CHAPTER 60 Intracranial Monitoring
History
The contribution of Otto von Guericke and later Ewald von Kleist and Pieter van Musschenbroek of Leyden in the late 1600s and early 1700s provided scientists with the means to generate and discharge static electricity. Although abundant hypotheses existed regarding the possible role of electricity in nerve and muscle conduction, Luigi Galvani was among the earliest scientists to demonstrate the role of, as he termed it, “animal electricity” in his experiments with frog leg preparations. Using electrostatic machines and static electricity generated from storms, he demonstrated contraction of muscle in response to the discharge of electricity and published these results in 1791. Galvani believed that these contractions resulted from the discharge of electricity from within the preparation; fortunately, not all agreed. Volta argued that intrinsic electricity was responsible, having been conducted into the tissue, possibly through the nerves. In the early 1800s, Hans Christian Oersted and J. S. C. Schweigger developed devices to measure small amounts of electricity (galvanometers). The development of such sensitive instruments allowed Richard Caton to record “feeble currents of the brain” directly from the cerebral cortex of animals in 1875.1 Fifty-four years later, Hans Berger is credited with being the first to describe the human EEG. His initial measurements were performed on patients with skull defects or trephine holes and later on intact patients. In 1931, he recorded spike wave activity from the brain of a person with epilepsy. Similarly, in 1935, Frederic and Erna Gibbs recorded comparable spike wave patterns with a frequency of 3 Hz from the scalp of a woman suffering from petit mal seizures. In 1929, Sachs, Schwartz, and Kerr first recorded such activity from the surface of the human brain. Victor Horsely had been using direct cortical stimulation to guide resections for epilepsy, but it was Wilder Penfield and Herbert H. Jasper who began recording abnormal electrical activity directly from the surface of the brain at the time of such surgery. The first use of stereotactic depth electrodes for the treatment of intractable seizures dates to 1950, when E. A. Spiegel and H. T. Wycis recorded from and subsequently lesioned the lateral thalamus in an attempt to relieve seizures. These and other early studies emphasized the use of interictal recordings to guide resections. Talairach and Bancaud realized the limited ability of interictal activity to delineate the areas of paroxysmal ictal discharge,2 and under this influence the American neurosurgeon Paul H. Crandall began chronic monitoring for the recording of spontaneous seizures in 1973.
Indications
Epilepsy
In a typical phased epilepsy surgery evaluation, patients undergo detailed history taking and physical examination, followed by MRI and an outpatient EEG. This is usually followed by an inpatient continuous audiovisual EEG to further document interictal patterns on the EEG and to capture detailed semiologic (semiology, or the physical and experiential manifestation of a seizure) data, along with the ictal patterns on the EEG. During this time, patients may also undergo interictal and ictal SPECT and interictal PET. Neuropsychological examination may take place at this time as well. At the end of this period of monitoring, all the data are examined in detail to assess whether a specific area is responsible for the seizures. A decision tree is presented in Figures 60-1 and 60-2. Each part of the preoperative data set has its own characteristics that must be weighed in making the final decision with regard to concordance. Some data, by their very nature, point to only broad areas of brain dysfunction, whereas others may be very specific. Other pieces of data, such as an ictal scalp EEG, may be more regional at best and confer more weight to an MRI-detected structural abnormality, or the ictal EEG may indicate multifocality or generalization or be characterized only by muscle artifact.
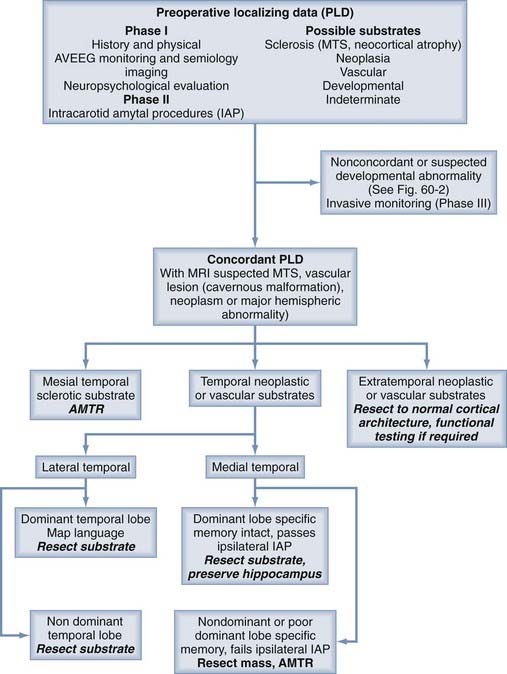
FIGURE 60-1 Part 1 of a decision-making tree for the evaluation of patients with intractable epilepsy.
