Chapter 56 Lumbar Interbody Cages
Lumbar fusion procedures have become increasingly common in the treatment of degenerative conditions of the lumbar spine. The use of interbody cages to add structural stability and to improve fusion rates has become more common over time. It has been demonstrated biomechanically that the most effective means of eliminating motion between two vertebrae is through the disc space rather than the facet joints, transverse processes, or spinous processes.1 Traditionally, dorsolateral fusion has been associated with a higher pseudarthrosis rate than has a circumferential fusion. Furthermore, it has been shown that posterolateral fusion does not completely achieve immobilization of the motion segment despite the presence of a solid dorsolateral fusion.2 This result makes intuitive sense, as 80% of spinal loads are transmitted through the anterior column.
Interbody Fusion
Despite the aforementioned theoretical advantages of anterior column fusion, it is not yet clear whether clinical outcomes are improved in comparison with a dorsolateral fusion alone. The disadvantages of including an interbody device include the added cost; increased operative time; the risk of neurologic injury due to nerve root or dural sac retraction; and the long-term, potentially deleterious, effects of complete immobilization of a motion segment on the adjacent lumbar levels. One study found that at long-term follow-up (average 14 years), clinical outcomes were not correlated with disc height after ventral fusion using tricortical iliac bone.3
Also, cages that are inserted from a ventral approach can have varying degrees of lordosis (Fig. 56-1). The first tapered cage that was developed was the Lumbar Tapered Cage (LT cage; Medtronic, Memphis, TN) (Fig. 56-2). Most implants on the market today are tapered and have built-in lordosis. Although lordotic cages can be inserted from a dorsal approach, this degree of lordosis is limited. Most cages that are inserted from a dorsal approach have a tapered tip to allow initial insertion of the cage into the disc space. Therefore, the degree of lordosis that is achievable with a cage inserted from a dorsal approach is somewhat limited.
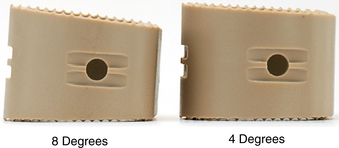
FIGURE 56-1 Anterior lumbar interbody fusion cages in different degrees of lordosis.
(Courtesy of Stryker Spine, AVS AL cage.)
One purported advantage of interbody fusion is the ability to obtain indirect decompression by increasing the size of the neural foramina. However, a recent study found that while an anterior lumbar interbody fusion (ALIF) restored foraminal height by 18.5% and lumbar lordosis by 6.2 degrees, transforaminal lumbar interbody fusion (TLIF) decreased foraminal height by 0.4% and decreased lumbar lordosis by 2.1 degrees.4 This decrease may be due to subsidence of the cage into the end plates. Also, after insertion of the cage from the dorsal approach, many surgeons apply compressive force across the pedicle screws to lock in the cage, and this may lead to a decrease in foraminal height.
In the standing position, 80% of spine loads are transmitted through the anterior column.5 The implant or graft must be capable of withstanding these loads to allow fusion to occur. Early on, autologous bicortical iliac crest autograft was the gold standard, supplemented with a screw and washer to keep the graft in place. However, high rates of pseudarthrosis, graft collapse, migration, and loss of correction were observed with the use of autologous iliac crest alone.6–11 Lumbar interbody cages have become more popular because of the high rate of pseudarthrosis associated with use of interbody bone graft alone.12,13 A cage provides immediate rigid axial mechanical support and stability postoperatively, allowing the graft material inside the cage as well as surrounding the cage to form a solid biologic fusion. There are a number of options to provide anterior column support, including fibular allograft, vascularized autograft (usually rib or fibula), vertical mesh cages (e.g., Harms cage; Fig. 56-3), carbon fiber cages (Brantigan cage; Fig. 56-4), cylindrical threaded cages (BAK, LT cages), and polyetheretherketone (PEEK) cages.
