducting ions or other substrates are often located in the middle of a group of subunits or subunit-like domains, but the pores of chloride, water, and ammonia channels are located within single subunits.
Channel Structure
The K+ channel KcsA from the Bacterium Streptomyces lividans serves the model for channels in general and the whole family of S5/S6 channels in particular (Fig. 10-3). Four identical subunits are composed of two transmembrane helices connected by a P loop (for pore)—a short third helix and a crucial strand that makes the selectivity filter. The transmembrane helices are packed close together on the cytoplasmic side of the bilayer but splay apart on the extracellular side to make room for the pore helices and selectivity filter.
The selectivity filter accommodates two K+ ions, a local concentration exceeding that inside or outside the cell by more than 10-fold, so it actually concentrates K+. However, it does not impede diffusion through the pore, since electrostatic repulsion between these closely spaced ions forces them apart. Outside the filter, the pore is lined with hydrophobic groups, but a cavity in the middle of this passage accommodates a hydrated K+ in an environment with a negative electrostatic potential that is thought to reduce the electrostatic barrier to the ion as it crosses the membrane, as predicted by earlier physiological studies.
Channel Activity
Single-channel electrical recordings show that channel pores are either open or closed (Figs. 10-4 and 10-5). Open channels, also called the active state, pass selected ions across the membrane at rates approaching their diffusion in water. Closed channels have a different conformation that does not pass ions, small solutes, or water. Many channels also have an inactivated state in which part of the channel protein or an impermeant ion blocks the pore of an otherwise open channel, preventing diffusion of ions through the pore. Inactivation makes a channel unresponsive to conditions that favor the active state. Voltage-gated Na+ channels provide a good example; they cycle from closed to open and then inactivate before returning to the closed state.
Selectivity in the Open State
Open channels vary widely in their ability to discriminate among ions. Highly selective channels, such as voltage-gated K+ channels, pass ions without bound water. Less selective channels, such as the nicotinic acetylcholine receptor, are equally permeable to Na+ and K+, which probably pass through as hydrated ions. Gap junction channels pass most molecules smaller than 800 D without discrimination (see Fig. 31-4).
Extensive physiological data and the structure of KcsA suggest that channels achieve their selectivity by virtue of the fact that particular dehydrated ions bind the channel filter as well as their water shell does (Fig. 10-3). Ions that fit poorly in the pore are rejected, as it is energetically unfavorable to shed their hydration shell. The ion flux through an open channel (at a fixed membrane potential) is approximately proportionate to the ion concentration on the side from which the ions migrate. The maximum rate of ion flux—106 to 108 ions/second—is limited by the time required for binding and dissociation at specific sites as an ion traverses the pore. At this high rate, channels discriminate between selected ions that bind and rejected ions that do not during an interaction lasting only 10 to 100 nano-seconds! Ions may move in single file through the pore, driven in part by electrostatic repulsion between the ions.
Transition between the Closed, Open, and Inactivated States
Switching between conducting and nonconducting states is called gating. Gating determines channel activity because channels generally do not open partway or change their ion selectivity. Transitions between closed and open states are so fast that channels are effectively either fully open or fully closed (Fig. 10-4). The steady-state probability of being open (Po) is simply the fraction of the total time that the channel is open. For a given channel, the fraction of time in the open state determines the ion flux. Because channels act independently, the total flux across a membrane depends on the number of channels that are open at a given time.
Comparison of two K+ channel structures shows how a conformational change physically opens and closes a gate (Fig. 10-5). In the closed state (the KcsA structure), the helices at the cytoplasmic end of the pore occlude the lumen. The gate is open in the Ca-gated K+ channel by virtue of a bend in these helices produced by force exerted by a regulatory domain. Energy from Ca2+ binding to the regulatory domain is converted into mechanical work to pull open the gate. Other gating mechanisms are likely to use this principle.
Some channels fluctuate spontaneously between open and closed, but in most cases, local physiological conditions, which are considered in detail in the following sections, control gating from moment to moment. External or internal ligands open some channels. The membrane potential opens and closes other channels without affecting the conductance of the open channels. Mechanical force gates some channels. Cells also use the full range of signaling mechanisms (see Chapters 24 to 26) from phosphorylation to second messengers to guanosine-triphosphate (GTP)–binding proteins to influence the probability that particular channels open or close. By modulating the sensitivity of various channels, cells modify the behavior of their membranes and their responses to external conditions. This modulation makes channels in general, and membrane excitability in particular, highly adaptable. Chapter 11 illustrates how channel modulation regulates the heart rate, changes the efficiency of communication between nerve cells, and adapts cells to some stresses.
In some cases, a process called inactivation stops the flux of ions through active channels. The pore of an inactivated channel remains in the open conformation, so it admits ions, but a part of the channel itself or an ion blocks the pore and prevents ions from crossing the membrane (Fig. 10-5). Flexible cytoplasmic domains inactivate voltage-gated channels (see later discussion) by plugging the open pore. Large organic or inorganic ions, such as polyamines and Mg2+, block other open channels simply by binding within and occluding the pore. The membrane potential influences ion blocking because it drives ions into or out of channels. A blocking ion that binds an open channel and dissociates slowly turns off the channel for a long time. Blockers that dissociate on a millisecond time scale cause the current through the channel to flicker on and off multiple times every time the channel opens. Even faster blocking events cannot be resolved but reduce the rate at which ions move through active channels. Local anesthetics such as lidocaine are pharmacological channel blockers. Binding sites for blocking ions can be found on the outside, the inside, or both sides of the membrane, depending on the channel.