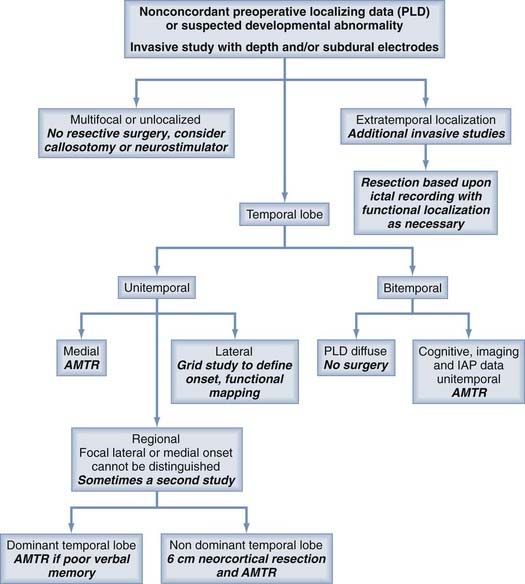
FIGURE 60-2 Part 2 of a decision-making tree for the evaluation of patients with intractable epilepsy.
A special case involves patients with lesions and evidence of hippocampal sclerosis. There is speculation in the literature about the relationship of these entities.3–5 It is possible that an extrahippocampal lesion may cause seizures that spread through the hippocampus. Over time, this spread pattern could damage the hippocampus through excitotoxicity and render it an independent source of seizures. At our institution, in general, patients with hippocampal atrophy and dysfunction (temporal lobe–specific poor memory) in whom the other data are concordant for the temporal lobe undergo combined resection of the lesion and the hippocampus. The more medial the lesion, the more likely we are to assume true dual pathology and resect both. Patients without evidence of hippocampal dysfunction on neuropsychological studies and the intracarotid amobarbital (Amytal) procedure (IAP) undergo lesionectomy without hippocampectomy, regardless of the volume of the hippocampus, although a small proportion of these patients may need further resection. In the context of these cases, the IAP (Wada test) is also specifically used to ensure the ability of the contralateral hemisphere to encode memory when hippocampal resection is planned. Poor memory performance of the contralateral hemisphere on the IAP would largely be a contraindication to resection and would necessitate consideration of nonresective strategies for treatment of the patient’s seizure disorder, such as investigational responsive electrical neurostimulation or multiple subpial transections, when applicable.
Brain Mapping
A separate indication for intracranial monitoring of patients without epilepsy is for extraoperative brain mapping (see Fig. 60-8). The goal is typically to map the areas of appreciable function in relation to a lesion (1) to provide data about the risks associated with surgical resection so that the clinician and patient can make a decision about the appropriateness of surgery and (2) to allow surgical planning to minimize those risks. In some instances, intraoperative monitoring may be the most efficient way to address these issues. Mapping of simple motor modalities can be accomplished at surgery through direct cortical stimulation. Similarly, sensory mapping can be performed via somatosensory evoked potentials. Intraoperative language mapping requires an awake patient who can participate at the time of surgery and demonstrate reversible deficits during cortical stimulation. Testing of higher cognitive function may be difficult during surgery because of the complexity of the tasks that must be performed. Beyond these straightforward tests, particular patients and particular types of mapping may make extraoperative mapping more desirable.
Hardware
A variety of electrodes are available for use in electrophysiologic monitoring, and current research is focused on developing wireless implantable systems. A combination of depth electrodes and subdural strip or grid electrodes are routinely used in most situations (Fig. 60-3). In certain situations in which the plane between the dura and brain surface is scarred and safe dissection is impossible, epidural peg electrodes can be used. Alternatively, a subdural electrode can be placed in the epidural space, but unless the dura has been denervated, these electrodes cannot be used for stimulation-based mapping. Some centers supplement scalp studies or intracranial studies with foramen ovale or sphenoidal electrodes. The construction, surgical technique, and use of each are discussed here.
Depth Electrodes
Depth electrodes are typically constructed with one or more contacts in a thin shaft with a blunt tip to minimize traumatic brain and vascular injury. Both rigid and flexible types exist. For insertion, the flexible electrodes are made rigid through a stylet that is fixed adjacent to the electrode or placed down the center and removed after insertion. An added advantage is that these electrodes can be tunneled subcutaneously, thereby reducing the risk for infection. The contacts themselves are usually made of platinum or platinum-iridium construction and spaced from 3 to 10 mm apart. For multicontact electrodes, individual insulated wires run up the inner cannula of the hollow electrode and lead to contacts that can be connected to a ribbon cable. Electrodes with multiple contacts can be used to record along the longitudinal dimension of a structure, such as the hippocampus, or they may allow simultaneous recording of a deep structure and the cortical surface through which the electrode was inserted (Figs. 60-4 and 60-5).
