CHAPTER 269 Intraoperative Monitoring of the Spinal Cord and Nerve Roots
Intraoperative neurophysiologic monitoring is an important technique that is essential for improvement of safety in modern complex spine surgery.1–3 In its ideal form, a properly engineered intraoperative monitoring environment provides real-time feedback information to the surgical team about the functional status of the spinal cord and nerve roots under surgical manipulation so that preventive or corrective actions can be taken to avoid irreversible injuries.4–8 To gain universal acceptance and facilitate utilization, an ideal monitoring environment should include multiple properly designed human-machine interfaces, some of which involve biomedical engineering. It is also imperative that an optimal team of neurophysiologists and neurosurgeons be assembled to properly and efficiently use these technologies. Intraoperative monitoring modalities should have high sensitivity, high specificity, low invasiveness, and ease of use. Although numerous intraoperative monitoring techniques have been investigated since the days of Penfield and Boldrey,9 no single monitoring method has been able to satisfy these requirements in clinical spine surgery practice. However, general consensus regarding the use of intraoperative neurophysiologic monitoring is emerging as the evidence for monitoring continues to build,10 thereby encouraging optimism for the development of an ideal intraoperative monitoring modality.
Somatosensory Evoked Potentials
Monitoring of dorsal column integrity with SSEPs is the most commonly used technique in spine surgery.11 Early monitoring solely with SSEPs resulted in false-negative results in multiple reports and thus necessitated the development of multimodality monitoring.12–14 Nevertheless, SSEPs remain a standard modality with demonstrated improved neurological outcomes in corrective procedures for spinal deformity,4,6,7 and it serves as a building block for more complex monitoring arrangements. Multiple reports of the use of SSEPs in spine surgery, ranging from the early 1980s to current prospective studies involving larger patient populations, have demonstrated an improvement in outcome.15–17
In SSEP monitoring, stimulation electrodes excite controlled repetitive action potentials propagating from peripheral nerves to dorsal roots, the dorsomedial tracts of the spinal cord, and eventually the contralateral sensory cortex, along which multiple recording sites may be anatomically accessible. Typically, platinum subdermal needle electrodes are used for both purposes. Stimulation sites for the upper extremity include the median and ulnar nerves. For the lower extremity, the posterior tibial nerve is a standard location. Bipolar stimulation (requiring both cathode and anode placement) is typically used. Electrode impedances are key parameters to ensure contact and reduce artifacts. Impedance values below 1 to 5 kΩ are clinically used thresholds for interelectrode or individual electrode measurements, respectively. Typical stimulation waveforms are 250-µsec-duration square-wave pulse trains at 4.7 Hz, with stimulation amplitudes ranging from 20 to 40 mA, depending on patient variables. Recording electrode positioning follows set standards, such as the international 10-20 system (Fig. 269-1).18 For example, Cv2-Fpz for the N31 subcortical response at the cervicomedullary junction and Cpz-Fpz for P37/N45 responses at the cerebral sensory cortex are commonly used. Other recording sites such as Erb’s point are also used. It is worth noting that after neural stimulation of the lower extremities (e.g., at the posterior tibial nerve), SSEP monitoring also reflects the physiologic integrity of the spinocerebellar tracts, which involve the dorsal nucleus of Clarke’s column between T1 and L2.
Subcortical signals have lower signal amplitude and a reduced signal-to-noise ratio within a given amplifier gain and averaging setting but are more resistant to changes in depth of anesthesia. Cortical signals have larger amplitude and a higher signal-to-noise ratio and therefore tend to be more reliable electrically but are sensitive to anesthetic-induced changes. This tradeoff is illustrated in the improvement in reliability, approaching 93% to 98% in selected studies,4,19–26 when using multiple cortical and subcortical recording sites. Recent advances in digitization equipment, filtering algorithms, and signal processing have resulted in the continued reduction of system complexity, which may potentially translate into a reduction in cost or an increase in the number of concurrently monitored channels.