Opening a channel for a few milliseconds can change the membrane potential but not the cytoplasmic ion composition, because only a few ions must cross the membrane to produce a large change in membrane potential (Appendixes 10-2 and 10-3). This conserves energy because ion gradients created by energy-requiring pumps are not dissipated. Longer openings of tens of milliseconds can alter the ion composition of the cell. For example, voltage-gated Ca2+ channels remain open long enough to change the intracellular Ca2+ concentration and trigger cellular events (see Fig. 39-15 B). In this way, they convert an electrical signal to a chemical signal.
Channels with One Transmembrane Segment
The simplest known channel is found in the membrane envelope of influenza virus. This M2 channel consists of four small subunits, each with but one transmembrane helix. After an infected cell takes the virus into an endosome (see Chapter 22), the acidic environment opens the channel, allowing protons to enter the virus and to begin to disassemble the protein shell that surrounds the genome. The antiviral drug amantadine blocks these channels. Bacteria secrete peptides (gramicidin, alamethicin, and colicins) that are designed to kill other species by forming highly selective and conductive channels. Only 13 amino acids are required for gramicidin A to form β-helical homodimers that function as K+-selective channels.
Channels with Two Transmembrane Segments
Mechanosensitive Channels
MscL from Mycobacterium tuberculosis (Fig. 10-6) is a simple channel of five subunits. One of the two transmembrane helices forms the wall of the pore. A third C-terminal helix extends the pore 4 nm into the cytoplasm. The central pore is lined with polar residues except for a gate at its narrowest constriction, where an isoleucine and a value reduce the diameter to about 0.2 nm. Tension in the plane of the membrane created by osmotic stress is believed to rearrange these helices and open the channel. Cations pass through the open channels indiscriminately at high rates, since they lack a selectivity filter like that of KcsA. This response avoids osmotic lysis of the cell. Such channels are widespread in prokaryotes and are also found in eukaryotes.
Inward Rectifier Potassium Channels
The Kir family of channels has the same evolutionary origin as KcsA (S5-P-S6 [Fig. 10-2]). Like KcsA (Fig. 10-3), Kir channels consist of two transmembrane helices with a P loop in between. The P loop and helix S6 line the K+-selective pore. In spite of these common features, these channels vary in many respects.
Several channels in this family (Kir2.1, Kir2.3, Kir3 family, and Kir4.1) are inward rectifiers. A rectifier is an electronic component that passes current preferentially in one direction. Inward rectifier K+ channels pass K+ into the cell when the membrane potential is below EK (Appendix 10-2), a membrane potential that is not achieved physiologically. Above the resting potential, these K+ channels pass only a small K+ current out of the cell when they open. The reason is that impermeant cytoplasmic cations, Mg2+, and polyamines (ornithine metabolites having net positive charges of 2+ to 4+) bind to negatively charged residues on the cytoplasmic end of the S6 segment of open channels and block the passage of K+. Despite their low permeability, these channels help to maintain the resting membrane potential in many cells and to repolarize excitable cells during an action potential.
Epithelial Sodium Channels
Epithelial Na+ channels accelerate the rate-limiting step in Na+ transport, an essential process that moves salt and water across epithelia in a number of organs (Fig. 10-1A). Typically, epithelial Na+ channels in the apical plasma membrane provide pores for Na+ to diffuse down its concentration gradient into the cytoplasm, and Na+/K+-ATPases in the basolateral plasma membrane pump Na+ out of the cell into the underlying extracellular space. Water follows Na+ through water channels. Renal collecting tubules use this strategy to resorb salt and water. Lung epithelial cells do the same to clear fluid from air spaces. Mice with knockout mutations in the lung epithelial Na+ channel gene die at birth with fluid in their lungs.
Epithelial Na+ channels consist of multiple α-, β-, and γ-subunits, but their stoichiometry is not known. All have two hydrophobic segments that are thought to be transmembrane helices but no verified P loops. The second putative helix probably lines a pore that is 10 times more permeable to Na+ than to K+. The channel opens and closes randomly for relatively long periods, between 0.5 and 5 seconds, unaffected by the membrane potential or any known natural ligand. The drug amiloride blocks epithelial Na+ channels, so they are called amiloride-sensitive Na+ channels to distinguish them from voltage-gated Na+ channels. The steroid hormone aldosterone, produced in response to salt loss, increases the plasma membrane content of Na+ channels and the rate of Na+ resorption by the kidney.
Channels with Four Transmembrane Helices
K+ channels with four transmembrane segments and two P loops (Fig. 10-2, TWIK) are abundant in animal genomes, with 40 to 50 genes in C. elegans. Two of these subunits form a channel with four domains similar to KcsA. They help to establish the resting potential of the plasma membrane by allowing K+ to leak out of the cell, independent of the membrane potential. These leak channels are activated by volatile anesthetics, leading to hyperpolarization of the membrane and reduced excitability.
Voltage-Gated Cation Channels
Voltage-gated channels have two main functions. First, voltage-gated K+ and Na+ channels produce action potentials in excitable cells (see Fig. 11-6). Depolarization of the membrane opens these channels transiently, driving the membrane potential first toward the Na+ equilibrium potential (Appendix 10-2) and then back toward the K+ equilibrium potential. Second, voltage-gated Ca2+ channels convert electrical signals into chemical signals when they admit Ca2+ to the cytoplasm, where it acts as a second messenger (see Figs. 11-8, 11-9, and 26-12) to stimulate secretion, activate protein kinases, trigger muscle contraction, or influence gene expression.
Voltage-gated channels share a common domain organization (Fig. 10-2). Crystal structures of voltage-gated K+ channels from a thermophilic Archaea and rat brain (Fig. 10-7) confirmed that hydrophobic segments S5 and S6 are transmembrane helices with a P loop just like KcsA. The P loop is the selectivity filter, since transplantation of the P loop from one channel to another can yield a chimeric channel with the ion conductance of the foreign P loop. Hydrophobic segments S1 to S4 form a separate domain lateral to the central pore.