Subdural Electrodes—Strips and Grids
Most grids are similarly constructed, but they have columns of multiple contacts for a wider area of coverage (see Fig. 60-4). These grids terminate in multiple thin, hollow tubes with contacts for connecting to ribbon cables. One special type is an L-shaped grid for recording from the medial surface of the hemisphere (Fig. 60-6). Once again, the contacts may be made of platinum or stainless steel. The platinum construction allows safe postoperative performance of MRI.6
Epidural Peg Electrodes
Recording of electrical activity from electrodes placed in the epidural space traces its history to Penfield and Jasper, who used epidural ball electrodes in a few difficult cases.7 In comparison to scalp EEGs, epidural recordings have the advantage of providing an improved signal-to-noise ratio. The effect is created by reducing volume conduction and amplitude attenuation and by eliminating the myogenic and kinesigenic artifacts inherent with scalp EEGs.8 Epidural recording has also been considered an alternative to subdural and intraparenchymal monitoring because of its semi-invasive nature and the presumed advantage of fewer infectious and hemorrhagic complications.
Epidural electrode designs have included ball electrodes,7 screws,8 and pegs.9 Epidural screws and peg electrodes are widely applied in some epilepsy surgery centers. The screw is usually made of titanium and has a shaft to prevent overpenetration. Screw length varies to allow stable placement and to accommodate the varying thickness of the scalp and calvaria. The screw head is hexagonal to permit easy placement and removal of the electrodes with a wrench. Right-angled EEG monitoring leads can be placed in the screw head.8 Epidural peg electrodes are composed of mushroom-shaped Silastic elastomer, and the stalk tapers from a diameter of 4.7 mm to a diameter of 0.5 mm. At the base of the stalk, either stainless steel or platinum tips are used to conduct the electrical current. The tips are continuous with the Teflon-coated steel wire that is tunneled through the peg and exits through the cap. Strip arrays of epidural electrodes have also been described.10
Foramen Ovale and Sphenoidal Electrodes
Foramen ovale electrodes were first developed in 1985 as a semi-invasive EEG alternative to intracerebral depth electrodes for the evaluation of mesial temporal lobe epilepsy.11 These electrodes generally consist of helical, wound, Teflon-coated silver wires that end in multicontact poles.12 The construct is mounted on a thin stainless steel wire. The mechanical properties conferred to this construct allow appropriate flexibility to avoid puncturing the pia-arachnoid layer. The external diameter permits easy passage through a specially constructed 18-gauge introducer cannula.11
Sphenoidal electrodes are used by many epilepsy centers as an adjunct to standard scalp EEG electrodes for the evaluation of temporal lobe epilepsy. These electrodes, in conjunction with scalp EEGs, can help determine whether the focus of the seizure is in the medial or lateral aspect of the temporal lobe. Sphenoidal electrodes were originally rigid, thus allowing only short-term monitoring, but they have evolved to a flexible design. Teflon-coated or multiple wires have silver or stainless steel tips, are flexible, and may be applied for EEG recordings for up to 3 weeks.13
Recording Equipment
After implantation, the leads are connected to an isolation box to prevent any inadvertent current from entering the patient. Impedances are checked with a small current in the vicinity of 10 nA.14 The isolation box is then connected to a multichannel amplifier and a recording system. Typically, systems with at least 64-channel capabilities are used; however, more extensive studies necessitate more channels. An initial montage with sampling from the contacts of each implanted device is used. The information gained as the study continues usually dictates changes in the montage to focus on the relevant areas. Simultaneous video recordings provide the information necessary to correlate semiology with electrophysiologic data.
Design of Studies
Epilepsy Studies
Several scenarios based on the type and multiplicity of lesions and the reliability or concordance of the preoperative data are typically encountered during the study design. Patients with lesions and discordant data generally require grid coverage over the lesion, as well as strip and depth electrode coverage of areas as indicated by the other preoperative data. Patients with developmental abnormalities merit special consideration. In contrast to most other lesions, these malformative lesions may be inherently capable of seizure generation. Subdural grid coverage may not identify the seizure source if it is located in a malformed area of gray matter; therefore, depth electrode coverage of the lesion itself may be required. Other less accessible lesions may be suitable for depth electrodes, such as the medial hemisphere, orbitofrontal area, or the insula (see Figs. 60-4 and 60-5). Patients with multiple lesions typically require coverage over the lesions most likely to be responsible for seizure generation, as well as other potential epileptogenic regions demonstrated on preoperative studies. In patients with suspected bilateral medial temporal lobe seizures, bilateral hippocampal electrodes are used in combination with bilateral temporal lobe strip coverage (Fig. 60-7; also see Fig. 60-4A and B).
Brain Mapping Studies
Typically, these focused studies cover the areas of the lesion, as well as adjacent functional brain. In most locations, a subdural grid is well suited to such studies (Fig. 60-8). In other cases, regional venous anatomy may dictate the use of custom-cut grids or subdural strips to avoid sacrificing a critical vein. For example, with lesions in the posterior fusiform gyrus, the location of the vein of Labbé may make grid placement difficult.