Motor Evoked Potentials
Transcranial electrical or magnetic stimulation of the cerebral motor cortex causes muscle activation, which can be detected peripherally to provide additional monitoring coverage specifically designed to detect motor deficits.27,29 This is particularly important with intramedullary resections and in procedures with a risk for vascular compromise of the anterior spinal artery and its associated vascular territory, which can affect motor function. Initial work28,29 paved the way for current single-pulse stimulation techniques, in which corticospinal tract recordings of direct waves (D waves) are detected, and multipulse stimulation techniques, in which short pulse trains are used to elicit CMAPs.30
Typically, in patients under general anesthesia, MEP monitoring involves an electrode montage over the scalp based on the international 10-20 electroencephalography system. The standard setup uses C3-4 for upper limb MEPs and C1-2 for the lower limbs, with selection of corkscrew, needle, or cup electrodes being based on the clinical scenario and the patient’s age. Skull thickness, electrode type and impedance, and the need for depth of stimulation determine the required stimulation amplitude. Typically, selective stimulation is preferred, and therefore superficial coverage of a small cortical area is used to provide activation of a single limb. With few exceptions, D waves directly generated by the electrical activation of axons of cortical motor neurons are recorded in patients under general anesthesia. This is due, in part, to the fact that vertically oriented excitatory chains of neurons terminating on the cortical motor neuron are blocked by anesthetics, thus abolishing indirect activation of the motor neuron and the associated indirect (I) waves.1 For D-wave recording, one or more sterile intraoperative epidural electrodes are required and placed caudally and cranially relative to the surgical lesion. Signal amplification typically requires a gain setting of 10,000× and bandpass filtering between 1.5 and 1700 Hz. Only a few traces are required for averaging, and the stimulation can be repeated at 0.5 to 2 Hz to provide real-time feedback.1 A reduction in D-wave amplitude of greater than 50% has been shown to correlate with new postoperative motor deficits.31 Myogenic MEPs use a short (5 to 7) pulse train with a 4-msec interpulse interval at the transcranial electrodes. Recordings of the resultant CMAPs at sites such as the thenar muscles or tibialis anterior are amplified at 10,000× and bandpass-filtered between 1.5 and 853 Hz. Again, multitrace signal averaging is not frequently required, and real-time feedback is feasible.
Multipulse stimulation and CMAP detection provide the benefit of monitoring the corticospinal tract, in addition to the distal muscle functional units, including the neuromuscular junction. Although the muscle units provide a physiologic nonlinear amplifier for the MEPs, which improves the electrical signal-to-noise ratio, the nonlinear relationship and preoperative muscle atrophy may cloud the interpretation. Thus, myogenic MEPs are typically interpreted as an all-or-none phenomenon with regard to preoperative clinical assessment and electrophysiologic findings. When MEP amplitude is lost, immediate intraoperative intervention can result in complete reversal, which can be sensitive and specific in predicting new postsurgical motor deficits.32 In conditions in which reliable transcranially stimulated MEPs are difficult to obtain, neurogenic MEPs, obtained through percutaneous stimulation, have been investigated.33,34 When combined with SSEP monitoring of the dorsal column, MEPs provide additional coverage for the ventral corticospinal tract and improve the efficacy of intraoperative monitoring (Fig. 269-2). The intermittent nature of MEP monitoring requires the surgeon to actively request interval measurements. These measurements are generally first taken as a baseline before surgery and then at surgical opening, during exposures approaching the spinal cord, on manipulation of the spinal cord, during different phases of intramedullary resection, and at closure. Close interaction between the neurophysiologist and the surgical team also allows periodic pauses intraoperatively to ensure that reliable recordings are being obtained throughout the procedure.