Anterior lumbar interbody fusion may be achieved with a stand-alone cage or supplemented with dorsal instrumentation. At present, there is no industry consensus for performing one procedure over the other. Proponents of the stand-alone ALIF contend that an exclusively ventral approach allows for superior preparation of the fusion surfaces while preserving the dorsal elements,14 which avoids perioperative morbidity related to the dissection of the spinal muscles and complications from the dorsal instrumentation.15 On the other hand, advocates for dorsal instrumentation assert that supplementary dorsal stabilization increases system constraint, creating an environment that is more conducive to fusion.16
A number of biomechanical studies have investigated the stabilization provided by stand-alone ALIF cages versus those supplemented with dorsal instrumentation. In general, stand-alone interbody cages significantly reduce flexion and lateral bending compared to intact spines.14,17,18 Constraint of axial rotation, however, has not been as successful,14,16,19,20 although studies suggest that ALIF procedures generate better stabilization in torsion than does posterior lumbar interbody fusion (PLIF).21 Regardless of the surgical approach, interbody cages do not stabilize in extension,18,21–23 although it is unclear whether the lack of stabilization in extension is clinically relevant and should warrant dorsal instrumentation.21
It is widely accepted that supplementary dorsal instrumentation further increases range of motion constraint and stiffness in one or more planes compared to stand-alone ALIF cages.16,18,19,23 However, the constraint parameters that are ideal for facilitating fusion have not been defined. If supplementary dorsal instrumentation is required to achieve a stable environment for fusion, the advantages of ALIF over dorsal procedures are lost.
Clinical studies comparing stand-alone interbody cages and cages with dorsal stabilization have been equivocal. Fusion rates of between 82% and 98% have been reported for both procedures,24,25 and patient satisfaction rates have also been similar between stand-alone and circumferential procedures. In a study of 71 patients who underwent stand-alone ALIF with bilateral low-profile interbody fusion cages, the overall fusion rate was 86% with an average time to fusion of 10 months. While the single-level fusion rate was 93%, the rates for two- and three-level procedures were lower, at 55% and 67%, respectively. This is consistent with other published data reporting fusion rates for both ALIF and PLIF procedures of around 90% to 94%, with lower fusion rates for multilevel procedures. This may suggest that stand-alone procedures are most beneficial for single-level fusions.24
Additionally, Greenough et al. concluded that ALIF for a single level was associated with significantly less perioperative morbidity than were dorsolateral fusion and instrumentation, although he did not find a significant difference in clinical outcome between the two approaches.15 Nichols et al. found that the addition of a ventral plate to ALIF provided stability similar to that of supplemental dorsal fixation, with dorsal fixation providing only additional constraint in lateral bending.26
Historical Perspective
The concept of interbody fusion was first introduced in the 1940s by Ralph Cloward, who pioneered the posterior lumbar interbody fusion technique. Toward the end of the 1970s, Dr. George Bagby introduced the stainless steel basket as an adjunct to spinal arthrodesis. He implanted a cervical interbody cage made of stainless steel in a racehorse with successful fusion. He called his technique distraction-compression stabilization. In the 1980s, Kuslich and others adapted Bagby’s basket for human use. Subsequently, threaded PLIF cages were introduced.27 Threaded PLIF cages were thought to have biomechanical advantages over traditional dorsolateral fusion, including providing anterior column support, placement closer to the vertebral center of rotation, and a reduced bone graft requirement.28 The FDA approved anterior lumbar interbody fusion cages in 1996. Until the end of the 1990s, most cages were made of titanium. It was not until the late 1990s that the first radiolucent polymer implants (PEEK cages) were introduced. Over the last decade, the field of interbody cage technology has exploded, and a variety of implants have been introduced into the market.
Cage Material and Design
The following features are desirable in the design of an interbody cage:
• A cage should ideally have a hollow region of sufficient size to allow packing of bone graft or bone graft substitute.
• It should be structurally sturdy so that it can withstand the great forces applied to it in the immediate postoperative period.
• It should have a modulus of elasticity that is similar to that of vertebral bone to optimize fusion and avoid subsidence.
• It should have ridges or teeth to resist ventral migration or retropulsion into the canal. Serrations on the top and bottom surfaces of the cage may improve fixation strength.
• It should be radiolucent to allow visualization of fusion on radiographs.
• If inserted from a dorsal approach (TLIF or PLIF) it should be tapered, with a bullet-shaped tip to allow easier initial insertion into the disc space.