In voltage-gated K+ channels, the domains consisting of S1 to S6 are four separate polypeptides that associate noncovalently as homo-oligomers or hetero-oligomers. Animal voltage-gated Na+ and Ca2+ channels consist of four similar but nonidentical domains (each with S1 to S6) linked in a single polypeptide (Fig. 10-2). Voltage-gated channels have additional specialized domains and/or subunits, but the four main domains carry out the basic functions.
The probability that a voltage-sensitive channel is open depends on the membrane potential (Fig. 10-8). The transition is sharp, likely because all four domains respond cooperatively. A negative internal membrane potential stabilizes the closed state. A voltage sensor couples membrane depolarization to channel opening, physically moving charged residues a small distance across the lipid bilayer. Helix S4 is a key part of the sensor. One side of this helix has a spiral of positively charged lysines or arginines. Spectroscopic measurements suggest that the S4 helix makes a subtle motion such as a rotation in response to membrane depolarization. This movement would bring positive charges on S4 closer to the external side of the membrane, accounting for the charge movement that is detected as a “gating current.” Movement of S4 pulls on the helix connecting S4 and S5 (Fig. 10-7A), producing force to open the gate of the channel.
Inactivation is accomplished by flexible parts of these channels, either a ball and chain at the N-terminus of some K+ channels (Fig. 10-7D) or a loop between the domains of Na+ channels. Inactivation depends on membrane depolarization in the sense that the channel must first open to expose a binding site for the inactivation peptide, which then occludes the pore and blocks conduction. As a result, the channel opens only transiently. Less is known about the transition from the inactivated state to the closed state, but a conformational change must occlude the pore before the ball dissociates from the cytoplasmic side of the pore.
Potassium Channels
Vertebrates and invertebrates use three strategies to produce voltage-gated K+ channels with diverse physiological properties. First, they express many different K+ channel proteins from about 20 genes (in Caenorhabditis elegans), augmented by alternate splicing of messenger RNAs. Metazoons appear to have four subfamilies of voltage-sensitive K+ channels. T1 domains near the N-termini of these subunits (Fig. 10-7A) restrict formation of tetramers to subunits from the same subfamily. Second, some K+ channels are heterotetramers, providing a combinatorial strategy with the potential to produce thousands of different tetramers. Third, soluble β-subunits associate with the cytoplasmic side of some α-subunits (Fig. 10-7A) and modify the behavior of the K+ channel tetramer. One type of voltage-gated K+ channel has a Ca2+ binding site at the C-terminus. Signaling events that raise cytoplasmic Ca2+ make these channels more sensitive to membrane depolarization, reducing the excitability of the membrane.
Shaker K+ channels are voltage-gated and rapidly inactivated by a globular “ball” on a flexible polypeptide “chain” at the N-termini of either α- or β-subunits (Fig. 10-7D). After a channel opens, a ball from any α- or β-subunit may inactivate the channel by binding in the open pore. Amputation of the ball residues eliminates inactivation, but a soluble peptide consisting of residues number 6 to 46 can rescue inactivation by binding open channels in reconstitution experiments.
Mutations in the gene for the cardiac K+ channel, called HERG, cause an autosomal-dominant human disease called long QT syndrome. The QT interval is the time between depolarization and repolarization of the heart muscle on electrocardiograms. HERG codes for a heart K+ channel of the delayed-rectifier type, which is responsible for repolarizing the membrane during action potentials (see Fig. 11-11). Mutant channels open more slowly in response to depolarization of the membrane. Affected patients have a mixture of normal and defective K+ channels, which prolongs the action potential and predisposes to abnormal cardiac rhythms and sudden death. Some HERG mutations also cause deafness.
Na+ Channels
Voltage-gated Na+ channels consist of one large α-subunit of four domains linked in series, each with S1 to S6 helices and a P loop (VG-NaCh [Fig. 10-2]). The 260-kD protein is 25% to 30% carbohydrate. These α-subunits alone form voltage-gated Na+ channels in vertebrate hearts and other organs. In some tissues, one or more small β-subunits help to target α-subunits to their proper places in the cell or modify channel behavior.
Voltage-gated Na+ channels depolarize the plasma membrane during action potentials (see Fig. 11-6), so their distribution effectively defines the excitable regions of nerve cell membranes (see Fig. 11-9A). When activated by membrane depolarization, Na+ channels cycle from closed to open to inactivated in 1 to 2 msec. At the threshold voltage, most Na+ channels open synchronously over a narrow range of membrane potential (Fig. 10-8). Open channels are selectively permeable to Na+ (PNa/PK = 12 to 45). In 1 to 2 msec after opening, the channel inactivates when a short cytoplasmic segment between domains III and IV binds to and blocks the open pore. The channel remains inactivated until the membrane repolarizes. Then the channel rearranges to the closed state without reopening. Inactivation does not depend on membrane potential, but because it rarely occurs unless the channel is open, inactivation appears to be voltage dependent.
Calcium Channels
Ca2+ channels are structurally the most complex voltage-gated ion channels (VG-CaCh [Fig. 10-2]). Heart Ca2+ channels were purified by using their affinity for dihydropyridine drugs, so they are also called dihydropyridine receptors. The a1-subunit has four internally homologous domains with sequence features similar to a Na+ channel. It forms voltage-gated, Ca2+-selective channels. The a2-subunit is a glycoprotein with no homology to other channel subunits. Its role is uncertain, but coexpression of a2 appears to be essential for assembly and normal gating kinetics of a1. The roles of the other, smaller peptide subunits designated β, γ, and δ are less well characterized.
Like voltage-gated Na+ channels, Ca2+ channels are activated by membrane depolarization, inactivated by a first-order process, and returned to the resting state when the membrane repolarizes. Inactivation is generally slower than that for Na+ channels.