Electromyography
Typically, free-running or spontaneous EMG activities can be recorded with paired stainless steel needle electrodes insulated to within 5 mm of the tip and transdermally inserted into the target muscle. For example, lumbosacral surgeries may use bilateral quadriceps femoris, tibialis anterior, and gastrocnemius recordings to achieve myotomal coverage from L2 to S2. Electrode impedance below 5 kΩ and interelectrode impedance below 1 kΩ ensure electrical contact. EMG recordings are obtained with a 200-µV/division gain, bandpass filtering between 10 and 5000 Hz, and analysis time of 1.5 to 2.0 seconds. When the nerve or nerve root is compressed, irritated, or injured, spontaneous firing occurs and activation of the corresponding motor unit is monitored by EMG, with different manifestations noted that may correlate with increasing degrees of injury. Morphologic descriptions such as spikes, bursts, train activity, and neurotonic discharges correspond to individual discharges, brief bundles of discharges, persistent regularly repeated discharge patterns, and persistent prolonged bursting, respectively. Intraoperative neuromonitoring units typically contain loudspeakers that convert the electrical activity into audio feedback to the surgical team in real time. A single burst of activity synchronized to surgical manipulations is useful for functional identification and correlation of specific roots and typically does not result in adverse outcomes. Sustained activity (>2 seconds) prompts the electrophysiologist to alert the surgeon to potential neurological deficits. The overall level of EMG activity may also correlate with outcome; however, further research is required to provide definitive sensitivity and specificity. It is also possible that during EMG-monitored procedures, false-positive results may be the direct result of intraoperative monitoring, with excessive EMG activity usually resulting in alteration or cessation of surgical manipulation and irritation of the nerve roots in question. Figure 269-3 shows typical intraoperative free-running EMG monitoring.
Triggered or evoked EMG activity can be obtained from suspicious tissues involving scar, tumor, filum terminale, or any tissue structure that may contain functional neural elements. Evoked EMG can assess the integrity between the interrogated tissue and the muscle units being recorded. Typical stimulation uses a bipolar probe intraoperatively that delivers monophasic square-wave pulses of 3 Hz with a duration of 100 µsec and a constant current source of less than 10 mA (generally 1 to 5 mA to elicit muscle responses). Figure 269-4 shows a disposable bipolar stimulator probe (Axon Systems, Hauppauge, NY.) designed for evoked EMG monitoring.
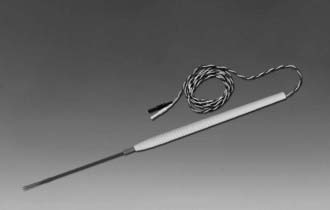
FIGURE 269-4 Bipolar disposable stimulator probe (Axon Systems, Hauppauge, NY) for evoked electromyographic monitoring.
Because all EMG recordings are performed simultaneously, multichannel recording units are required. Historically, for technical reasons, only limited anatomic areas can be recorded by a given set of equipment. With the advent of new low-cost digital equipment, new intraoperative monitoring units with 32, 64, or more channels are available, thus increasing the anatomic areas that can be recorded in a given surgery. Continuous EMG has high sensitivity but low specificity in predicting postoperative neurological deficits,10,35,36 EMG is therefore typically used as part of a comprehensive monitoring regimen in most spine surgeries.
Additional Options for Comprehensive Multimodality Monitoring
Combining the aforementioned modalities may help improve the overall sensitivity and specificity of intraoperative monitoring.37 A prospective analysis of a consecutive series of cases demonstrated reciprocal sensitivity and specificity of SSEPs and EMG in thoracolumbar spine surgery, where SSEPs had a sensitivity of 28.6% and a specificity of 94.7% and EMG had a sensitivity of 100% and a specificity of 23.7% for the detection of new postoperative neurological deficits.38 Similarly, the combination of MEPs and SSEPs has also been investigated to address the relatively low sensitivity of SSEPs in a variety of spinal procedures.32,39
Additional options for comprehensive monitoring can include coverage for anal or bladder sphincter function (or both), especially during microsurgical procedures involving the lumbosacral spinal canal for resection of tumor or untethering of the spinal cord. The associated risk for loss of urinary bladder or anal sphincter function, or both, can be assessed by monitoring the S2-4 spinal nerve roots. Individual7,40 or simultaneous41 monitoring of the EAS and EUS by EMG has been investigated. Figure 269-5 shows some urinary bladder and anal sphincter electrodes (Medtronic Dantec Skovlunde, Denmark) used for EUS and EAS EMG monitoring.