The stiffness of a cage has been found to influence fusion rates.29,30 Ideally, a cage would have a modulus of elasticity that is similar to that of vertebral bone, which would optimize the load transfer between the cage and the adjacent vertebral bodies and reduce the effects of stress shielding on the graft material. Carbon fiber cages have a modulus of elasticity closer to that of cortical bone.31 In contrast, metal and titanium cages exceed the stiffness of the vertebral bone. The modulus of elasticity of stainless steel and titanium implants is 200 and 110 GPa, respectively,32 compared with that of vertebral trabecular and cortical bone, which is 2.1 and 2.4 GPa, respectively.33,34
Titanium cages have the disadvantage of incomplete radiographic assessment of the fusion mass. Furthermore, owing to the mismatch of modulus of elasticity of titanium and vertebral bone, the stiffness of titanium cages may cause subsidence into the vertebral end plates. PEEK is a semicrystalline aromatic polymer used as a structural spacer that has gained popularity because of its similar modulus of elasticity to that of bone and its radiolucency. It can be used in conjunction with carbon fiber reinforcement or as pure PEEK. Radiomarker dots are used at the ventral and dorsal aspects of the cage so that the surgeon can see the implant on radiographs. PEEK is also MRI and CT compatible and does not create significant implant artifact on these imaging studies. PEEK cages come in a variety of sizes (Fig. 56-5).
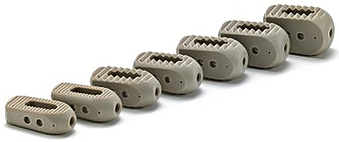
FIGURE 56-5 Tapered PEEK (polyetheretherketone) cages for transforaminal lumbar interbody fusion.
(Courtesy of Stryker Spine, AVS PL cages.)
There is some biomechanical evidence showing lower primary fixation and initial stability of PEEK cages compared to titanium cages of equal dimensions.18 Despite this evidence, PEEK implants have been shown to have good to excellent clinical outcomes35–37 and are widely used. Particularly when augmented with dorsal instrumentation, PEEK cages have been shown to provide stability similar to that of titanium cages, reduce stress at the end plates adjacent to the cage, and increase load transfer through the graft.38
In a study of lordosis correction in circumferential arthrodesis using PEEK cages, Rousseau et al.37 found that immediate postsurgery increases in lumber lordosis were lost at subsequent follow-up. Lordosis loss was statistically related to the initial increase in lordosis, cage size, patient age, and presence of dorsal instrumentation and level of fusion. This tendency to restore preoperative sagittal balance occurred despite a 98% fusion rate and 86% excellent or good clinical outcomes. Similar loss of lordosis correction, despite solid fusion and good clinical outcomes, has been found in other studies regardless of interbody cage material.3,39
Bioabsorbable cages have also been designed. These cages are absorbed over time, thus leaving only a bony fusion between vertebrae without any foreign material in the spinal segment. There is limited experience with the clinical application of resorbable implants in lumbar fusion surgery, and studies are contradictory. Jiya et al.40 randomized 26 patients to undergo instrumented posterior lumbar interbody fusion with either a nonresorbable PEEK cage or a resorbable poly(L-lactide-co-D,L-lactide) (PLDLLA) cage. The fusion rate was significantly higher with the PEEK cage compared with the PLDLLA cage. Furthermore, the PLDLLA cage showed a significantly higher rate of subsidence compared with the PEEK cage. Other studies have evaluated the fusion rate and its relationship to cage stiffness, evaluating a poly-L-latic acid (PLLA) cage versus a titanium cage.29,30 After 6 months, increased interbody fusion was seen with the PLLA cages. An in vitro study showed that PLLA cages were mechanically sufficient directly after implantation.41 Other studies have supported the use of resorbable cages, with fusion rates being comparable to those of nonresorbable implants without adverse effects related to the implant.42–44
Subsidence
Intraoperatively, during discectomy and end-plate preparation, the surgeon should aim to remove the cartilaginous end plate but preserve the bony end plate. Removing the cartilaginous end plate is important for improving the vascularity to aid the process of fusion. However, removing the bony end plate may compromise the stability of the end plate, weaken the compressive strength of the vertebral body, and lead to subsidence of the interbody device. There is some evidence to support this claim: Oxland et al. reported that strength was mechanically reduced by approximately 33% with resection of the bony end plate compared to the rate without resection.45 While removal of the end plate to expose bleeding cancellous bone is advantageous from a fusion standpoint, it may weaken the bone and increase the risk of implant subsidence. Although the bony end plate itself is very thin (usually <0.5 mm thick),46,47 it likely serves to distribute loads evenly over the underlying cancellous bone. Most surgeons leave the bony end plate intact to preserve structural support.