Operative Considerations
Subdural Strips
Before preparation, the skin incision should be marked. Localization can be performed with simple craniometric measurement in conjunction with MRI, or stereotaxy can be used as an aid. Once again, the possible need to incorporate incisions into a future larger incision for resection should be considered. For example, in patients undergoing temporal lobe strip studies in which anteromedial temporal lobe resection may be required, we typically make the skin incision for insertion of the subdural strip in the same location as the upper limb of the temporal lobe craniotomy incision (see Fig. 60-7). After the skin incision, a small craniotomy is made, or a bony trough is created in the anteroposterior direction with multiple bur holes that are connected via a cutting bur. After removal of the bone, the dura is coagulated and opened. The strips are inserted with a pair of forceps while directing them toward the desired target with a steady stream of irrigation under the strip. This irrigation helps the strip slide smoothly over the surface of the brain and prevents trauma. If any resistance is felt, the strip is removed and reinserted. These areas of resistance may represent bridging cortical veins and thus should be avoided to prevent hemorrhage under the bone, to which the surgeon has no access through the same craniotomy. Finding the correct trajectory and depth of insertion for a given cortical location requires both patience and experience. After insertion, the intended location is recorded in the patient’s chart, and the electrode is tunneled through the skin. A purse-string suture is placed around the site of exit, and the electrode is secured with a separate skin suture. Gelfoam is placed over the dural incision, and the skin is closed.
One particular technique of note is placement of a medial temporal subdural strip. Typically, a 12-contact strip is used, and the strip is inserted parallel to the sylvian fissure by gently pushing in a posterior to anterior direction, just below the sphenoid ridge. This strip will guide itself along the sphenoid and eventually place contacts close to the parahippocampal gyrus (see Fig. 60-4D).
Subdural Grids
Placement of large subdural grids requires a craniotomy flap of the same approximate size as the grid (see Fig. 60-4C). Once again, the skin flap should be designed so that if necessary, it can be incorporated into an incision for resection. The skin is incised in a U-shaped fashion and reflected. A periosteal graft may be harvested for use in dural closure after grid placement if desired. The bone flap is removed and the bone is cleaned thoroughly and sent to the bone bank for the duration of the study. Alternatively, an incision can be made in the abdomen and the bone inserted subcutaneously for safekeeping until the time of replacement, or it can be left in the craniotomy site but not secured tightly to the skull. After hemostasis, the dura is opened and removed in a circumferential fashion. This denervates the dura and decreases the frequency of postoperative headaches. A digital photograph of the brain is then taken. The grid is placed on the brain in the appropriate position and the multiple leads are tunneled subcutaneously and brought through the base of the skin flap, if possible. Purse-string sutures are placed around the exit of each site, and each electrode is secured to the skin with a separate suture. An additional photograph is taken with the grid, strip, and depth electrodes in place. These photographs often reveal gyral anatomy and electrode placement that cannot be achieved even with three-dimensional reconstructed MRI scans. The dura can then be closed with the previously harvested periosteal and dural grafts. Alternatively, we have been using a Gore-Tex dural substitute (Gore Preclude MVP Dura Substitute). Use of this particular product has drastically reduced scarring at the brain surface and facilitates dissection at the time of resection. An epidural drain is placed and tunneled through a distant separate stab incision. A “sleeper stitch” is placed around the drain exit site and wrapped around the drain. This stitch is used to secure the skin when the drain is removed the following morning. The skin is closed in two layers.
Foramen Ovale and Sphenoidal Electrodes
Härtel’s landmarks15 are used to insert foramen ovale and sphenoidal electrodes. The introductory cannula is inserted 3 cm lateral to the oral commissure and passed to the foramen ovale via Kirschner’s technique under fluoroscopic guidance. The cannula is guided along a line formed by the intersection of two orthogonal planes. The first plane is defined by the insertion point and the point on the lower eyelid corresponding to the medial border of the pupil. The second plane is defined by the insertion point and a spot 5 cm anterior to the external auditory meatus. Return of cerebrospinal fluid is visualized with removal of the inner cannula. The electrode is then placed through the cannula, usually without resistance, until its expected placement in the cistern. The cannula is then withdrawn, and the electrodes are fixed to the skin with gauze and adhesive tape. Removal of foramen ovale electrodes does not require anesthesia. Transient spasm or dysesthesias in the ipsilateral teeth may be elicited during withdrawal of the electrodes.