Insertion of a urethral ring electrode is facilitated with a two-way Foley catheter (10 or 14 French); the electrode is placed over the catheter tip in sterile fashion and positioned approximately 1 to 2 cm proximal to the inflated balloon. Insertion of an anal sphincter electrode is performed manually, similar to rectal temperature probes, except that care must be taken to ensure proper electrical contact between the electrodes and the sphincter muscle with the aid of conductive gels. EMG recording parameters for the EAS and EUS are similar to those for other continuous EMG settings.41 Figure 269-6 shows a typical intraoperative monitoring record with combined SSEP, EMG, EUS, and EAS measurements during resection of an L3 schwannoma.
Krassioukov and colleagues demonstrated that in approximately 90% of patients, individual intraoperative EAS or EUS activities were recorded.41 In 11% of the cases (3/27 patients), simultaneous activities in both the EAS and EUS were observed. Therefore, continuous and simultaneous recordings for both the EAS and EUS are advocated to ensure proper and adequate coverage of the pudendal nerve branches (S2-4) and provide sufficient information to avoid adverse outcomes given the significant impact of sphincter dysfunction on patients.
Anesthetic Considerations
In general, intraoperative monitoring requires an increased level of finesse in anesthesia so that reliable recording and comparisons can be made. SSEP amplitude and latency are especially affected by halogenated or nitrous oxide–based agents. Myogenic MEPs require total intravenous anesthesia without neuromuscular blockade to allow CMAP monitoring. Under such conditions, close communication between the surgical and anesthetic teams during all phases of the operation becomes paramount for ensuring safety during surgical exposure and the efficacy of monitoring during peridural or intradural microsurgical maneuvers. Typically, MEP monitoring requires induction with short-lived muscle relaxants, continuous infusion of propofol and fentanyl, and nitrous oxide use kept to a low level (not exceeding 50% by volume). A single intravenous injection of rocuronium at the time of induction offers a recovery index of approximately 30 minutes less than other anesthetic agents and wears off at the time of EMG recordings. Intermittent train-of-four testing provides confirmation that a low level of neuromuscular blockade is present to allow consistent EMG monitoring. Significant changes in SSEPs can be the result of changes in body temperature and MAP, which are constantly monitored.42 Halogenated agents are avoided if possible.30 Bolus injections of anesthetics can cause variations in the recorded waveforms, and their use needs to be minimized or coordinated with the neurophysiologist.1
Illustrative Cases
Case Example 1
Intramedullary Tumor
A 66-year-old woman with a complex past medical history, including breast cancer and lower limb osteosarcoma, was evaluated for progressive gait difficulty over a 9-month period. Her clinical examination confirmed myelopathy. In addition, she had diffuse bilateral sensory impairment in the legs with the right side worse than the left. Serial magnetic resonance imaging suggested a T8-9 intramedullary mass measuring 1.2 cm anteroposteriorly, 1.0 cm in width, and 4.7 cm craniocaudally (Fig. 269-7). There was extensive edema within the cord above and below the lesion. On a magnetic resonance angiogram, the mass demonstrated very early arterial enhancement, homogeneous enhancement in the venous phase, and very large dilated vessels surrounding the lesion. The patient was electively booked for T7 to T9 laminectomy and microsurgical resection of this intramedullary mass. After induction and intubation, baseline intraoperative recordings of SSEPs and MEPs were obtained. As shown in Figure 269-8A to D, there was a marked difference in the SSEPs recorded from the upper and the lower extremities. Because the upper extremity SSEPs demonstrated good amplitude and the expected waveform, they provided the evidence to exclude anesthetics or other effects as the cause of the low signal amplitude in the lower extremity SSEPs. It was concluded that the intramedullary lesion had caused significant cord compression at the thoracic level and thus SSEP monitoring would be insufficient for this case. Fortunately, multichannel MEP recordings (Fig. 269-8E to H) demonstrated adequate reproducibility and redundancy for intraoperative monitoring. With MEPs for monitoring and intraoperative ultrasound for localization, complete microsurgical resection of the lesion, including both the extramedullary and intramedullary components, was carried out. There were no changes in MEPs during the operation. There were no immediate postoperative mortor deficits and no worsening of her sensory deficits. The final pathology was consistent with capillary hemangioma.
