63 Intradiscal Biologics
A Potential Minimally Invasive Cure for Symptomatic Degenerative Disc Disease?
KEY POINTS
Introduction
The development of a cell-based, biological replacement to restore, maintain, and improve the function of damaged tissues and organs has become the en vogue frontier to developing potential novel approaches to patient cures. The intervertebral disc (IVD) undergoes very extensive degenerative changes (Figure 63-1) with the various macro- and microtraumas that come with age and daily life activities. Individual differences have been demonstrated in young individuals exhibiting the disc of an elderly person and vice versa. It is generally accepted that an extremely prevalent rate and degree of asymptomatic disc degeneration exists in the general population. Therefore, differentiating normal aging from symptomatic pathological degeneration is very difficult and cannot be assessed by simply identifying the most abnormal disc on imaging (Figure 63-2). At this time, controlled provocative lumbar discography (Figure 63-3) remains our best clinical test to identify a physiologically painful structurally compromised IVD. The term ‘‘discogenic low back pain” is the term often used to indicate degenerative disc disease associated with concordant pain.
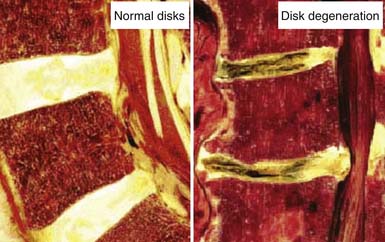
FIGURE 63-1 Macroscopic pathoanatomy evident in disc degeneration compared side by side to healthy intervertebral discs.
Functional Anatomy of the Intervertebral Disc
It is also important to note that the intervertebral disc is a largely avascular structure. With increasing age, as growth and skeletal maturation proceed, degenerative processes begin to change the morphology and therefore the function of the disc. The most widely accepted conceptual model of spinal segmental degeneration was proposed by Kirkaldy-Willis.1 In this model, the nucleus pulposus of degenerated discs is characterized by a decreased water and proteoglycan content leading to the loss of its gel-like appearance and hydrostatic properties. Degenerative changes of the annulus fibrosus are less obvious, but result in irregular lamellae with the collagen and elastin networks becoming more disorganized. Replacing the gel-like structure of the nucleus pulposus with fibrocartilaginous tissue results in decreased flexibility and therefore often in cleft formation with fissures. Up to 50% of the cells show signs of necrosis and some of them reveal signs of apoptosis, potentially resulting in cell loss from the disc.2 Although there is broad consensus about these hallmarks of degeneration, the question of whether revascularization and/or reinnervation of the inner parts of the disc may occur during degeneration is still a topic of debate.3 Although studies have described revascularization, possibly accompanied by reinnervation, of the inner parts of the IVD, it is not completely clear at which stage of degeneration these occur.3 Clarification of this question is of special importance since the interplay between neovascularization and neoinnervation might be of crucial importance for the pain sensation caused by degenerated discs. Answers to these questions may ultimately determine the rate-limiting step to the potential of biologic cures of symptomatic degenerative disc disease.
Causes of Degenerative Disc Disease
Degenerative disc disease is a complex process with a multifactorial etiology. Nutritional effects, mechanical load, and genetics all likely have contributory pathologic effects on the IVD. Of these, nutrition and removal of waste products likely play a special role in realizing the potential that intradiscal biologics may ultimately hold. Insufficient nutritional supply of the cells is thought to be the major obstacle contributing to degenerative disc disease. Cells of the IVD face the precarious situation of having to maintain a huge extracellular matrix with a ‘’fragile’’ supply of nutrients that is easily disturbed because the IVD is avascular and nutrition is dependent on diffusion. Because of the size of the intervertebral disc, the nutrients need to diffuse from a capillary network in the vertebral bodies, through the endplates and the disc matrix to the cells in the nucleus of the disc. The supply of nutrition becomes more restricted as the originally cartilaginous endplates become calcified as the degenerative process progresses. As glucose and oxygen is restricted because of diffusion distances, the removal of metabolic waste such as lactic acid becomes critically impaired. Measurements have demonstrated that as oxygen concentrations were very low in the nucleus and increased toward the disc surface, the lactic acid concentration showed the reverse profile. The buildup of lactic acid results in an intradiscal environment with a lowered pH. Low oxygen concentrations and acidic pH adversely affect the synthetic activity and proteoglycan synthesis rates of disc cells. This toxic environment may lead to a fall in proteoglycan content and therefore to degenerative disc disease. This suboptimal environment may lead to increased cell death and therefore reduced cell numbers in the disc.4 Ultimately, the result of poor nutritional supply of the IVD is that very few remaining cells are confronted with the task of maintaining an extensive matrix. Unfortunately, it likely holds true that the progression of matrix degeneration becomes irreversible once the cell density falls below a minimal threshold.