The periphery of the end plate is stronger than the center, and the dorsolateral portions (just ventral to the pedicles) are the strongest regions of the end plate. The dorsolateral sites have more than twice the strength of the central end plate. Given this pattern, if a small portion of the end plate is removed in the central portion of the end plate, the risk of subsidence may not be substantially increased,45 and the exposed central cancellous bone may improve fusion. Removal of the end plate in the peripheral regions, particularly in regions where the cage is in contact with the end plate (depending on cage design), likely increases the risk of subsidence.
Cage material can affect the degree of subsidence. Previous studies have shown that anterior lumbar interbody fusion using iliac allograft or autograft has a very high rate of subsidence.48 Hollowell et al.49 loaded thoracic vertebrae in compression comparing a titanium mesh cage, humerus, tricortical iliac graft, triple-rib strut graft, and single-rib graft on intact vertebrae or on a cancellous trough of vertebrae. The titanium mesh cage construct provided the greatest resistance to axial load. However, there is concern that rigid metal cages have a high incidence of subsidence. Unfortunately, interstudy comparison of subsidence is complicated by inconsistency in defining criteria and the lack of a standard measuring technique. Schiffman et al.24 studied the radiographs and clinical outcomes from 56 patients at least 12 months following surgery with bilateral low-profile interbody fusion cages (BAK/Proximity; Minneapolis, MN) and found minimal evidence of subsidence (<2 mm) and no significant differences in amount of subsidence between one- and two-level procedures. Additionally, he found no association between the amount of subsidence and disc height, fusion status, change in lordosis, or patient satisfaction. In other studies, Beutler and Peppelman39 and Eck et al.50 found subsidence greater than 2 mm in 10% and 14% of patients, respectively, who underwent noninstrumented ALIF with titanium mesh cages. Likewise, subsidence incidence was not associated with loss of lordosis or patient satisfaction. Increased subsidence was associated with greater reaming depth and with larger cage diameters.39 Furthermore, ideal positioning of interbody cages at the dorsolateral sites was identified as an important factor to limit subsidence. Another study found that the addition of dorsal instrumentation did not change the compressive strength of the cage-vertebra interface, indicating that dorsal fixation was unlikely to contribute to subsidence.51
Factors Affecting Construct Rigidity
Anular tension is influenced primarily by the vertical height of the cage. Axial “oversizing” of the cage leads to increased anular tension, which may improve the rigidity of the construct.52 Most systems have trials that can be inserted into the disc space to assess ligament tension. One method of assessing the size of the implant is to measure the height of a normal lumbar disc one level above or below the level to be fused on preoperative imaging studies. This height approximates the desired height at the diseased level. However, intraoperative assessment of anular tension with trial implants is the most reliable method of determining cage size. Undersized cages with anular tissue relaxation may lead to device loosening, fusion failure, and possibly migration of the implant.
Vertebral bone quality, or end-plate strength, is critical to cage stability.45,49,53–55 The dorsolateral region of the end plate, the region near the pedicle base, has the greatest resistance to subsidence, while the central region provides the least resistance.56 The additional cortical bone at the pedicle base may account for this improved strength.56 This is of clinical importance in performing a TLIF, in which smaller cages are used. Positioning the cage dorsolaterally in these cases maximizes stability of the construct.56 The disadvantage of this position is that it may increase the likelihood of cage retropulsion into the canal. For this reason, cages that are inserted through a dorsal approach are typically countersunk relative to the dorsal vertebral body by at least 3 to 4 mm.