Case Example 2
Adult Tethered Cord Syndrome
A 49-year-old woman with a childhood diagnosis of spinal bifida and tethered conus previously underwent L3-4 laminectomy, untethering, and duraplasty. She had symmetrical 4/5 lower extremity power, progressive spastic gait difficulties, patchy sensory loss below the level of the umbilicus, and episodic urinary and fecal incontinence. Over a 4-month period, her neurological condition worsened from using two canes to being wheelchair-bound. Magnetic resonance imaging of the thoracic and lumbar spine at 3 T demonstrated a complex intradural mass adherent to the posterior aspect of the cord at L1-2, with the cord tethering down to the L4 level. A large posterior defect in the spinal canal from T12 to L4 was present, along with a large sac at the level of the dysraphism that was herniating through the bony defect. There was also spondylolisthesis at the L5-S1 level, anomalous segmentation of the lumbosacral junction, anomalous formation of pedicles in the lower lumbar region, and a thoracolumbar scoliotic deformity (Fig. 269-9). The patient underwent L3 to S1 laminectomy, bilateral foraminotomy, durotomy, intradural lysis of adhesions, detethering of the spinal cord, and thoracolumbosacral reconstruction with instrumented fusion from T10 to the sacrum. The patient was set up for SSEP, segmental EMG, and EAS monitoring.
As shown in Figure 269-10A to D, there were marked differences in the SSEPs recorded from the upper and the lower extremities. The low signal amplitudes in the SSEPs from the lower limbs and the random fluctuations suggested severe sensory impairment, in accordance with this patient’s condition. The upper limb SSEPs had good amplitude and provided confidence in the monitoring technique and state of anesthesia. Segmental EMG and EAS recordings were then used for monitoring throughout the duration of this procedure. There were no changes in the upper limb SSEPs. The activity in the EMG and EAS recordings provided real-time warnings to the surgical team during the detethering and instrumentation portions of the operation. There were no perioperative neurological deficits, and the patient reported improvement in the extent of her lower limb neuropathic pain.
Future Developments for Combining Surgical Microscopy with Intraoperative Monitoring
Modern complex spine surgeries are typically assisted with surgical microscopes. Although current intraoperative monitoring strategies rely on electrode-based stimulation and recordings, it may be possible to directly visualize the myelinated axonal structures in vivo with improved microscopy techniques, especially with the recent advances in optics and laser technology. For example, a novel imaging modality based on coherent anti-Stokes Raman scattering (CARS) has been demonstrated to be sensitive to lipid-rich cells such as adipocytes, Schwann cells, and oligodendrocytes without the use of exogenous labeling. This technique uses the C-H Raman vibration that is present in lipids by exciting it with two lasers tuned to different wavelengths such that the difference in their frequency corresponds to the frequency of the vibration. Through a vibrationally resonant third-order nonlinear process (coherent anti–Stokes Raman scattering), a photon at a higher frequency is emitted to provide lipid-based contrast. By virtue of being a nonlinear process, it benefits from three-dimensional sectioning and high spatial resolution similar to two-photon fluorescence excitation. When combined with in vivo video rate microscopy, CARS imaging (Fig. 269-11) is ideally positioned to directly visualize and quantify myelinated fibers intraoperatively. It may also be possible to directly visualize membrane depolarization and action potentials through the fibers by using advanced microscopy techniques such as second-harmonics generation (SHG) imaging. In the future, a hybrid system consisting of electrode-based stimulation and microscopy imaging–based recordings may be able to provide spatially localized functional information of the spinal cord and nerve roots to the surgical team.