Therapeutic Biologic Strategies
Intradiscal Injection of a “Naked” Biologically Active Factor
Percutaneous intradiscal injection would provide the most straightforward approach to delivery of an active biologic factor to the disc cells (see Figure 63-3). Although direct application of potentially beneficial factors, mostly proteins like growth factors, cytokines, or anabolic enzymes, has been used frequently in vitro, few studies have been published attempting this approach in vivo. Promising results have been reported after injecting rabbit lumbar IVD in vivo with osteogenic protein-1 (OP-1), a growth factor belonging to the Transforming Growth Factor Beta (TGF-b) superfamily of growth factors. Direct injection of this growth factor resulted in significantly increased proteoglycan synthesis and restoration of disc height that was found to be stable up to 24 weeks after injection.5,6 Additional studies demonstrated that OP-1 injection exhibited a physiologic effect by inhibiting pain-related behavior in a rat disc degeneration model.7,8 Subsequently, Chubinskaya and colleagues9 documented the anticatabolic effect of intradiscal injection of OP-1 in a rat model by demonstrating reduced immunostaining for aggrecanase, Matrix Metalloproteinase (MMP)-13, substance P, Tumor necrosis factor (TNF)-α, and Interleukin (IL)-1β. Because substance P is a neuropeptide linked with inflammation and pain, the aforementioned reduction in level of this noxious protein support the previously stated physiologic inhibition of pain-related behavior.7–9 Furthermore, Miyamoto and coworkers10 were able to demonstrate that intradiscal injection of OP-1 restored the biomechanical properties of IVDs in the rabbit model of degenerative disc disease. They reported not only that a single injection of OP-1 significantly restored IVD height, but also that the treated discs demonstrated a higher viscous and elastic modulus due to increased proteoglycan content in the nucleus as well as increased collagen content in the nucleus and annulus. Concerns regarding the potential of ectopic bone formation in the epidural space with OP-1 therapies was addressed by Kawakami and associates.11 They demonstrated that there was no macroscopic evidence of ectopic bone formation, no motor paresis, and no behavioral differences to motor stimuli with epidural administration of OP-1 in a rat model. The aforementioned studies demonstrate the feasibility of direct injection, yet this technique may be limited to the presence of disc cells that are still healthy, numerous, and able to respond to a biologically active agent. Taking into context the decreasing viability and synthetic activity of human disc cells during progressive degeneration, future directions for this technique may be best suited for success in the younger patient population with discogenic Low back pain (LBP) due to modestly degenerated discs in synergistic combinations with other biologically active agents.
As opposed to injecting a biologically active enzyme or growth factor, Klein and colleagues12 published a clinical pilot study utilizing direct injection of a mixture of matrix components and aiding components known to induce proteoglycan synthesis. This solution of glucosamine and chondroitin sulfate combined with hypertonic dextrose and dimethylsulfoxide was injected into 30 patients who exhibited concordant pain on provocative lumbar discography. These patients responded well regarding reduction in a disability score and a visual analogue pain score at an average follow-up of 13 months. The authors suggested that the good outcome might be due to the combination of several components resulting in a parallel replenishing of the matrix by increased proteoglycan synthesis and induction of disc repair by simultaneous induction of multiple growth factors. This approach might prove superior to the injection of a single bioactive factor. It is conceivable that the injected matrix components are able to modulate and improve the intradiscal environment, enabling the disc cells, even in a degenerated disc, to react to the resulting secretion of growth factors and continue with the maintenance of the cellular environment. However, from this pilot study it is not clear if the injected components will be contained inside the disc in heavily degenerated discs and therefore be able to ensure a prolonged beneficial effect on the disc cells. Further controlled comparative studies are required before any therapeutic conclusions can be rendered.