Cage size also affects construct stability. In compression, larger cages have greater maximum load to failure of the end plates than smaller cages do.55 Wider implants that are supported by the periphery of the end plate provide superior stability.57 For this reason, cages that are inserted through the lateral approach (e.g., extreme lateral interbody fusion or direct lateral interbody fusion approaches) likely provide superior compressive stability in comparison to smaller cages that are inserted through the dorsal approach. Closkey et al. have shown the association between contact area of the cage and compressive strength.53 A smaller cage applies more load to the central portion of the end plate, which is much weaker than the periphery of the end plate. Many cages, particularly those that are inserted through the dorsal approach, have too small an area of graft bone/host bone contact surface area. The small total contact surface area between the device and the vertebral end plates may also result in subsidence.
Cage features such as serrations or spikes may also affect the overall stability. There is biomechanical evidence for this. Buttermann et al. compared three cage types: a PEEK spacer with small ridges, a modular interbody device with end-plate spikes, and a dual tapered threaded interbody cage. Cages with end-plate spikes provided improved motion segment rigidity in bending modes and particularly in torsion.14 End-plate engagement with spikes is beneficial in limiting torsion.14 Like the threaded cage, the spiked cage engages the end plates, but with spikes instead of threads (Fig. 56-6).
The question that remains is how much rigidity is necessary. The degree of micromotion that would not compromise biologic fusion is not known. Pilliar et al.58 studied the effect of micromotion on bone ingrowth into porous surfaced implants and found that while a small amount of micromotion of up to 28 μm does not affect bone ingrowth, a large amount of micromotion of over 150 μm can produce fibrous tissue development at the implant–end plate interface. If optimal construct rigidity is not obtained, supplemental fixation should be considered, particularly in cases of osteopenia, end-plate disruption, or excessive anular laxity. In osteoporotic spine, supplemental fixation has been recommended.55
Complications and Management
Failure to achieve adequate distraction of the anulus fibrosus and undersizing the cage can risk pseudarthrosis and cage migration with potential injury to the neural elements. Vascular injury can occur if the cage migrates ventrally in the lower lumbar spine. Furthermore, ventral migration of the graft can lead to loss of correction and segmental kyphosis. Dorsal fusion and instrumentation, with compression across the pedicle screws, add further stability and enhance the fusion rate. The use of fluoroscopy during end-plate preparation and cage insertion may lower the incidence of complications that are related to suboptimal positioning of the cage. In placing cages from the dorsal approach, countersinking the implant by 3 to 4 mm may lower the risk of neural element impingement if micromigration of the implant occurs (Fig. 56-7).
If dorsal cage migration occurs after a TLIF procedure such that the neural elements are at risk, a ventral approach is the safest method of retrieving the cage without causing nerve root injury. In such a setting, if a repeat dorsal approach is utilized, the surgeon is likely to encounter scarring around the nerve roots, and retrieval of the cage can lead to nerve root injury or a ventral dural tear. For these reasons, a ventral approach may be the safest technique to remove the implant. This approach would also allow an effective anterior interbody fusion, as pseudarthrosis was likely the cause of the implant migration.
Bagby G.W. Arthrodesis by the distraction-compression method using a stainless steel implant. Orthopedics. 1988;11:931-934.
Brantigan J.W., Steffee A.D. A carbon fiber implant to aid interbody lumbar fusion: two-year clinical results in the first 26 patients. Spine (Phila Pa 1976). 1993;18:2106-2117.
Hollowell J.P., Vollmer D.G., Wilson C.R., et al. Biomechanical analysis of thoracolumbar interbody constructs: how important is the endplate? Spine (Phila Pa 1976). 1996;21:1032-1036.
Kuslich S.D., Danielson G., Dowdle J.D., et al. Four-year follow-up results of lumbar spine arthrodesis using the Bagby and Kuslich lumbar fusion cage. Spine (Phila Pa 1976). 2000;25:2656-2662.
Lowe T.G., Hashim S., Wilson L.A., et al. A biomechanical study of regional endplate strength and cage morphology as it relates to structural interbody support. Spine (Phila Pa 1976). 2004;29:2389-2394.
Oxland T.R., Grant J.P., Dvorak M.F., et al. Effects of endplate removal on the structural properties of the lower lumbar vertebral bodies. Spine (Phila Pa 1976). 2003;28:771-777.