Conclusion
Table 269-1 provides a summary of the respective advantages and disadvantages of the most commonly used intraoperative monitoring techniques; it is intended to serve as a quick reference on what should be monitored during a particular procedure. Multimodality intraoperative monitoring with an increasing number of simultaneously recorded channels is becoming economically and technically feasible and thus may provide the optimal monitoring environment for complex spine surgery, especially for patients with preexisting spinal cord deficits. As with any technique that involves multiple disciplines, constant communication, regular use, and attempts to fully integrate it into daily clinical practice will make intraoperative monitoring a valuable asset to spine surgery centers.
Accadbled F, Henry P, de Gauzy JS, et al. Spinal cord monitoring in scoliosis surgery using an epidural electrode. Results of a prospective, consecutive series of 191 cases. Spine. 2006;31:2614-2623.
Costa P, Bruno A, Bonzanino M, et al. Somatosensory- and motor-evoked potential monitoring during spine and spinal cord surgery. Spinal Cord. 2007;45:86-91.
Daube JR. Monitoring spinal function during surgery. In: Brown WF, Bolton MJ, Aminoff MJ, editors. Neuromuscular Function and Disease, vol 2. Philadelphia: WB Saunders; 2002:1839-1856.
Deletis V, Shils JL. Neurophysiology in Neurosurgery: A Modern Intraoperative Approach. Philadelphia: Academic Press; 2002.
Gunnarsson T, Krassioukov AV, Sarjeant R, et al. Real-time continuous intraoperative electromyographic and somatosensory evoked potential recordings in spinal surgery: Correlation of clinical and electrophysiologic findings in a prospective, consecutive series of 213 cases. Spine. 2004;29:677-684.
Hilibrand AS, Schwartz DM, Sethuraman V, et al. Comparison of transcranial electric motor and somatosensory evoked potential monitoring during cervical spine surgery. J Bone Joint Surg Am. 2004;86:1248-1253.
Kelleher MO, Furlan JC, Fehlings MG. Is neuromonitoring beneficial during spinal surgery? In: Wright JG, ed. Evidence Based Orthopaedics. Philadelphia: Saunders Elsevier; 2009;46-55.
Kombos T, Suess O, Da Silva C, et al. Impact of somatosensory evoked potential monitoring on cervical surgery. J Clin Neurophysiol. 2003;20:122-128.
Krassioukov AV, Sarjeant R, Arkia H, et al. Multimodality intraoperative monitoring during complex lumbosacral procedures: Indications, techniques, and long-term follow-up review of 61 consecutive cases. J Neurosurg Spine. 2004;1:243-253.
Padberg AM, Thuet ED. Intraoperative electrophysiologic monitoring: Considerations for complex spinal surgery. Neurosurg Clin N Am. 2006;17:205-226.
Paradiso G, Lee GY, Sarjeant R, et al. Multimodality intraoperative neurophysiologic monitoring findings during surgery for adult tethered cord syndrome: Analysis of a series of 44 patients with long-term follow-up. Spine. 2006;31:2095-2102.
Paradiso G, Lee GY, Sarjeant R, et al. Multimodality neurophysiological monitoring during surgery for adult tethered cord syndrome. J Clin Neurosci. 2005;12:934-936.
Pelosi L, Lamb J, Grevitt M, et al. Combined monitoring of motor and somatosensory evoked potentials in orthopaedic spinal surgery. Clin Neurophysiol. 2002;113:1082-1091.
Quinones-Hinojosa A, Gadkary CA, Gulati M, et al. Neuro-physiological monitoring for safe surgical tethered cord syndrome release in adults. Surg Neurol. 2004;62:127-133.
Seyal M, Mull B. Mechanisms of signal change during intraoperative somatosensory evoked potential monitoring of the spinal cord. J Clin Neurophysiol. 2002;19:409-415.
Wiedemayer H, Sandalcioglu IE, Armbruster W, et al. False negative findings in intraoperative SEP monitoring: Analysis of 658 consecutive neurosurgical cases and review of published reports. J Neurol Neurosurg Psychiatry. 2004;75:280-286.