Ca2+ channels have numerous functions. First, in some cells, Ca2+ channels contribute to membrane depolarization during action potentials. Given the very low Ca2+ concentration inside cells (see Fig. 26-12), open Ca2+ channels have a powerful effect on membrane potential. During an action potential, Ca2+ currents supplement Na+ currents in vertebrate heart cells and replace Na+ currents in heart pacemaker cells (see Fig. 11-11) and some invertebrate neurons.
Second, given their long activity cycles, Ca2+ channels can convert electrical signals (membrane depolarization) into chemical signals by raising the cytoplasmic Ca2+ concentration. In cardiac muscle, Ca2+ triggers the release of Ca2+ from internal stores to stimulate contraction (see Fig. 39-15). In nerve terminals, an influx of Ca2+ triggers the secretion of neurotransmitters (see Figs. 11-8 and 11-9). In some neurons, changes in postsynaptic Ca2+ levels are associated with changes in the strength of synaptic signals. These changes constitute one level of synaptic learning (see Fig. 11-10).
Third, plasma membrane Ca2+ channels act as voltage sensors in skeletal muscle. Action potentials stimulate Ca2+ channels, which use direct physical contact to activate Ca2+ release channels located in the endoplasmic reticulum (see Fig. 39-15). The released Ca2+ stimulates contraction.
To carry out these diverse physiological functions, vertebrate cells express a variety of Ca2+ channel proteins with different physiological properties. Traditionally, Ca2+ channels have been divided into several classes, termed N, T, L, and P/Q, based on their sites of expression, voltage required for activation, open channel currents, inactivation kinetics, and sensitivity to drugs (Table 10-2). For example, only L-type calcium channels are sensitive to dihydropyridines, which are used therapeutically to dilate blood vessels by relaxing smooth muscle. N-type Ca2+ channels resist dihydropyridines but are blocked selectively and nearly irreversibly by w-conotoxin, which prevents neurotransmitter release at some synapses. Although this classification is still useful, the continued discovery of channels with novel properties has blurred these distinctions. Now cDNA cloning, expression, and characterization of single molecules provide more discrimination.
TRP Channels
No high-resolution structures are available, but the sequences of TRP channels indicate six transmembrane helices and a possible P-loop (Fig. 10-9). These subunits form tetrameric channels that are thought to be similar in architecture to voltage-sensitive K-channels, including a gate on the cytoplasmic side of the ion-conduct-ing pore.
All TRP family members are cation channels, admitting modest amounts of both extracellular Na+ and Ca+2 when active. Diverse stimuli activate the various TRP channels, but the mechanisms are still poorly understood and subject to controversy. In the simplest case, extracellular ligands such as hot spices or cooling chemicals open particular channels. High temperature also activates the hot spice channels, accounting for the perception of such spices as being “hot.” The brain cannot discern whether the TRP channels in a sensory nerve are activated by heat or a spice. Similarly, cold temperatures activate another TRP channel that responds also to cooling chemicals such as menthol. Signaling mechanisms downstream from seven-helix receptors and receptors tyrosine kinases (see Chapter 24) activate other TRP channels, in some cases by producing a second messenger (see Chapter 26) such as the membrane lipids PIP2 and diacylglycerol.
Channels Gated by Intracellular Ligands
Genes for families of channels gated by cytoplasmic Ca2+, cyclic nucleotides, or β/γ-subunits of trimeric γ-proteins (see Fig. 25-9) diverged from K+ channels relatively recently in evolution, about the time when animals diverged from fungi. Their sequences are similar to each other (LC ligand-gated [Fig. 10-2]).
Ca2+-activated K+ channels are first cousins of voltage-gated K+ channels. They have six transmembrane segments and a P loop. The Ca2+-binding protein, cal-modulin (see Fig. 3-12C), binds constitutively to the cytoplasmic tail following S6. Ca2+, entering the cytoplasm through the plasma membrane or released from intracellular stores (see Fig. 26-12), binds this associated calmodulin and activates the channel by making it more sensitive to membrane depolarization. Expression from different genes and alternative splicing produce a variety of these channels with different physiological properties.
Cyclic nucleotide–gated ion channels have six membrane-spanning segments with a P loop and a C-terminal cyclic nucleotide–binding domain homologous with bacterial cyclic nucleotide–binding proteins (Fig. 10-10). Four of these subunits, some of which may be different isoforms, form a functional channel. Binding of cyclic adenosine monophosphate (cAMP) to the cytoplasmic receptor domain opens a pore for Na+ and Ca2+ and depolarizes the membrane. Changes in cyclic nucleotide concentration provide a sharp on/off switch, as ligand must occupy at least three of the four subunits to open the channel. Ca2+ entering the cytoplasm binds to calmodulin associated with the N-terminal cytoplasmic part of the protein. This provides negative feedback to the channel.
Ion channels gated by intracellular cyclic nucleotides are particularly important in sensory systems, including olfaction (see Fig. 27-1) and vision (see Fig. 27-2). Odorant molecules stimulate olfactory sensory neurons by binding seven-helix receptors in the plasma membrane. These receptors work through trimeric γ-proteins to increase the cytoplasmic concentration of cAMP. cAMP opens cAMP-gated cation channels, depolarizes the membrane, and activates voltage-gated Na+ channels to fire an action potential. Visual transduction also uses a cyclic nucleotide–gated channel. Light activates a seven-helix receptor, leading to a decline in cytoplasmic cyclic guanosine monophosphate (cGMP). This closes cGMP-gated channels, hyperpolarizing the photoreceptor plasma membrane and reducing the secretion of neurotransmitter (see the next section).