Postoperative Care
The head is wrapped in a bulky dressing with a chin strap and the electrodes exiting from one or two sites. This type of dressing is necessary to prevent dislodgement of the electrodes during seizures and to contain any minor cerebrospinal fluid leaks. Patients who undergo grid placement usually receive a 1- to 3-day tapering course of methylprednisolone (Solu-Medrol). MRI and a high-resolution tomographic scan are performed the following morning to localize the electrodes. Before sending the patient for an MRI, care is taken to ensure that none of the electrodes form a loop. The electrode contacts are well visualized on computed tomographic scans; however, the signal artifact makes it difficult to delineate postoperative complications.6 In addition, the superior resolution of MRI provides anatomic detail that is not possible to obtain with computed tomography. Therefore, we digitally fuse the MRI and computed tomographic images to create three-dimensional renderings of the electrodes and the brain (http://www.bioimagesuite.org/). This allows us to better understand the locations of the electrodes relative to the potential epileptogenic substrate. Interictal SPECT may be performed at this time for later substraction from an ictal injection.
Complications include small intraparenchymal hematomas associated with depth electrodes (2%), subdural electrodes mistakenly placed intraparenchymally (4%),16 and placement of depth electrodes in the wrong position (2%).17
After the imaging studies are completed, the patient is taken to the epilepsy unit and connected to the monitoring equipment. The exiting electrodes and their connections must be tethered to the dressing. Wyler and colleagues found no difference in rates of meningitis in patient receiving continuous antibiotics after subdural strip placement versus those receiving only perioperative coverage18; thus, our patients routinely receive a 24-hour course of antibiotics and then no antibiotics during the remainder of the study unless other indications arise. Cerebrospinal fluid leaks may occur in up to 19% of patients,19 so the head dressing is checked twice daily for evidence of leakage. A wet dressing is changed with sterile technique, and the source of the leak is sought and sutured. Patients usually experience low-grade fever and headache after the procedure. These fevers do not correlate with intracranial infection,20 but care should be taken to evaluate patients with prolonged fever. Headache is especially troublesome and patients may require narcotics for 36 to 48 hours.
Outcome with Intracranial Monitoring
Approximately 64% to 94% of patients are found to have sufficient localizing information to proceed to resection after intracranial study.17,21–23 Thus, many more patients can be offered resection than those evaluated with scalp EEGs only.24 An increasing percentage of patients being monitored are those with extratemporal epilepsy because MRI identifies most lesions and hippocampal atrophy.
After resection, 28% to 64% of patients are seizure free.17,23,25 The range is so wide because series from different centers have substantially varied patient populations, experience, and indications for intracranial monitoring. In 21 patients with seizures localizing to the temporal lobe, Cascino and coworkers found that only 9 were seizure free after resection.26 The largest number of patients with inferior outcomes had normal findings on MRI (without hippocampal atrophy), along with 3 patients who had hypothalamic masses and were thought to have gelastic seizures.
Epidural peg and screw electrodes have limited applications in the presurgical evaluation of epilepsy patients and have been used in addition to standard seizure monitoring techniques.27,28 Their main advantages are the relative ease of placement and the probably decreased rates of major complications associated with subdural and intraparenchymal monitoring. Even though the signal-to-noise ratio of epidural monitoring is significantly improved over that of scalp EEG, it provides a lower density of coverage than possible with subdural or intraparenchymal monitoring and does not allow the definition of functional anatomy. The issues regarding the density of coverage may be overcome in part by the placement of epidural grid electrodes, which requires a craniotomy.
Surgical outcome regarding the preoperative use of epidural arrays was reported by Goldring and Gregorie, who reviewed 100 cases in which epidural electrode arrays were used to define the epileptic focus.10 Sixty-four percent of the epileptic children and 62% of the epileptic adults had “good” outcomes. Kuzniecky and coauthors also reported greater than 90% improvement in seizures after resection based on the use of epidural grids and strips in 50 patients with predominantly temporal lobe epilepsy.29 Epidural electrodes can identify epileptogenic foci in select patients with temporal lobe epilepsy and poorly localizing scalp EEG recordings.30 Nevertheless, questions remain about whether surgical outcomes based on the use of epidural arrays are comparable to those of resections based on more invasive monitoring.