1 Deletis V, Shils JL. Neurophysiology in Neurosurgery: A Modern Intraoperative Approach. Philadelphia: Academic Press; 2002.
2 Loftus CM, Traynelis VC. Intraoperative Monitoring Techniques in Neurosurgery. New York: McGraw-Hill; 1994.
3 Moller AR. Intraoperative Neurophysiologic Monitoring. Luxembourg: Harwood Academic Publishers; 1995.
4 Nuwer MR. Spinal cord monitoring. Muscle Nerve. 1999;22:1620-1630.
5 Daube JR. Monitoring spinal function during surgery. In: Brown WF, Bolton MJ, Aminoff MJ, editors. Neuromuscular Function and Disease, vol 2. Philadelphia: WB Saunders; 2002:1839-1856.
6 Herdmann J, Deletis V, Edmonds HLJr, et al. Spinal cord and nerve root monitoring in spine surgery and related procedures. Spine. 1996;21:879-885.
7 Kothbauer K, Schmid UD, Seiler RW, et al. Intraoperative motor and sensory monitoring of the cauda equina. Neurosurgery. 1994;34:702-707.
8 Quinones-Hinojosa A, Gadkary CA, Gulati M, et al. Neuro-physiological monitoring for safe surgical tethered cord syndrome release in adults. Surg Neurol. 2004;62:127-133.
9 Penfield W, Boldrey E. Somatic motor and sensory representation in the cerebral cortex of man as studied by electrical stimulation. Brain. 1937;60:389-443.
10 Kelleher MO, Furlan JC, Fehlings MG. Is neuromonitoring beneficial during spinal surgery? In: Wright JG, ed. Evidence Based Orthopaedics. Philadelphia: Saunders Elsevier; 2009:46-55.
11 Padberg AM, Thuet ED. Intraoperative electrophysiologic monitoring: Considerations for complex spinal surgery. Neurosurg Clin N Am. 2006;17:205-226.
12 Lesser RP, Raudzens P, Luders H, et al. Postoperative neurological deficits may occur despite unchanged intraoperative somatosensory evoked potentials Ann Neurol. 1986.2;19:22-25
13 Ben-David B, Haller G, Taylor P. Anterior spinal fusion complicated by paraplegia. A case report of a false-negative somatosensory-evoked potential. Spine. 1987;12:536-539.
14 Wiedemayer H, Sandalcioglu IE, Armbruster W, et al. False negative findings in intraoperative SEP monitoring: Analysis of 658 consecutive neurosurgical cases and review of published reports. J Neurol Neurosurg Psychiatry. 2004;75:280-286.
15 Grundy BL, Nash CLJr, Brown RH. Deliberate hypotension for spinal fusion: Prospective randomized study with evoked potential monitoring. Can Anaesth Soc J. 1982;29:452-462.
16 Epstein NE, Danto J, Nardi D. Evaluation of intraoperative somatosensory-evoked potential monitoring during 100 cervical operations. Spine. 1993;18:737-747.
17 Kombos T, Suess O, Da Silva C, et al. Impact of somatosensory evoked potential monitoring on cervical surgery. J Clin Neurophysiol. 2003;20:122-128.
18 Jasper HH. The ten-twenty electrode system of the international federation. Electroencephalogr Clin Neurophysiol. 1958;10:371-375.
19 Nuwer MR, Dawson EG, Carlson LG, et al. Somatosensory evoked potential spinal cord monitoring reduces neurologic deficits after scoliosis surgery: results of a large multicenter survey. Electroencephalogr Clin Neurophysiol. 1995;96:6-11.
20 Nuwer MR. Evoked Potential Monitoring in the Operating Room. New York: Raven Press; 1986.
21 Owen JH, Sponseller PD, Szymanski J, et al. Efficacy of multimodality spinal cord monitoring during surgery for neuromuscular scoliosis. Spine. 1995;20:34-43.
22 Owen JH. The application of intraoperative monitoring during surgery for spinal deformity. Spine. 1999;15:2649-2662.
23 Lubicky JP, Spadoro JA, Yuan HA, et al. Variability of somatosensory evoked potential monitoring during spinal surgery. Spine. 1989;14:790-798.
