Chapter 31 Magnetic Resonance Imaging
PRINCIPLES OF MAGNETIC RESONANCE IMAGING
Varying certain acquisition parameters, especially timing, changes the images so that their contrast varies depending more on their interactions with each other (T1-weighted imaging) or on their interactions with their local environment (T2-weighted imaging). Many other techniques and strategies are employed to vary the contrast, sensitivity, and efficiency of MRI and much more complete descriptions are available to the interested reader.1
MRI VERSUS OTHER IMAGING MODALITIES
The following sections include illustrative cases of normal anatomy and benign pathology frequently encountered by reproductive surgeons. The focus is on congenital and acquired pathology, but we do not discuss differentiation of a benign or malignant cyst. The assessment of malignant versus benign cysts is most commonly determined by ultrasonography (see Chapter 30).
NORMAL ANATOMY
The normal uterus, cervix, vagina, endometrium, and ovaries can be readily identified on T2-weighted images in a variety of planes. Also the plicae palmitae, the anterior and posterior fornix, the cul-de-sac, rectum, urinary bladder, and urethra are easily identified (Fig. 31-1). The uterine MRI anatomy can be subdivided. The signal intensity and thickness of these layers can vary based on the hormonal milieu. The myometrium is seen externally and tends to be intermediate in signal intensity. Centrally is the endometrium, which tends to be of increased signal on T2-weighted sequences. Between the two layers is a much darker, uniform layer, called the junctional zone. Although histologically similar to the adjacent myometrium, the difference in signal intensity is believed to result from a lower water content relative to the adjacent myometrium3 and the compact longitudinally oriented smooth muscle bundles oriented parallel to the endometrium4 (see Fig. 31-1). The radiologist, when assessing the uterus for pathology, evaluates the thickness, signal characteristics, and distinctness of each of these zones.
CONGENITAL ANOMALIES OF THE REPRODUCTIVE TRACT
The MRI appearance of müllerian anomalies directly corresponds to the findings on both hysterosalpingography and gross examination. Several potential anatomic observations can be made. In cases of uterine agenesis, no vaginal or uterine tissue is present (Fig. 31-2).
The arcuate uterus is a frequent müllerian anomaly that usually has little clinical significance and is identified as a contour or “bulge” of the fundus, with no changes associated with the endometrial canal (Fig. 31-3).
Several anomalies are easily determined by MRI, such as a unicornuate uterus where a single horn can be identified (Fig. 31-4). However, others are more difficult, especially when the decision of whether to proceed to surgery relies on the MRI diagnosis.
The most frequent differential diagnosis that a radiologist is asked to make on MRI is between a septate and bicornuate uterus. In a septate uterus, the septum can be seen extending from the fundus to any level in the uterine cavity up to the internal os. MRI can distinguish between a muscular and a fibrous septum based on the signal characteristics. A fibrous septum is of low signal on T2-weighted sequences, whereas the signal of a muscular septum will follow that of the myometrium, if obtained in the correct plane (Figs. 31-5 to 31-9). The basic concept holds that a bicornuate uterus would have a muscular septum and a septate uterus would be more fibrous. A septate uterus will have some “dimpling” of the fundus of the uterus, but the external surface of the uterus does not form two horns. In a bicornuate uterus the two horns are identified and the external fundal surface is clearly divided (Figs. 31-10 and 31-11). In a uterus didelphys the separate canals and cervices are easily identified. In cases where there is a vaginal septum, it is also easily identified (Fig. 31-12).
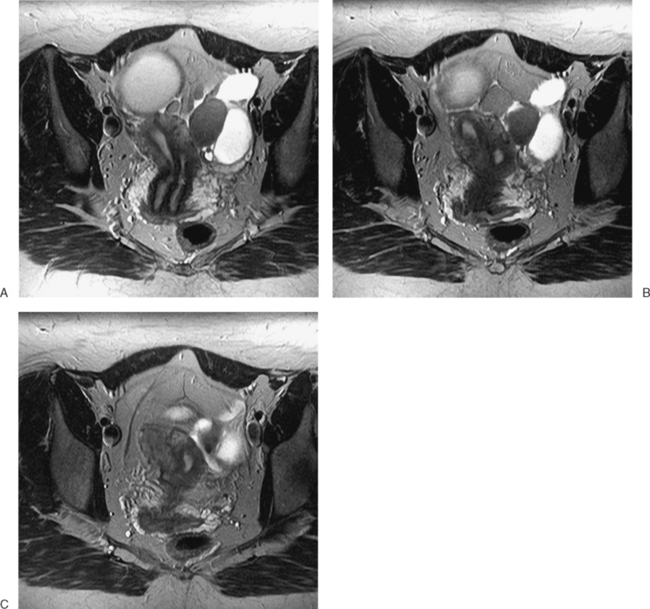
Figure 31-12 Uterus didelphys. Series of contiguous axial images (A–C) with two uterine canals and cervices.
In patients who present at puberty with acute pain and amenorrhea, the differential diagnosis should include an intact hymen, vaginal septum, and congenital absence of the cervix. In these cases, blood products of varying ages may be identified in the vaginal canal or uterus. Blood products range from bright through the spectrum of grey to dark. The darkest grey (almost black) represents hemosiderin (Fig. 31-13).