Rousseau M.A., Lazennec J.Y., Saillant G. Circumferential arthrodesis using PEEK cages at the lumbar spine. J Spinal Disord Tech. 2007;20:278-281.
1. Zdeblick T.A., Smith G.R., Warden K.E., et al. Two-point fixation of the lumbar spine. Differential stability in rotation. Spine (Phila Pa 1976). 1991;16(Suppl 6):S298-S301.
2. Rolander S.D. Motion of the lumbar spine with special reference to the stabilizing effect of posterior fusion. An experimental study on autopsy specimens. Acta Orthop Scand. 1966;90(Suppl):1-144.
3. Cheung K.M., Zhang Y.G., Lu D.S., et al. Reduction of disc space distraction after anterior lumbar interbody fusion with autologous iliac crest graft. Spine (Phila Pa 1976). 2003;28(13):1385-1389.
4. Hsieh P.C., Koski T.R., O’Shaughnessy B.A. Anterior lumbar interbody fusion in comparison with transforaminal lumbar interbody fusion: implications for the restoration of foraminal height, local disc angle, lumbar lordosis, and sagittal balance. J Neurosurg Spine. 2007;7(4):379-386.
5. Yang K., King A. Mechanism of facet load transmission as a hypothesis for low back pain. Spine (Phila Pa 1976). 1984;9:557-565.
6. Siff T.E., Kamaric E., Noble P.C., et al. Femoral ring versus fibular strut allografts in anterior lumbar interbody arthrodesis: a biomechanical analysis. Spine (Phila Pa 1976). 1999;24(7):659-665.
7. Ishihara H., Osada R., Kanamori M., et al. Minimum 10 year follow-up study of anterior lumbar interbody fusion for isthmic spondylolisthesis. J Spinal Disord. 2001;14(2):91-99.
8. Janssen M.E., Nguyen C., Beckham R., et al. Biological cages. Eur Spine J. 2000;9(suppl 1):S102-S109.
9. Loguidice V.A., Johnson R.G., Guyer R.D., et al. Anterior lumbar interbody fusion. Spine (Phila Pa 1976). 1988;13:366-369.
10. Soini J. Lumbar disc space heights after external fixation and anterior interbody fusion: a prospective 2-year follow-up of clinical and radiographic results. J Spinal Disord. 1994;7:487-494.
11. Pfeiffer M., Griss P., Haake M., et al. Standardized evaluation of long-term results after anterior lumbar interbody fusion. Eur Spine J. 1996;5:299-307.
12. Cloward R.B. The treatment of ruptured lumbar intervertebral discs by vertebral body fusion: indications, operative technique, after care. J Neurosurg. 1953;10:154-168.
13. Crock H.V. Observations on the management of failed spinal operations. J Bone Joint Surg [Br]. 1976;58:193-199.
14. Buttermann G.R., Beaubien B.P., Freeman A.L., et al. Interbody device endplate engagement effects on motion segment biomechanics. Spine J. 2009;9(7):564-573.
15. Greenough C.G., Peterson M.D., Hadlow S., Fraser R.D. Instrumented posterolateral lumbar fusion: results and comparison with anterior interbody fusion. Spine (Phila Pa 1976). 1998;23(4):479-486.
16. Vadapalli S., Robon M., Biyani A., et al. Effect of lumbar interbody cage geometry on construct stability: a cadaveric study. Spine (Phila Pa 1976). 2006;31:2189-2194.
17. Kuslich S.D., Danielson G., Dowdle J.D., et al. Four-year follow-up results of lumbar spine arthrodesis using the Bagby and Kuslich lumbar fusion cage. Spine (Phila Pa 1976). 2000;25:2656-2662.
18. Spruit M., Falk R.G., Beckmann L., et al. The in vitro stabilising effect of polyetheretherketone cages versus a titanium cage of similar design for anterior lumbar interbody fusion. Eur Spine J. 2005;14:752-758.
19. Gerber M., Crawford N.R., Chamberlain R.H., et al. Biomechanical assessment of anterior lumbar interbody fusion with an anterior lumbosacral fixation screw-plate: comparison to stand-alone anterior lumbar interbody fusion and anterior lumbar interbody fusion with pedicle screws in an unstable human cadaver model. Spine (Phila Pa 1976). 2006;31:762-768.