Common to these injection techniques is the concern that the aforementioned demonstrated short-term effects might cease when the originally injected material has been consumed or is lost to the disc cells by diffusion. In order to provide the disc with a continuing supply of biologically active factors, it would be desirable to continuously produce the biologic of choice or include the substance in a pharmacological slow-release system as suggested for the use of growth factors.13 Considering these data, it is conceivable that combination therapy of growth factor(s) and matrix replenishment might be the way to obtain a more sustained improvement of degenerated discs. This approach may also allow for expansion of the previously stated limitations with application of this technique to various grades of degeneration because of the requirement of a certain density of healthy disc cells.
Gene Therapy Approaches
Prolonged supply of the discal matrix with a beneficial agent could be achieved by genetically modifying disc cells to produce a desired gene product. Because of advances in molecular genetics, techniques are readily available to insert genetic elements (DNA) into almost any type of target cell. These genetic elements usually consist of the gene encoding the desired product and a control element modulating the expression of the respective gene. Typically, two strategies can be used to achieve expression of the desired gene at the targeted site. Direct, or in vivo, gene therapy requires the direct introduction of the gene of interest into resident cells in situ (see Figure 63-4), whereas indirect, or ex vivo, gene therapy requires the removal of target cells, introduction of the gene of interest in vitro and implantation of the transformed cells (see Figure 63-5).14 Uptake of the desired genetic material, usually in the form of pure “naked” DNA, can be optimized by application of a carrier, also called vector. Viral vectors are very efficient transporters of genetic material; they are able to enter mammalian cells, taking over DNA replication and the protein expression machinery. For the purposes of gene therapy, several engineered viruses are available that have the original viral genome removed or inactivated and, in addition, are modified to not replicate or exhibit their pathogenicity. Of interest, these viruses vary in their ability to integrate the transferred DNA into the host cell genome, their ability to invade dividing or nondividing cells, and their infection efficiency. Because of the properties of the IVD and its cells, the virus of choice needs to efficiently infect nondividing, quiescent cells. Furthermore, the low cell density inside the disc might hamper efficient infection of a sufficient fraction of the disc cells. On the other hand, the avascular and contained IVD might provide an advantageous environment to achieve high concentrations of the injected viral vector, leading to higher efficiency of the infection process while also lessening the danger of an immune response against viral proteins.
Studies have demonstrated that adenoviral vectors are able to efficiently transform disc cells from various species. The main disadvantage of adenoviral vectors lies in the activation of innate and adaptive parts of the patient’s immune system when the vector is applied in vivo. To overcome this potentially lethal complication, Lattermann and colleagues15 recently tested an adeno-associated viral vector (AAV) for its applicability to degenerative disc disease. The authors demonstrated that AAV was able to efficiently transduce human disc cells in vitro and rabbit disc cells in vivo. Although AAV caused a humoral immune response, no significant cellular immune response, as seen with adenoviral vectors, was observed. Interestingly, despite the observed humoral immune response, significant transgene expression was observed in the preexposed animals.15 These findings suggest that AAV might offer a valuable and safer alternative to adenoviral vectors in the future. Although the aforementioned studies suggest the feasibility of direct gene therapy using viral vectors to target disc cells, the question of the delivered gene and therefore expressed gene product remains open. Nishida and coworkers14 published one of the initial studies to deliver an exogenous therapeutic gene in vivo using an adenoviral vector carrying the gene for TGF-b1 to modify cells of rabbit IVD. The authors found significant increases of TGF-b1 and proteoglycan production in the injected disc, suggesting the feasibility of direct gene therapy to treat intervertebral disc degeneration.14 Lim Mineralization Protein (LMP) is another potentially beneficial gene factor that has been shown to positively affect the degenerated disc cellular matrix by increasing the disc cell production of Bone Morphogenetic Proteins (BMP) and proteoglycans in vitro.16 In vivo studies involving the intradiscal injection of rabbit discs resulted in increased expression of the anabolic cytokines BMP-2 and BMP-7 mRNA and also led to increased production of aggrecan mRNA.16 These data suggest that LMP-1 is also a beneficial factor that could be applied to gene therapeutics for disc degeneration. Sox9-on the other hand, does not affect the proteoglycan content of the disc cellular matrix. Sox9 is a gene transcription factor responsible for the synthesis of type II collagen, and its transfer into cells from degenerated human discs resulted in increased levels of type II collagen.17 Injection of a Sox9-carrying adenoviral vector into traumatized rabbit discs resulted in preservation of the histologic appearance seen in healthy discs, whereas the injured control discs displayed typically recognized degenerative changes.17 Therefore, not only increased proteoglycan production but also collagen type II production seems to be able to prevent disc degeneration in vivo, thereby offering multiple potentially therapeutic options. Although the increased production of a single gene product seems to result in the transformation of disc cells, a combination of related gene-producing factors may prove synergistic and more physiologic. In fact, Moon and coworkers18 have already reported that the combined transfer of Transforming growth factor Beta-1 (TGF-b1), Insulin like growth factor(IGF)-1 and BMP-2 revealed the aforementioned hypothetical synergistic effect of these factors on protein synthesis by demonstrating an amplification of protein synthesis.