The efficacy of foramen ovale electrodes in identifying the mesolimbic temporal lobe as the epileptogenic substrate has been examined on a limited basis. However, in scenarios in which clinical, imaging, and scalp EEG data do not give clear temporal lateralizing information, there may be a safe and valuable adjunct.20,31 With careful analysis, foramen ovale electrode recordings have been used to reliably identify the primary temporal source of seizures.32 When candidates for amygdalohippocampectomy were studied preoperatively with foramen ovale electrodes, favorable outcomes were obtained by Wieser and associates11; similar outcomes were reported in that group (64% seizure free) and in the group studied with stereoelectroencephalography (59% seizure free). Similarly, Shih and colleagues demonstrated that 78% of patients with mesial temporal lobe epilepsy became seizure free after resection based on the use of foramen ovale electrodes.31
The advantages of foramen ovale electrodes are their semi-invasiveness, relative ease of placement, and elimination of the increased risk associated with the placement of depth electrodes and subdural grids and strips.33 They may also be more cost-effective.34 One drawback involves interpretation of the information generated without the appropriate context of having accurate electrographic information from other alternative epileptogenic sites. The most serious drawback may be the possibility of falsely localizing the seizure focus to the mesial temporal lobe and missing the real epileptogenic focus, which may be in the neocortical temporal lobe or in an extratemporal location. Some centers with extensive experience in the technique emphasize the need to closely examine the seizure discharge pattern, as well as the scalp EEG, when interpreting the results recorded from foramen ovale electrodes. In light of the theoretical disadvantage of false localization, foramen ovale electrodes have not been used at our institution. We favor the techniques of intracranial depth electrodes and subdural grids and strips, which remain the “gold standard” in this field. Rather than replacing invasive monitoring, foramen ovale electrodes may have a more rational place as an adjunct, perhaps in the selection of patients for further invasive electrographic localization.
Early studies comparing sphenoidal electrodes with scalp EEG electrodes revealed that in patients suspected of having mesial temporal lobe epilepsy, the spike amplitude was largest with sphenoidal electrodes. It was believed that the proximity of these electrodes to the basal medial aspect of the temporal lobe increased their sensitivity for detecting mesial temporal discharges.35 This notion of sphenoidal spikes’ specificity for mesial temporal discharges has been questioned, however; Marks and coworkers found that the large sphenoidal spikes may be associated with extratemporal and extrahippocampal foci, thus raising questions regarding the specificity of sphenoidal electrodes.36 Historically, many sphenoidal electrodes were passed blindly, which might have compromised the accuracy of the EEG recordings. When compared with the blind approach, Kanner and coauthors reported markedly improved sensitivity and specificity when sphenoidal electrodes were placed under fluoroscopic guidance.37 Furthermore, when combined with scalp EEG, sphenoidal electrodes can be used to distinguish medial and neocortical epilepsy, with localization of medial temporal lobe ictal foci in 90% of patients.38
Awad IA, Assirati JAJr, Burgess R, et al. A new class of electrodes of ‘intermediate invasiveness’: preliminary experience with epidural pegs and foramen ovale electrodes in the mapping of seizure foci. Neurol Res. 1991;13:177-183.
Barnett GH, Burgess RC, Awad IA, et al. Epidural peg electrodes for the presurgical evaluation of intractable epilepsy. Neurosurgery. 1990;27:113-115.
Baumgartner C, Lindinger G, Lurger S, et al. Prolonged video EEG monitoring in differential diagnosis of seizures and in presurgical epilepsy diagnosis. Wien Med Wochenschr. 1998;148:2-8.
Behrens E, Zentner J, van Roost D, et al. Subdural and depth electrodes in the presurgical evaluation of epilepsy. Acta Neurochir (Wien). 1994;128:84-87.
Brazier MAB. A History of the Electrical Activity of the Brain: The First Half-Century. New York: Macmillan; 1961.
Carter DA, Lassiter AT, Brown JA. Cost-efficient localization of seizures of mesiotemporal onset with foramen-ovale electrodes. Neurol Res. 1998;20:153-160.
Cascino GD, Jack CRJr, Parisi JE, et al. Operative strategy in patients with MRI-identified dual pathology and temporal lobe epilepsy. Epilepsy Res. 1993;14:175-182.
Cascino GD, Trenerry MR, Sharbrough FW, et al. Depth electrode studies in temporal lobe epilepsy: relation to quantitative magnetic resonance imaging and operative outcome. Epilepsia. 1995;36:230-235.
Cendes F, Cook MJ, Watson C, et al. Frequency and characteristics of dual pathology in patients with lesional epilepsy. Neurology. 1995;45:2058-2064.
Drury I, Schuh L, Ross D, et al. Ictal patterns in temporal lobe epilepsy recorded by epidural screw electrodes. Electroencephalogr Clin Neurophysiol. 1997;102:167-174.
Goldring S, Gregorie EM. Surgical management of epilepsy using epidural recordings to localize the seizure focus. Review of 100 cases. J Neurosurg. 1984;60:457-466.
Härtel F. Über die intracranielle Injecktionbehandlung der Trigeminusneuralgie. Med Klin. 1914;10:582-584.
Kanner AM, Parra J, Gil-Nagel A, et al. The localizing yield of sphenoidal and anterior temporal electrodes in ictal recordings: a comparison study. Epilepsia. 2002;43:1189-1196.
Kanner AM, Ramirez L, Jones JC. The utility of placing sphenoidal electrodes under the foramen ovale with fluoroscopic guidance. J Clin Neurophysiol. 1995;12:72-81.