24 Ashkenaze D, Mudiyan R, Boachie-Adjei O, et al. Efficacy of spinal cord monitoring in neuromuscular scoliosis. Spine. 1993;18:1627-1633.
25 He JM, Tang Y. Combined application of two somatosensory evoked potential techniques at various recording points for monitoring the onset of stretch spinal injury during rhachial orthomorphia. Zhongguo Linchuang Kangfu. 2005;9:164-165.
26 McCaffrey MT. Somatosensory evoked-potential monitoring during spinal surgery. Semin Spine Surg. 1997;9:309-314.
27 Malmivuo J, Plonsey R. Bioelectromagnetism—Principles and Application of Bioelectric and Biomagnetic Fields. New York: Oxford University Press; 1995.
28 Hill DK, McDonnel MJ, Merton PA. Direct stimulation of the abductor pollicis in man. J Physiol. 1980;305:2.
29 Merton PA, Morton HB. Electrical stimulation of human motor and visual cortex through the scalp. J Physiol. 1980;305:9-10.
30 Taniguichi M, Cedzich C, Schramm J. Modification of cortical stimulation for motor evoked potentials under general anesthesia: Technical description. Neurosurgery. 1993;32:219-226.
31 Morota N, Deletis V. Constantini S, et al. The role of motor evoked potentials during surgery for intramedullary spinal cord tumors. Neurosurgery. 1997;41:1327-1336.
32 Hilibrand AS, Schwartz DM, Sethuraman V, et al. Comparison of transcranial electric motor and somatosensory evoked potential monitoring during cervical spine surgery. J Bone Joint Surg Am. 2004;86:1248-1253.
33 Accadbled F, Henry P, de Gauzy JS, et al. Spinal cord monitoring in scoliosis surgery using an epidural electrode. Results of a prospective, consecutive series of 191 cases. Spine. 2006;31:2614-2623.
34 Komanetsky RM, Padberg AM, Lenke LG, et al. Neurogenic motor evoked potentials: A prospective comparison of stimulation methods in spinal deformity surgery. J Spinal Disord. 1998;11:21-28.
35 Paradiso G, Lee GY, Sarjeant R, et al. Multimodality neurophysiological monitoring during surgery for adult tethered cord syndrome. J Clin Neurosci. 2005;12:934-936.
36 Paradiso G, Lee GY, Sarjeant R, et al. Multimodality intraoperative neurophysiologic monitoring findings during surgery for adult tethered cord syndrome: Analysis of a series of 44 patients with long-term follow-up. Spine. 2006;31:2095-2102.
37 Pelosi L, Lamb J, Grevitt M, et al. Combined monitoring of motor and somatosensory evoked potentials in orthopaedic spinal surgery. Clin Neurophysiol. 2002;113:1082-1091.
38 Gunnarsson T, Krassioukov AV, Sarjeant R, et al. Real-time continuous intraoperative electromyographic and somatosensory evoked potential recordings in spinal surgery: Correlation of clinical and electrophysiologic findings in a prospective, consecutive series of 213 cases. Spine. 2004;29:677-684.
39 Costa P, Bruno A, Bonzanino M, et al. Somatosensory- and motor-evoked potential monitoring during spine and spinal cord surgery. Spinal Cord. 2007;45:86-91.
40 James HE, Mulcahy JJ, Walsh JW, et al. Use of anal sphincter electromyography during operations on the conus medullaris and sacral nerve roots. Neurosurgery. 1979;4:521-523.
41 Krassioukov AV, Sarjeant R, Arkia H, et al. Multimodality intraoperative monitoring during complex lumbosacral procedures: Indications, techniques, and long-term follow-up review of 61 consecutive cases. J Neurosurg Spine. 2004;1:243-253.
42 Seyal M, Mull B. Mechanisms of signal change during intraoperative somatosensory evoked potential monitoring of the spinal cord. J Clin Neurophysiol. 2002;19:409-415.