An opposite approach that might be a potential alternative to the use of anabolic factors to induce disc cells into the production of matrix components is the application of anticatabolic factors. Inhibition of catabolic activity would ensue in maintaining or increasing the content of the respective matrix component by slowing down its degradation without the need to force the disc cells to higher synthesis rates. Wallach and colleagues19 recently published an in vitro study utilizing an adenoviral vector to introduce the gene encoding forTissue inhibitor of metalloproteinase (TIMP)-1 into disc cells isolated from degenerated human IVD. Gene delivery of TIMP-1 increased the proteoglycan content in the disc cell cultures, suggesting the anticatabolic approach to be a potentially promising strategy for gene therapy of degenerative disc disease.
The aforementioned index gene therapeutic approaches report results that sound promising. Despite the obvious potentials, the reality is that the application to human IVD will be challenged by the suboptimal and eventually toxic microenvironment inside the severely degenerated disc. It is questionable if the remaining compromised IVD cells in the degenerated disc will be able to produce reasonable amounts of gene-induced growth factors over extended periods of time. Furthermore, one can argue that it is unlikely that the existing starving cells are able to properly respond and produce an improved matrix even if the production of the respective gene product is achieved.
Autologous Implantation of Cultivated, Modified Cells
Degenerated discs could be treated by supplementation of the deserted matrix with in vitro cells that have been removed, cultivated, and modified. Autologous cells are optimal because their utilization makes the potential immunological complications a moot issue. Autologous cells compatible with disc tissue have to be harvested, expanded in vitro, and subsequently implanted into the symptomatic IVD. Once the cells have been removed and cultivated in vitro, this approach allows for indirect gene therapy via genetic modification of the withdrawn cells and/or tissue engineering via seeding of the cells in supporting biomaterials before implantation into the symptomatic degenerated IVD (see Figure 63-5). The combination of these techniques potentially improves efficiency by improving cell survival or enhancing the biosynthetic activity of the implanted cells. Genetic modification of cultivated cells in vitro is technically very similar to the aforementioned approaches previously discussed. The focus in this section turns to the cultivation of disc cells and creation of suitable implants.
For several reasons, it is extremely difficult to obtain suitable and sufficient numbers of target cells from intervertebral disc tissue. Removal of nucleus pulposus cells, the obvious target cells, would require opening of the annulus fibrosus to gain access. This would almost certainly cause damage to the annulus. In addition to the very restricted accessibility, the very low cell density in degenerated discs will further complicate the acquisition of ample usable cells for successful in vitro cultivation. Therefore only a limited number of scenarios are conceivable that would allow the withdrawal of sufficient cells from the disc to perform a disc cell–based approach without further damaging the already affected disc or accelerating its degeneration. Withdrawal of herniated disc material might be one scenario that would facilitate the removal of sufficient disc cells for in vitro cultivation. However, the introduction of cells/implants after a surgical intervention on the same disc could be disputed since the outcome of microdiscectomy has been shown to be satisfactory for most patients with radiculopathy. That being said, the direct insult as well as the likely accelerated degenerative process of the surgical level post microdiscectomy predisposes that segment to discogenic LBP postoperatively. This increased risk may justify cell implantation to prevent postoperative acute and/or chronic discogenic LBP. Another potential clinical application would be the use of cell-based approaches to prevent the accelerated degeneration of discs adjacent to an interbody fusion level. Mechanical stress at segmental levels adjacent to fusion procedures is a known biomechanical issue. This is known to result in accelerated rates of degenerative disc disease and consequently discogenic low back pain at segments juxtapositioned to the fusion level (Figure 63-8). The disc material removed during the fusion procedure could be used as a source of cells to treat the adjacent disc. However, this would imply an intervention at an asymptomatic nondegenerated disc that only has the potential to degenerate in the future and is therefore rather questionable. Thus, at this time, autologous disc cell transplantation is limited to a few clinical scenarios but has the potential to prove to be a powerful approach within these limitations.