20. Tsantrizos A., Andreou A., Aebi M., Steffen T. Biomechanical stability of five stand-alone anterior lumbar interbody fusion constructs. Eur Spine J. 2006;9:14-22.
21. Oxland T.R., Lund T. Biomechanics of stand-alone cages and cages in combination with posterior fixation: a literature review. Eur Spine J. 2000;9(Suppl 1):S95-S101.
22. Oxland T.R., Lund T., Jost B., et al. The relative importance of vertebral bone density and disc degeneration in spinal flexibility and interbody implant performance. An in vitro study. Spine (Phila Pa 1976). 1996;21:2558-2569.
23. Burton D., McIff T., Fox T., et al. Biomechanical analysis of posterior fixation techniques in a 360 degrees arthrodesis model. Spine (Phila Pa 1976). 2005;30:2765-2771.
24. Schiffman M., Brau S.A., Henderson R., Gimmestad G. Bilateral implantation of low-profile interbody fusion cages: subsidence, lordosis, and fusion analysis. Spine J. 2003;3:377-387.
25. McAfee P. Interbody fusion cages in reconstruction operations on the spine. J Bone Joint Surg [Am]. 1999;81:859-880.
26. Nichols T.A., Yantzer B.K., Alamda S., et al. Augmentation of an anterior lumbar interbody fusion with an anterior plate or pedicle fixation: a comparative biomechanical in vitro study. J Neurosurg Spine. 2007;6(3):267-271.
27. Bagby G.W. Arthrodesis by the distraction-compression method using a stainless steel implant. Orthopedics. 1988;11:931-934.
28. Cassinelli E., Wallach C., Hanscom B., et al. Prospective clinical outcomes of revision fusion surgery in patients with pseudarthrosis after posterior lumbar interbody fusion using stand-alone metallic cages. Spine J. 2006;6(4):428-434.
29. van Dijk M., Smit T.H., Sugihara S., et al. The effect of cage stiffness on the rate of lumbar interbody fusion. An in vivo model using poly (L-lactic acid) and titanium cages. Spine (Phila Pa 1976). 2002;27:682-688.
30. Smit T.H., Müller R., Dijk van M., Wuisman P.I.J.M. Changes in bone architecture during spinal fusion: three years follow-up and the role of cage stiffness. Spine (Phila Pa 1976). 2003;28:1802-1809.
31. Brantigan J.W., Steffee A.D. A carbon fiber implant to aid interbody lumbar fusion: two-year clinical results in the first 26 patients. Spine (Phila Pa 1976). 1993;18:2106-2117.
32. Tunc D.C. Orientruded polylactide based body-absorbable osteosynthesis devices: a short review. J Biomater Sci Polym Ed. 1995;7:375-380.
33. Guo E. Mechanical properties of cortical bone and cancellous bone tissue. In: Cowin S.C., editor. Bone mechanics handbook. Boca Raton, FL: CRC Press; 2001:pp 1-90.
34. Van der Elst M., Patka P., van der Werken C. Biodegradable implants in fracture fixation: state of the art. Unfallchirurg. 2000;103:178-182.
35. Sears W. Posterior lumbar interbody fusion for degenerative spondylolisthesis: restoration of sagittal balance using insert-and-rotate interbody spacers. Spine J. 2005;5:170-179.
36. Cutler A.R., Siddiqui S., Mohan A.L., et al. Comparison of polyetheretherketone cages with femoral cortical bone allograft as a single-piece interbody spacer in transforaminal lumbar interbody fusion. J Neurosurg Spine. 2006;5:534-539.
37. Rousseau M.A., Lazennec J.Y., Saillant G. Circumferential arthrodesis using PEEK cages at the lumbar spine. J Spinal Disord Tech. 2007;20:278-281.
38. Vadapalli S., Sairyo K., Goel V.K., et al. Biomechanical rationale for using polyetheretherketone (PEEK) spacers for lumbar interbody fusion: a finite element study. Spine (Phila Pa 1976). 2006;31:E992-E998.