Ion Channels Gated by Extracellular Ligands
Channels that are gated by chemicals mediate communication between nerve terminals and other nerves or muscles. This communication takes place at specializations called synapses, which facilitate chemical transmission (see Figs. 11-8 and 11-9). On the sending side, presynaptic terminals are specialized for exocytosis of chemicals called neurotransmitters, which they package in small synaptic vesicles. Neurotransmitters include acetylcholine, serotonin, glutamic acid, glycine, and γ-aminobutyric acid (GABA) (see Fig. 11-7). When an action potential arrives at a nerve terminal, voltage-gated Ca2+ channels admit Ca2+ to the cytoplasm, causing synaptic vesicles to fuse with the plasma membrane, releasing transmitter outside the cell. Transmitters diffuse to the postsynaptic membrane in micro-seconds.
Stimulation of ligand-gated channels is transient because of an inactivating conformational change called desensitization and because neurotransmitters are rapidly removed from the synaptic cleft between the cells (see Figs. 11-8 and 11-9). An extracellular enzyme degrades acetylcholine. Carriers (see Chapter 9) remove all other neurotransmitters by pumping them back into the presynaptic cell.
Glutamate Receptors
Glutamate receptors depolarize the postsynaptic membrane when glutamate binding opens a cation channel that is permeable to both Na+ and K+ (see Fig. 11-9). This depolarization of the plasma membrane excites the cell by activating voltage-sensitive sodium channels to trigger an action potential. Eukaryotic glutamate receptor channels (Fig. 10-11) have an extracellular ligand-binding domain and four hydrophobic segments: M2 is a P loop between transmembrane helices M1 and M3. Four subunits form a channel with their P loops on the cytoplasmic side of the plasma membrane rather than outside, like KcsA and its many relatives. A change in the conformation of the extracellular domain induced by glutamate binding opens a pore through the middle of the channel. Successive binding of glutamate to each of the four subunits opens the pore in steps (although binding is usually too fast to resolve these partially open states).
Multiple genes, alternative splicing, and RNA editing (see Fig. 16-7) all provide a diversity of glutamate receptor subunits, which assemble into homomeric and heteromeric channels used in different parts of the nervous system. Three families of isoforms are sensitive to different pharmacologic agonists in addition to glutamate: N-methyl-d-aspartate (NMDA), α-amino-3-hydroxy-5-methyl-4-isoxazole propionate (AMPA), or kainate. NMDA receptors are more permeable to Ca2+ than to Na+ and K+. Because excess intracellular Ca2+ can be damaging, overstimulation of NMDA receptors by glutamate released from cells during strokes or constitutive activation of NMDA receptors by point mutations can kill nerve cells.
Eukaryotic glutamate receptor channels apparently originated in Bacteria by fusion of genes for a periplasmic amino acid–binding protein (similar to Escherichia coli glutamine-binding protein) and an S5/P/S6 potassium channel similar to KcsA. The domain organiza-tion of plant glutamate receptors is similar to that of animal brain glutamate receptors. Glutamate receptors participate in the response of developing plants to light.
Nicotinic Acetylcholine Receptor
The best-characterized ligand-gated channel is an excitatory cation channel—the nicotinic acetylcholine receptor from the plasma membrane of skeletal muscle cells. This receptor triggers action potentials that stimulate muscle contraction (see Figs. 11-8 and 39-14). It is called the nicotinic acetylcholine receptor because it also binds the tobacco alkaloid nicotine. Related nicotinic acetylcholine receptors in the central nervous system are the targets in tobacco addiction.
The muscle nicotinic acetylcholine receptor is a pentamer of four different, but homologous, subunits with the composition a2bge (Fig. 10-12). Each subunit has a large N-terminal extracellular segment, four transmembrane α-helices (M1 to M4), and a large cytoplasmic segment between M3 and M4. M2 α-helices from the five subunits line a central transmembrane pore like staves of a barrel. Hydrophobic side chains line this pore except for a few negative charges that may contribute to cation selectivity. Three other α-helices of each subunit separate the M2 helices from the surrounding lipid. The N-terminal segments of each subunit form massive extracellular domains, each folded into similar, highly twisted β-sandwiches. The α-subunits have deep cavities that bind acetylcholine.
Gating and ion selectivity of acetylcholine receptors differ in concept from the P-loop family of channels. In closed channels, the narrowest part of the closed pore is less than 7 Å in diameter, too small for hydrated K+ and Na+ ions, and the hydrophobic pore does not provide a passage for unhydrated ions. Acetylcholine binding to the two α-subunits changes the conformations of the extracellular domains, which rotate the M2 helices and open a channel that is more permeable to K+ and Na+ than to Ca2+. The resulting permeability to all three ions causes the membrane potential to collapse toward a reversal potential (see the section titled “Net Current through Ion-Selective Channels”) around 0 mV. This triggers voltage-gated Na+ channels to initiate a self-propagating action potential in the muscle plasma membrane with nearly 100% efficiency (see Fig. 11-8).
Many toxins bind nicotinic acetylcholine receptors, blocking transmission of impulses between motor nerves and skeletal muscle (Table 10-1). -Bungarotoxin has been used to characterize the receptor. Curare is a powerful muscle relaxant that is used during surgery because it blocks acetylcholine-binding sites without opening the channel. Local anesthetics, such as procaine, bind within the channel and block ion conductance.
ClC Chloride Channels
ClC subunits are triangular transmembrane proteins formed from 18 α-helices (Fig. 10-13). These helices surround a pore that passes through the middle of each subunit, like the pores of ammonia channels (Fig. 10-14), aquaporins (Fig. 10-15), and porins (see Fig. 7-8C). Several helices around the pore extend only part way across the lipid bilayer. Highly conserved residues in the loops between these helices form the selectivity filter for Cl− in the middle of the protein and the membrane bilayer. Two subunits associate tightly in the lipid bilayer, so each channel has two pores.