The most direct approach to support a degenerated disc by autologous cells would be injecting a suspension of ex vivo proliferated disc cells.20 An approach to prepare autologous disc cells for subsequent transplantation into the degenerated disc is the cultivation of the disc cells in three-dimensional cultivation systems. Initial experiments by Maldonado and associates21 demonstrated that cultivation of disc cells in three-dimensional constructs conserved the native phenotype, as demonstrated by the synthesis of matrix components similar to those observed in native discs. Since then, a wide variety of techniques have been recently applied to supply disc cells with the desired three-dimensional contructs.22 Experimental strategies whose feasibility has been tested in vivo in animal experiments have also been undertaken. Gruber and colleagues,23 in a study utilizing a sand rat–based model, applied autologous disc cells, expanded in routine monolayer cultures and seeded into a three-dimensional scaffold, to a hollowed cavity created in an intervertebral disc. After up to 33 weeks, no giant cell response was observed and the cells showed an appropriate morphology. In addition, no abnormality in the cell-surrounding matrix was observed, suggesting appropriate survival of the implanted cells during the analyzed time period. From their data the authors concluded that autologous disc cell implantation can be successful, although technically challenging. However, because of the immediate implantation after the seeding of the scaffold, the disc cells do not have the time to synthesize appropriate amounts of matrix before encountering the adverse environment within the disc. Cultivation of the disc cells in a three-dimensional system before implantation might, therefore, improve the chances for survival in the hostile environment of the degenerated disc. Sato and coworkers24 evaluated this approach by utilizing a nonimmunogenic atelocollagen scaffold to seed and cultivate annulus fibrosus cells. This process demonstrated an increased ability to express type II collagen mRNA and deposited more type II collagen and proteoglycan compared to cells grown in monolayers. Atelocollagen scaffolds seeded with annulus fibrosus cells have been allografted into the lacunae of recipient rabbits after laser diskectomy of the nucleus pulposus. Implantation resulted in a significant prevention of the narrowing of the intervertebral disc space up to 12 weeks postoperative compared to the nucleotomized control animals. Histological analysis also showed that the allografted cells were viable, proliferated, and produced a hyaline-like matrix in the disc tissue of the recipients. Although the rabbit model does not appropriately simulate the mechanical forces experienced by implanted disc cell in human discs, it is conceivable that cells surrounded by their own matrix might withstand mechanical forces with more success. That being said, another problem not addressed by the presented studies is the acute shortage of nutrients experienced by the cells after implantation into the degenerated disc. Considering that the nutrient supply is hardly sufficient for the original disc cells, it is questionable if the additional cells will survive for the prolonged time span likely required to provide a sustained structural improvement of the disc.
Implantation of Mesenchymal Stem Cells
Adult mesenchymal stem cells (MSCs) are uncommitted pluripotent stem cells that are found in several tissues, such as skeletal muscle, bone marrow, synovial membranes, and the dermis.25,26 Mesenchymal stem cells are of high plasticity and have a high capacity of multilineage differentiation. Members of the BMP family of growth factors have been used thus far to induce differentiation of mesenchymal stem cells into chondrocytes.27 However, since BMPs are not exclusively inducing cartilage differentiation, its expression needs to be carefully timed and modulated to prevent the subsequent generation of bone structures. To overcome this problem, signal transduction and transcription factors, such as members of the Sox family as well as the Brachyury factor, that exclusively induce cartilage differentiation have been tested and demonstrated with encouraging results.28,29 It has been found that cocultivation of MSCs with disc cells might be sufficient to induce a disc cell–like phenotype in MSCs.30,31 Although these data originate from in vitro experiments, it might be conceivable that MSCs would also differentiate in vivo after injection into the disc. Besides the cocultivation with disc cells, cultivating of mesenchymal stem cells in three-dimensional cultivating systems appears to be sufficient to induce a nucleus pulposus–like phenotype.32 Implantation of collagen gel–embedded mesenchymal stem cells into artificially degenerated rabbit discs resulted in preserved nuclear and annular structures, prevention of proteoglycan decrease from the nucleus pulposus, and increased disc height.33,34 The implanted cells were shown to survive and express genetic markers typical for nucleus pulposus cells. Similar results were also found after injection of a bone-derived MSC suspension into rabbit discs and injection of gel embedded MSCs into rat coccygeal discs.35,36
1. Kirkaldy-Willis W.H., Wedge J.H., Yong-Hing K., et al. Pathology and pathogenesis of lumbar spondylosis and stenosis. Spine. 1978;3(4):319-328.