ACQUIRED ANOMALIES OF THE REPRODUCTIVE TRACT
Adenomyosis
The diffuse or localized forms of adenomyosis can be accurately detected with MRI. In diffuse adenomyosis, the thickness and signal characteristics of the junctional zone are evaluated. If the junctional zone is greater than 10 to 12mm thick, adenomyosis has been shown to be present. Rarely, uterine contractions5 have been shown to produce segmental thickening of the junctional zone, hence the reliance on these values. In adenomyosis, the junctional zone may contain regions of bright or high signal intensity on the T1– or T2-weighted images, corresponding to regions of hemorrhage6 (Figs. 31-14 to 31-17).
A focal adenomyoma may appear as mass with heterogeneous to low signal intensity, with an appearance that may be similar to a leiomyoma. However, the margins should be less distinct, corresponding to the interdigitation of the adenomyoma with the myometrium (see Fig. 31-15).
Leiomyoma
These benign tumors have a characteristic appearance on T2-weighted images. The margins are distinct from the surrounding myometrium, and the signal intensity is less than the surrounding myometrium, approaching that of the junctional zone. The degenerated myomas contain blood, blood degradation products, mucin, or hyaline and will have a heterogeneous appearance. Although MRI can distinguish these more complex myomata from the “typical” myoma, it cannot distinguish which of these substances are present. MRI has also proven useful in distinguishing fibroids from other solid pelvic masses and for localization, which may affect therapy7 (Figs. 31-18 and 31-19). However, there are no clear criteria that can distinguish malignant from benign lesions.
Post-Cesarean Section Defects
The high frequency of cesarean sections and potential dehiscence or rupture at the scar site has led to some interesting although clinically obscure observations on MRI. The scar or myometrial defect that corresponds to the surgical site can be identified on MRI (Fig. 31-20). However, there are no good data on the correlation between the scar characteristics and potential future reproductive outcomes.
OVARY
Normal ovaries are typically identified by the follicles and cysts (Fig. 31-22; see also Fig. 31-1). A major indication for MRI is often to distinguish a persistent physiologic cyst from a pathologic one. Hemorrhagic cysts are identified by high signal on a T1-weighted sequences without loss of signal on fat-suppressed sequences. This indicates blood or proteinaceous material within the cyst lumen (Fig. 31-23).
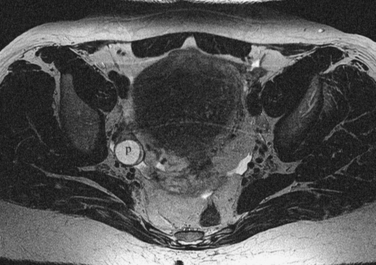
Figure 31-22 Ovary. Normal physiologic follicle (p) in right ovary on axial T2-weighted image through the pelvis.
Dermoid cysts are identified mainly by the fat component, with a sequence that will have fat as high signal intensity (bright) and a sequence that suppresses the fat signal (dark) lesion (Fig. 31-24).
Endometriosis cysts can be identified by the cyst contents, which will include blood or proteinaceous fluid of varying ages. Typically, there is a peripheral dark signal, indicating hemosiderin. There may be a relatively large amount of associated soft tissue, which would represent the associated inflammatory response or adhesions. Although MRI may identify endometriomas as small as 5 mm, it cannot identify the sheetlike peritoneal involvement (Figs. 31-25 and 31-26).
In peritoneal inclusion cysts, typically the ovary is not visualized within the fluid. The pelvic fluid will appear as a loculated collection (Fig. 31-27). Potential malignancies can be identified on MRI by similar criteria as those used in ultrasonography.
URETHRA
A urethral diverticulum is identified as a fluid collection adjacent to the urethra (Fig. 31-28). At times, the neck of the diverticulum can be identified. On occasion, complex fluid (typically proteinaceous) is identified. Only the wall of the diverticulum should enhance, without any nodularity, which would be concerning for a neoplastic process.
1 Mitchell DG, Schonholz L, Hilpert PL, et al. Zones of the uterus: Discrepancy between US and MR images. Radiology. 1990;174:827-831.
2 McCarthy S, Tauber C, Gore J. Female pelvic anatomy: MR assessment of variations during the menstrual cycle and with the use of oral contraceptives. Radiology. 1986;160:119-123.
3 McCarthy S, Scott G, Majumdai S, et al. Uterine junctional zone: MR study of water content and relaxation properties. Radiology. 1989;171:241-243.
4 Brown HK, Stoll BS, Nicosia SV, et al. Uterine junctional zone: Correlation between histologic findings and MR imaging. Radiology. 1991;179:409-413.
5 Kang S, Turner DA, Foster GC, et al. Adenomyosis: Specificity of 5 mm as the maximum normal uterine junctional zone thickness in MR images. Am J Roentgenology. 1996;34:1157-1182.
6 Togashi K, Nishimura K, Itoh K, et al. Adenomyosis: Diagnosis with MR imaging. Radiology. 1988;166:111-114.
7 Weinreb JC, Barkoff ND, Megibow A, Demopoulos R. The value of MR imaging in distinguishing leiomyomas from other solid pelvis masses when sonography is indeterminate. Am J Roentgenology. 1990;154:295-299.