The best-known member of the family is ClC0 from skeletal muscle. Like voltage-gated cation channels, ClC0 channels open when the membrane depolarizes and subsequently inactivate. In contrast to cation channels, which have a single conductance state, active Cl− channels conduct at two levels: 10 or 20 pS (picosiemens; see the section titled “Net Current through Ion-Selective Channels”). The pairing of two subunits, each with a pore capable of conducting at 10 pS, explains this behavior. When active, either one or both subunits conduct Cl−. A negatively charged glutamate side chain is believed to block the pore of inactive channels and to swing out of the way in active channels. In an unexpected turn of events, physiological analysis of the bacterial ClC channel used for structural studies revealed many features of a carrier that exchanges Cl− for H+ rather than features of a typical ion channel behavior like other members of this family. This is one of several examples of blurred distinctions among channels, carriers, and pumps.
Ammonia Channels
One ancient channel family evolved in early prokaryotes to conduct ammonia across the cell membrane. Ammonia can directly penetrate lipid bilayers, but these channels allow low concentrations of ammonia to serve as a source of nitrogen that prokaryotes use to synthesize proteins and nucleic acids. Bacteria, Archaea, and eukaryotes still depend on these channels. In humans, these channels conduct both ammonia and carbon dioxide across the plasma membranes of red blood cells, where they are known as Rh antigens (Box 10-1). These channels are also important for ammonia transport in the human kidney and liver.
Ammonia channels consist of three identical subunits, each composed of 11 transmembrane helices and having its own conducting pore (Fig. 10-14). These are the only known trimeric channels (Fig. 10-2). The interfaces between these subunits are tightly sealed, but each subunit has a narrow internal pore that is highly selective for ammonia and methylammonium. Chloride channels (Fig. 10-13) and aquaporins (Fig. 10-15) also have conducting pores through subunits rather than the more common strategy of forming pores at a central interface among subunits. Both substrates (ammonium NH4+ and methylammonium CH3NH3+) are charged in aqueous solution and must leave behind a proton to pass through the pore as uncharged species (NH3, CH3NH2). They pick up a replacement proton on the other side of the membrane as they exit the channel. Selectivity is achieved by the tight fit of the substrates in the hydrophobic pore and by transient formation of an unusual hydrogen bond within the pore. With millimolar ammonium on one side of a membrane, these channels conduct hundreds of ammonia molecules per second without leaking water, protons, or other charged species.
Water Channels
Aquaporins provide highly permeable pores for water to cross membranes. Four identical subunits form a stable tetramer in the plane of the membrane (Fig. 10-15). Each subunit has a narrow pore that is selective for water passing through the middle of a bundle of α-helices. About 10 water molecules line up in a pore about 0.3 nm in diameter. Hydrogen bonding of waters with a pair of asparagine residues at a narrow point in the pore allows the channel to be selective for water. The two halves of the protein arose by a gene duplication, since their sequences are remarkably similar.
Osmotic pressure created by pumps, carriers, and the macromolecular composition of the cytoplasm drives water through aquaporins at rates exceeding 109 molecules per second. This explains why red blood cells rapidly swell and shrink passively, depending on the osmolarity of the surrounding fluid (see Fig. 7-6). Water channels have no gates, so they are open constitutively.
Various human tissues express 12 different aquaporin isoforms. Aquaporin-1 is found in red blood cells, renal proximal tubules, blood vessel endothelial cells, and the choroid plexus (which makes spinal fluid in the brain). A few humans carry mutations that inactivate aquaporin-1; remarkably, homozygotes have no symptoms, despite the low water permeability of their red blood cells (and presumably other tissues that depend on this isoform). Aquaporin-2 is required for renal collecting ducts to reabsorb water. A patient with inactivating mutations in both aquaporin-2 genes suffered from severe water loss, called nephrogenic diabetes insipidus. Antidiuretic hormone (vasopressin) controls the placement of aquaporin-2 in the collecting duct membrane. It activates a seven-helix receptor, causing cytoplasmic vesicles storing aquaporin-2 to fuse with the plasma membrane. This increases the permeability of apical plasma membranes to water, allowing it to move from the urine into the hypertonic extracellular space of the renal medulla. Reaction of sensitive cysteine residues with mercuric chloride closes the water pores of aquaporins. This explains how mercurials, used therapeutically as diuretics in the past, inhibit the reabsorption of water filtered by the kidney. The reversible inhibition of aquaporins with mercurials provides a test for their participation in physiological processes.
Porins
Porins are channels with wide, water-filled pores found in the outer membranes of gram-negative bacteria and mitochondria. The subunits are composed of an antiparallel barrel of 16 or 18 β-strands that cross the membrane (see Fig. 6-8C). One to three of the loops connecting the strands extend into the center of the barrel and line the pore. The functional molecule consists of three identical subunits.
Armstrong CM. Voltage-gated K channels. Sci STKE. 2003;188:re10. Available at http://stke.sciencemag.org/cgi/content/gloss/sigtrans;2003/188/re10.
Bichet D, Haass FA, Jan LY. Merging functional studies with structures of inward-rectifier K(+) channels. Nat Rev Neurosci. 2003;4:957-967.
Birnbaum SG, Varga AW, Yuan LL, et al. Structure and function of Kv4-family transient potassium channels. Physiol Rev. 2004;84:803-833.
Catterall WA, Goldin AL, Waxman SG. International Union of Pharmacology. XXXIX. Compendium of voltage-gated ion channels: Sodium channels. Pharmacol Rev. 2003;55:575-578.
Catterall WA, Striessnig J, Snutch TP, Perez-Reyes E. International Union of Pharmacology. XL. Compendium of voltage-gated ion channels: Calcium channels. Pharmacol Rev. 2003;55:579-581.
Clapham DE. TRP channels as cellular sensors. Nature. 2003;426:517-524.
Dolphin AC. G protein modulation of voltage-gated calcium channels. Pharmacol Rev. 2003;55:607-627.