2. Gruber H.E., Hanley E.N.Jr. Analysis of aging and degeneration of the human intervertebral disc. Comparison of surgical specimens with normal controls. Spine. 1998;23:751-757.
3. Johnson W.E., Evans H., Menage J., Eisenstein S.M., El Haj A., Roberts S. Immunohistochemical detection of Schwann cells in innervated and vascularized human intervertebral discs. Spine. 2001;26:2550-2557.
4. Horner H.A., Urban J.P. 2001 Volvo Award Winner in basic science studies: effect of nutrient supply on the viability of cells from the nucleus pulposus of the intervertebral disc. Spine. 2001;26:2543-2549.
5. An H.S., Thonar E.J., Masuda K. Biological repair of intervertebral disc. Spine. 2003;28:S86-S92.
6. Masuda K., Imai Y., Okuma M., et al. Osteogenic protein-1 injection into a degenerated disc induces the restoration of disc height and structural changes in the rabbit anular puncture. Spine. 2006 Apr 1;31(7):742-754.
7. An H.S., Takegami K., Kamada H., Nguyen C.M., Thonar E.J., Singh K., Andersson G.B., Masuda K. Intradiscal administration of osteogenic protein-1 increases intervertebral disc height and proteoglycan content in the nucleus pulposus in normal adolescent rabbits. Spine. 2005;30:25-31. discussion 31–22
8. Kawakami M., Masumoto T., Hashizume H., et al. Osteogenic protein-1 inhibits degeneration and pain-related behavior induced by chronically compressed nucleus pulposus in the rat. Spine. 2005 Sep 1;30(17):1933-1939.
9. Chubinskaya S., Kawakami M., Rappoport L., et al. Anti-catabolic effect of OP-1 in chronically compressed intervertebral discs. J. Orthop. Res. Apr.. 2007;25(4):517-530.
10. Miyamoto K., Masuda K., Kim J.G., et al. Intradiscal injections of osteogenic protein-1 restore the viscoelastic properties of degenerated intervertebral discs. Spine J.. 2006 Nov-Dec;6(6):692-703.
11. Kawakami M., Hashizume H., Matsumoto T., et al. Safety of epidural administration of osteogenic protein-1: behavioral and macroscopic observation. Spine. 2007 Jun 1;32(13):1388-1393.
12. Klein R.G., Eek B.C., O’Neill C.W., et al. Biochemical injection treatment for discogenic low back pain: a pilot study. Spine J.. 2003;3(3):220-226.
13. Masuda K., Oegema T.R.Jr., An H.S. Growth factors and treatment of intervertbral disc degneration. Spine. 2004;29:2757-2769.
14. Nishida K., Gilbertson L.G., Robbins P.D., Evans C.H., Kang J.D. Potential applications of gene therapy to the treatment of intervertebral disc disorders. Clin. Orthop. Relat. Res.. 2000;379(Suppl):S234-S241.
15. Lattermann C., Oxner W.M., Xiao X., et al. The adeno associated viral vector as a strategy for intradiscal gene transfer in immune competent and pre-exposed rabbits. Spine. 2005;30:497-504.
16. Yoon S.T., Park J.S., Kim K.S., et al. LMP-1 upregulates intervertebral disc cell production of proteoglycans and BMPs in vitro and in vivo. Spine. 2004;29:2603-2611.
17. Paul R., Haydon R.C., Cheng H., Ishikawa A., Nenadovich N., Jiang W., Zhou L., Breyer B., Feng T., Gupta P., He T.C., Phillips F.M. Potential use of Sox9 gene therapy for intervertebral degenerative disc disease. Spine. 2003;28:755-763.
18. Moon S.H., Nishida K., Gilbertson L., et al. Biologic response of human intervertebral disc cell to gene therapy cocktail. San Francisco, CA: International Society for the study of the Lumber Spine (ISSLS); 2001.
19. Wallach C.J., Sobajima S., Watanabe Y., Kim J.S., Georgescu H.I., Robbins P., Gilbertson L.G., Kang J.D. Gene transfer of the catabolic inhibitor TIMP-1 increases measured proteoglycans in cells from degenerated human intervertebral discs. Spine. 2003;28:2331-2337.