Kim OJ, Ahn JY, Lee BI. Analysis of electrical discharges made with the foramen ovale electrode recording technique in mesial temporal lobe epilepsy patients. J Clin Neurophysiol. 2004;21:391-398.
Kuzniecky R, Faught E, Morawetz R. Electroencephalographic correlations of extracranial and epidural electrodes in temporal lobe epilepsy. Epilepsia. 1991;32:335-340.
Kuzniecky R, Faught E, Morawetz R. Surgical treatment of epilepsy: initial results based upon epidural electroencephalographic recordings. South Med J. 1990;83:637-639.
Li LM, Cendes F, Watson C, et al. Surgical treatment of patients with single and dual pathology: relevance of lesion and of hippocampal atrophy to seizure outcome. Neurology. 1997;48:437-444.
Marks DA, Katz A, Booke J, et al. Comparison and correlation of surface and sphenoidal electrodes with simultaneous intracranial recording: An interictal study. Electroencephalogr Clin Neurophysiol. 1992;82:23-29.
Nakase H, Tamura K, Kim YJ, et al. Long-term follow-up outcome after surgical treatment for lesional temporal lobe epilepsy. Neurol Res. 2007;29:588-593.
Pacia SV, Ebersole JS. Intracranial EEG substrates of scalp ictal patterns from temporal lobe foci. Epilepsia. 1997;38:642-654.
Penfield W, Jasper HH, editors. Epilepsy and the Functional Anatomy of the Human Brain. Boston: Little, Brown, 1954.
Rosenbaum TJ, Laxer KD, Vessely M, et al. Subdural electrodes for seizure focus localization. Neurosurgery. 1986;19:73-81.
Ross DA, Brunberg JA, Drury I, et al. Intracerebral depth electrode monitoring in partial epilepsy: the morbidity and efficacy of placement using magnetic resonance image–guided stereotactic surgery. Neurosurgery. 1996;39:327-333.
Ross DA, Henry TR, Dickinson LD. A percutaneous epidural screw electrode for intracranial electroencephalogram recordings. Neurosurgery. 1993;33:332-334.
Shih YH, Yiu CH, Huang CI. Role of foramen ovale electrodes in presurgical evaluation of intractable complex partial seizures. Zhonghua Yi Xue Za Zhi (Taipei). 1997;60:155-160.
Silberbusch MA, Rothman MI, Bergey GK, et al. Subdural grid implantation for intracranial EEG recording: CT and MR appearance. AJNR Am J Neuroradiol. 1998;19:1089-1093.
Spencer SS. Depth electroencephalography in selection of refractory epilepsy for surgery. Ann Neurol. 1981;9:207-214.
Spencer SS, Spencer DD, editors. Surgery for Epilepsy. Boston: Blackwell Scientific, 1991.
Spencer SS, Spencer DD, Williamson PD, et al. Combined depth and subdural electrode investigation in uncontrolled epilepsy. Neurology. 1990;40:74-79.
Swartz BE, Rich JR, Dwan PS, et al. The safety and efficacy of chronically implanted subdural electrodes: a prospective study. Surg Neurol. 1996;46:87-93.
Talairach J, Bancaud J. Lesion, “irritative” zone and epileptogenic focus. Confin Neurol. 1966;27:91-94.
Velasco TR, Sakamoto AC, Alexandre VJr, et al. Foramen ovale electrodes can identify a focal seizure onset when surface EEG fails in mesial temporal lobe epilepsy. Epilepsia. 2006;47:1300-1307.
Wieser HG, Elger CE, Stodieck SR. The ‘foramen ovale electrode’: a new recording method for the preoperative evaluation of patients suffering from mesio-basal temporal lobe epilepsy. Electroencephalogr Clin Neurophysiol. 1985;61:314-322.
Wieser HG, Siegel AM. Analysis of foramen ovale electrode–recorded seizures and correlation with outcome following amygdalohippocampectomy. Epilepsia. 1991;32:838-850.
Wilkus RJ, Thompson PM. Sphenoidal electrode positions and basal EEG during long term monitoring. Epilepsia. 1985;26:137-142.
Wyler AR, Ojemann GA, Lettich E, et al. Subdural strip electrodes for localizing epileptogenic foci. J Neurosurg. 1984;60:1195-1200.
Wyler AR, Walker G, Somes G. The morbidity of long-term seizure monitoring using subdural strip electrodes. J Neurosurg. 1991;74:734-737.
1 Brazier MAB. A History of the Electrical Activity of the Brain: The First Half-Century. New York: Macmillan; 1961.
2 Talairach J, Bancaud J. Lesion, “irritative” zone and epileptogenic focus. Confin Neurol. 1966;27:91-94.
3 Cascino GD, Jack CRJr, Parisi JE, et al. Operative strategy in patients with MRI-identified dual pathology and temporal lobe epilepsy. Epilepsy Res. 1993;14:175-182.