Dutzler R. The structural basis of ClC chloride channel function. Trends Neurosci. 2004;27:315-320.
Gulbis JM, Doyle DA. Potassium channel structures: Do they conform? Curr Opin Struct Biol. 2004;14:440-446.
Hille B. Ion Channels of Excitable Membranes, 3rd ed. Sunderland, Mass: Sinauer Associates, 2001.
Hulme JT, Scheuer T, Catterall WA. Regulation of cardiac ion channels by signaling complexes: Role of modified leucine zipper motifs. J Mol Cell Cardiol. 2004;37:625-631.
Jiang Y, Lee A, Chen J, et al. Crystal structure and mechanism of a calcium-gated potassium channel. Nature. 2002;417:515-522.
Jiang Y, Lee A, Chen J, et al. X-ray structure of a voltage-dependent K+ channel. Nature. 2003;423:33-41.
Khademi S, O’Connell J, Remis J, et al. Mechanism of ammonia transport by Amt/MEP/Rh: Structure of AmtB at 1.35 Å. Science. 2004;305:1587-1594.
King LS, Kozono D, Agre P. From structure to disease: The evolving tale of aquaporin biology. Nat Rev Mol Cell Biol. 2004;5:687-698.
Li B, Gallin WJ. VKCDB: Voltage-gated potassium channel database. BMC Bioinformatics. 2004;5:3.
Lu Z. Mechanism of rectification in inward-rectifier K+ channels. Annu Rev Physiol. 2004;66:103-129.
Matulef K, Zagotta WN. Cyclic nucleotide-gated ion channels. Annu Rev Cell Devel Biol. 2003;19:23-44.
Miyazawa A, Fujiyoshi Y, Unwin N. Structure and gating mecha-nism of the acetylcholine receptor pore. Nature. 2003;423:949-955.
Noskov SY, Berneche S, Roux B. Control of ion selectivity in potassium channels by electrostatic and dynamic properties of carbonyl ligands. Nature. 2004;431:830-834.
Perozo E, Rees DC. Structure and mechanism in prokaryotic mechanosensitive channels. Curr Opin Struct Biol. 2003;13:432-442.
Roosild TP, Lê K-T, Choe S. Cytoplasmic gatekeepers of K+-channel flux. A structural perspective. Trends Biochem Sci. 2004;29:39-45.
Schild L. The epithelial sodium channel: From molecule to disease. Rev Physiol Biochem Pharmacol. 2004;151:93-107.
Sigworth F. Life’s transitors. Nature. 2003;423:21-22.
Stocker M. Ca(2+)-activated K+ channels. Molecular determinants and function of the SK family. Nat Rev Neurosci. 2004;5:758-770.
Stroud RM, Miercke LJW, O’Connell J, et al. Glycerol facilitator GlpF and the associated aquaporin family of channels. Curr Opin Struct Biol. 2003;13:424-431.
Viswanathan PC, Balser JR. Inherited sodium channelopathies: A continuum of channel dysfunction. Trends Cardiovasc Med. 2004;14:28-35.
Yu FH, Catterall WA. The VGL-chanome: A protein superfamily specialized for electrical signaling and ionic homeostasis. Sci STKE. 2004;253:re15.
APPENDIX 10-1 Electrical Recordings in Biology
Analysis of electrical activity across biological membranes requires sensitive methods to detect electrical potential differences and the flow of current on a rapid time scale. Physiologists and clinicians use four general methods, with different sensitivities, to detect electrical activity of single channels (patch electrodes), cell membranes (microelectrodes and fluorescent dyes), and whole tissues (extracellular electrodes).
Single-Channel Recordings with Patch Electrodes
Patch-clamp microelectrodes (Fig. 10-16A) provide the best way to characterize the behavior of individual channels. A small-diameter, fire-polished glass capillary is pressed onto the surface of a cell and suction is used to form a high-resistance seal (10 to -50 gigaohms). The membrane patch is small enough to contain just a few ion channels. The electrode becomes part of an electric circuit that can measure current or voltage across the membrane. The high-resistance seal between micropipette and membrane ensures that more electrical current (composed of ions) flows through a single open channel than leaks in around the side of the electrode. When a channel opens, a sensitive ammeter connected to the micropipette records the direction and magnitude of ion flow through the channel as an electrical current. Patch electrodes give direct information about both current and the time that individual channels spend open or closed.
Variations of the patch-clamp technique provide access to channel properties. Leaving the membrane patch on the cell (cell-attached configuration) reveals properties of the channels in their cellular context. Lifting the membrane patch off the cell (excised-patch configuration) exposes the cytoplasmic surface of the membrane to ions, enzymes, or second messengers that the investigator adds to the bath. Similarly, the investigator can test the effects of potential ligands, drugs, and ions in the micropipette.
Measurement of Membrane Potentials with Intracellular Microelectrodes and Fluorescent Dyes
A glass capillary is drawn to a fine tip (˜0.5 mm), filled with a conducting solution (3 M KCl), and inserted through the plasma membrane. The tip penetrates the cell with minimal damage, and the membrane seals tightly around it. The microelectrode is connected to a meter to record current and voltage (Fig. 10-16B). Alternatively, the investigator can apply a patch electrode to the cell surface and suck forcefully to breach the membrane, putting the micropipette in continuity with the cytoplasm for recordings from the rest of the membrane.
APPENDIX 10-2 The Biophysical Basis of Membrane Potentials
The membrane potential arises from separation of charges across an insulating surface (Fig. 10-17). The lipid bilayer provides the insulation required to separate charges. Either pumps or channels can produce unpaired charges. Pumps that transport unpaired ions generate membrane potentials directly. Channels that pass un-paired ions can use ion concentration gradients across membranes to generate membrane potentials. The concentration gradient provides a diffusional force to drive ions through channels. Because channels are ion specific, an excess of charge builds up after very few ions cross a membrane. This excess charge creates a membrane potential and stops the net movement of additional ions across the membrane.