20. Ganey T.M., Meisel H.J. A potential role for cell-based therapeutics in the treatment of intervertebral disc herniation. Eur. Spine J.. 2002;11(Suppl 2):S206-S214.
21. Maldonado B.A., Oegema T.R.Jr. Initial characterization of the metabolism of intervertebral disc cells encapsulated in microspheres. J. Orthop. Res.. 1992;10:677-690.
22. Gruber H.E., Hanley E.N.Jr. Recent advances in disc cell biology. Spine. 2003;28:186-193.
23. Gruber H.E., Johnson T.L., Leslie K., Ingram J.A., Martin D., Hoelscher G., Banks D., Phieffer L., Coldham G., Hanley E.N.Jr. Autologous intervertebral disc cell implantation: a model using Psammomys obesus, the sand rat. Spine. 2002;27:1626-1633.
24. Sato M., Asazuma T., Ishihara M., Kikuchi T., Kikuchi M., Fujikawa K. An experimental study of the regeneration of the intervertebral disc with an allograft of cultured annulus fibrosus cells using a tissue-engineering method. Spine. 2003;28:548-553.
25. Bianco P., Gehron Robey P. Marrow stromal stem cells. J. Clin. Invest.. 2000;105:1663-1668.
26. Bianco P., Riminucci M., Gronthos S., Robey P.G. Bone marrow stromal stem cells: nature, biology, and potential applications. Stem Cells. 2001;19:180-192.
27. Mason J.M., Breitbart A.S., Barcia M., Porti D., Pergolizzi R.G., Grande D.A. Cartilage and bone regeneration using gene-enhanced tissue engineering. Clin. Orthop. Relat. Res.. 2000;379(Suppl):S171-S178.
28. Akiyama H., Chaboissier M.C., Martin J.F., Schedl A., de Crombrugghe B. The transcription factor Sox9 has essential roles in successive steps of the chondrocyte differentiation pathway and is required for expression of Sox5 and Sox6. Genes. Dev.. 2002;16:2813-2828.
29. Hoffmann A., Czichos S., Kaps C., Bachner D., Mayer H., Kurkalli B.G., Zilberman Y., Turgeman G., Pelled G., Gross G., Gazit D. The T-box transcription factor Brachyury mediates cartilage development in mesenchymal stem cell line C3H10T1/2. J. Cell Sci.. 2002;115:769-781.
30. Kim S., Le Visage C., Tateno K., Sieber A., Kostuik J., Leong K. Interaction of human mesenchymal stem cells with disc cells: changes in biosynthesis of extracellular matrix. Spine J.. 2002;2:107S.
31. Richardson S.M., Walker R.V., Parker S., Rhodes N.P., Hunt J.A., Freemont A.J., Hoyland J.A. Intervertebral disc cell mediated mesenchymal stem cell differentiation. Stem Cells. 2005;24:707-716.
32. Risbud M.V., Izzo M.W., Adams Cs, et al., Mesenchymal stem cells respond to their microenvironment in vitro to assume nucleus pulposus-like phenotype, 30th Annual meeting: International Society for the Study of the Lumber Spine (ISSLS), vancourer, canada, 2003
33. Sakai D., Mochida J., Iwashina T., Hiyama A., Omi H., Imai M., Nakai T., Ando K., Hotta T. Regenerative effects of transplanting mesenchymal stem cells embedded in atelocollagen to the degenerated intervertebral disc. Biomaterials. 2006;27:335-345.
34. Sakai D., Mochida J., Iwashina T., Watanabe T., Nakai T., Ando K., Hotta T. Differentiation of mesenchymal stem cells transplanted to a rabbit degenerative disc model: potential and limitations for stem cell therapy in disc regeneration. Spine. 2005;30:2379-2387.
35. Crevensten G., Walsh A.J., Ananthakrishnan D., et al. Intervertbral disc cell therapy for regeneration: mesenchymal stem cell implantation in rat intervertebral discs. Ann. Biomed. Eng.. 2004;32:430-434.
36. Zhang Y.G., Guo X., Xu P., et al. Bone mesenchymal stem cells transplanted into rabbit intervertebral discs can increase proteoglycans. Clin. Orthop. Relat. Res.. 2005;430:219-226.