39. Beutler W.J., Peppelman W.C. Anterior lumbar fusion with paired BAK standard and paired BAK proximity cages: subsidence incidence, subsidence factors, and clinical outcome. Spine J. 2003;3:289-293.
40. Jiya T., Smit T., Deddens J., Mullender M. Posterior lumbar interbody fusion nonresorbable poly-ether-ether-ketone versus resorbable poly-L-lactide-co-D, L-lactide fusion devices: a prospective, randomized study to assess fusion and clinical outcome. Spine (Phila Pa 1976). 2009;34(3):233-237.
41. van Dijk M., Smit T.H., Arnoe M.F., et al. The use of poly-L-lactic acid in lumbar interbody cages: design and biomechanical evaluation in vitro. Eur Spine J. 2003;12:34-40.
42. Coe J.D. Instrumented transforaminal lumbar interbody fusion with bioabsorbable polymer implants and iliac crest autograft. Neurosurg Focus. 2004;16:E11.
43. Coe J.D., Vaccaro A.R. Instrumented transforaminal lumbar interbody fusion with bioresorbable polymer implants and iliac crest autograft. Spine (Phila Pa 1976). 2005;30:S76-S83.
44. Couture D.E., Branch C.L.Jr. Posterior lumbar interbody fusion with bioabsorbable spacers and local autograft in a series of 27 patients. Neurosurg Focus. 2004;16:E80.
45. Oxland T.R., Grant J.P., Dvorak M.F., Fisher C.G. Effects of endplate removal on the structural properties of the lower lumbar vertebral bodies. Spine (Phila Pa 1976). 2003;28:771-777.
46. Roberts S., McCall I.W., Menage J., et al. Does the thickness of the vertebral subchondral bone reflect the composition of the intervertebral disc? Eur Spine J. 1997;6:385-389.
47. Amstutz H.C., Sissons H.A. The structure of the vertebral spongiosa. J Bone Joint Surg [Br]. 1969;51:540-550.
48. Dennis S., Watkins R., Landaker S., et al. Comparison of disc space heights after anterior lumbar interbody fusion. Spine (Phila Pa 1976). 1989;14:876-878.
49. Hollowell J.P., Vollmer D.G., Wilson C.R., et al. Biomechanical analysis of thoracolumbar interbody constructs: how important is the endplate? Spine. 1996;21:1032-1036.
50. Eck K.R., Bridwell K.H., Ungacta F.F., et al. Analysis of titanium mesh cages in adults with minimum two-year follow-up. Spine (Phila Pa 1976). 2000;25:2407-2415.
51. Jost B., Cripton P.A., Lund T., et al. Compressive strength of interbody cages in the lumbar spine: the effect of cage shape, posterior instrumentation and bone density. Eur Spine J. 1998;7:132-141.
52. Goh J.C., Wong H.K., Thambyah A., Yu C.S. Influence of PLIF cage size on lumbar spine stability. Spine (Phila Pa 1976). 2000;25:35-39.
53. Closkey R., Parson R., Lee C., et al. Mechanics of interbody spinal fusion: analysis of bone graft area. Spine (Phila Pa 1976). 1993;18:1011-1015.
54. Grant P., Oxland T., Dvorak M. Mapping the structural properties of the lumbosacral vertebral endplates. Spine (Phila Pa 1976). 2001;26:889-896.
55. Hasegawa K., Abe M., Washio T., et al. An experimental study on the interface strength between titanium mesh cage and vertebra in reference to vertebral bone mineral density. Spine (Phila Pa 1976). 2001;26:957-963.
56. Lowe T.G., Hashim S., Wilson L.A., et al. A biomechanical study of regional endplate strength and cage morphology as it relates to structural interbody support. Spine (Phila Pa 1976). 2004;29:2389-2394.
57. Steffen T., Tsantrizos A., Aebi M. Effect of implant design and endplate preparation on the compressive strength of interbody fusion constructs. Spine (Phila Pa 1976). 2000;25:1077-1084.
58. Pilliar R.M., Lee J.M., Maniatopoulos C. Observations on the effect of movement on bone ingrowth into porous-surfaced implants. Clin Orthop. 1986;208:108-113.