Diffusion Potentials
An impermeable membrane enclosing concentrated potassium chloride is suspended in a bath of more dilute potassium chloride (Fig. 10-17). If the mem-brane contains a pore that is selectively permeable for bidirectional diffusion of K+, the concentration gradient drives K+ out of the membrane compartment. Because Cl− cannot pass through this selective pore or the membrane bilayer, the inside compartment loses positive charge. Charge imbalance creates an electrical field, negative inside, called the membrane potential. By convention, extracellular voltage is de-fined as zero.
Quantitative Relationships
A typical cell has a Ki:Ko ratio of about 35.
where R is the gas constant and T is the absolute temperature.
The K+ fluxes in and out are equal when
RT is the thermal energy of a mole of particles. The ratio RT/zF has the dimensions of voltage and provides the electrical potential that gives a mole of charged particles with valence z an electrical energy (zFE) equal to the thermal energy (RT). At physiological temperatures, its value is about 25 mV for univalent ions where z = 1. The ratio of RT/zF establishes the range of potentials (tens of millivolts) that occur in cells.
Nernst Potential for Various Ions
The Nernst potential can be calculated for each ion known to have a selective channel in cell membranes: Na+, K+, Ca2+, and Cl− (Fig. 10-18). Given physiological gradients of these ions across the plasma membrane, the membrane potential could range from -98 to +128 mV, depending on which channels are open. In resting cells, only K+ channels are open, so the resting membrane potential is close to EK. Thus, variation of extracellular K+ concentration changes the membrane potential. In vertebrates, the normal extracellular K+ concentration is about 4 mM, but it varies from 2 mM to >8 mM in disease states. This fourfold variation in Ko changes the membrane potential by 30 to 37 mV, enough to affect cellular processes that are sensitive to the membrane potential. Other channels open and close selectively in response to extracellular or intracellular ligands, membrane potential, physical forces, or other factors (see text). Selective activation of channels is responsible for action potentials and other behavior of excitable membranes (see Fig. 11-6).
Membrane Capacitance
Capacitance depends on membrane area, thickness (physical separation between internal and external charges), and dielectric constant. If the capacitance is large, many ions must move to change the membrane potential. For cell membranes, the capacitance is approximately 1 mF/cm 2. One farad is 6 × 1018 charges per volt.
Charge Movement for a Small Cell
In Chapters 8 and 9, pumps and carriers were also noted to produce opposing membrane potentials when moving ions across membranes. This can be avoided by opening ion channels that short-circuit the change in membrane potential by providing pathways for counterions to move in the same direction or similar ions to move in the opposite direction across the membrane.
Net Current through Ion-Selective Channels
Another way to describe ionic current across a membrane is
where eo is the elementary charge. The dependence of current on membrane potential for real channels is complicated (Fig. 10-17B), so electrophysiologists approximate this current-voltage relationship of channels by a linear relationship, such as Ohm’s law (E = IR):
Opening Na+ and K+ channels has opposite effects because the ion concentration gradients are reversed. The Nernst potential for Na+ is about +65 mV in a typical cell, given a 10-fold excess of Na+ outside the cell. Current through an Na+ channel is negative (i.e., inward) at membrane potentials below ENa. Thus, if a Na+ channel opens on a cell in which E equals 0, the membrane potential rises toward ENa.
Consequence of Multiple Channel Types Opening Simultaneously
More than one type of open channel creates a situation more complicated than the equilibrium described by the Nernst potential for a single-ion species (Figs. 10-18 and 10-19). Consider a cell with physiological ion gradients and two channels—one open K+ channel and one open Na+ channel—having conductances of gK and gNa. The total current through these two channels is the sum of the individual currents:
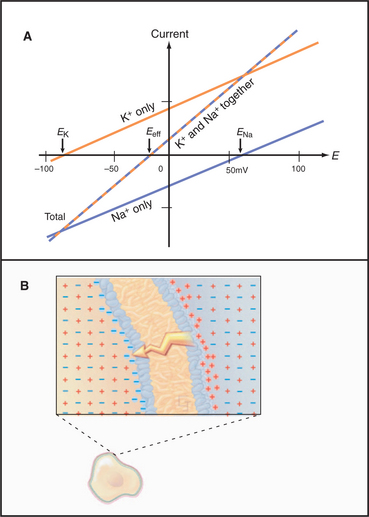
Figure 10-19 membrane potential and currents across a membrane with two types of channels. A, Dependence of currents on membrane potential resulting from opening either K+ channels or Na+ channels individually or together. In contrast to Figure 10-17, which shows ion fluxes in each direction, this is a plot of net current. EK and ENa are the equilibrium potentials (zero current) when only potassium or sodium channels are open. When both types of channels are open, the equilibrium potential (Eeff) is midway between the equilibrium potentials of the two types of channels. B, Distribution of positive (red) and negative (blue) ions across the plasma membrane and around a cell having a negative membrane potential. Excess negative charge builds up near the inside of the membrane, with the excess positive charge near the outside.
Which channel predominates? The equation for Itotal can also be written as
where the effective conductance geff and reversal potential Eeff are given by
and
The two channels together act like a single channel with an effective conductance equal to the sum of their conductances and a reversal potential that is the weighted average of their reversal potentials, that is, weighted by their relative conductances (Fig. 10-19A).
Charge Redistribution by Electrical Conduction
Most cellular ions have balancing counterions, whereas unpaired ions contributing to membrane potentials are confined to boundary layers near the membrane (Fig. 10-19B). Like-charged ions repel one another, so unpaired ions tend to accumulate at boundaries where they can move no farther.