4 Cendes F, Cook MJ, Watson C, et al. Frequency and characteristics of dual pathology in patients with lesional epilepsy. Neurology. 1995;45:2058-2064.
5 Li LM, Cendes F, Watson C, et al. Surgical treatment of patients with single and dual pathology: relevance of lesion and of hippocampal atrophy to seizure outcome. Neurology. 1997;48:437-444.
6 Silberbusch MA, Rothman MI, Bergey GK, et al. Subdural grid implantation for intracranial EEG recording: CT and MR appearance. AJNR Am J Neuroradiol. 1998;19:1089-1093.
7 Penfield W, Jasper HH, editors. Epilepsy and the Functional Anatomy of the Human Brain. Boston: Little, Brown, 1954.
8 Ross DA, Henry TR, Dickinson LD. A percutaneous epidural screw electrode for intracranial electroencephalogram recordings. Neurosurgery. 1993;33:332-334.
9 Barnett GH, Burgess RC, Awad IA, et al. Epidural peg electrodes for the presurgical evaluation of intractable epilepsy. Neurosurgery. 1990;27:113-115.
10 Goldring S, Gregorie EM. Surgical management of epilepsy using epidural recordings to localize the seizure focus. Review of 100 cases. J Neurosurg. 1984;60:457-466.
11 Wieser HG, Siegel AM. Analysis of foramen ovale electrode–recorded seizures and correlation with outcome following amygdalohippocampectomy. Epilepsia. 1991;32:838-850.
12 Wieser HG, Elger CE, Stodieck SR. The ‘foramen ovale electrode’: a new recording method for the preoperative evaluation of patients suffering from mesio-basal temporal lobe epilepsy. Electroencephalogr Clin Neurophysiol. 1985;61:314-322.
13 Wilkus RJ, Thompson PM. Sphenoidal electrode positions and basal EEG during long term monitoring. Epilepsia. 1985;26:137-142.
14 Spencer SS, Spencer DD, editors. Surgery for Epilepsy. Boston: Blackwell Scientific, 1991.
15 Härtel F. Über die intracranielle Injecktionbehandlung der Trigeminusneuralgie. Med Klin. 1914;10:582-584.
16 Ross DA, Brunberg JA, Drury I, et al. Intracerebral depth electrode monitoring in partial epilepsy: the morbidity and efficacy of placement using magnetic resonance image–guided stereotactic surgery. Neurosurgery. 1996;39:327-333.
17 Rosenbaum TJ, Laxer KD, Vessely M, et al. Subdural electrodes for seizure focus localization. Neurosurgery. 1986;19:73-81.
18 Wyler AR, Walker G, Somes G. The morbidity of long-term seizure monitoring using subdural strip electrodes. J Neurosurg. 1991;74:734-737.
19 Velasco TR, Sakamoto AC, Alexandre VJr, et al. Foramen ovale electrodes can identify a focal seizure onset when surface EEG fails in mesial temporal lobe epilepsy. Epilepsia. 2006;47:1300-1307.
20 Swartz BE, Rich JR, Dwan PS, et al. The safety and efficacy of chronically implanted subdural electrodes: a prospective study. Surg Neurol. 1996;46:87-93.
21 Behrens E, Zentner J, van Roost D, et al. Subdural and depth electrodes in the presurgical evaluation of epilepsy. Acta Neurochir (Wien). 1994;128:84-87.
22 Spencer SS, Spencer DD, Williamson PD, et al. Combined depth and subdural electrode investigation in uncontrolled epilepsy. Neurology. 1990;40:74-79.
23 Wyler AR, Ojemann GA, Lettich E, et al. Subdural strip electrodes for localizing epileptogenic foci. J Neurosurg. 1984;60:1195-1200.
24 Spencer SS. Depth electroencephalography in selection of refractory epilepsy for surgery. Ann Neurol. 1981;9:207-214.
25 Nakase H, Tamura K, Kim YJ, et al. Long-term follow-up outcome after surgical treatment for lesional temporal lobe epilepsy. Neurol Res. 2007;29:588-593.
26 Cascino GD, Trenerry MR, Sharbrough FW, et al. Depth electrode studies in temporal lobe epilepsy: relation to quantitative magnetic resonance imaging and operative outcome. Epilepsia. 1995;36:230-235.
27 Baumgartner C, Lindinger G, Lurger S, et al. Prolonged video EEG monitoring in differential diagnosis of seizures and in presurgical epilepsy diagnosis. Wien Med Wochenschr. 1998;148:2-8.
28 Drury I, Schuh L, Ross D, et al. Ictal patterns in temporal lobe epilepsy recorded by epidural screw electrodes. Electroencephalogr Clin Neurophysiol. 1997;102:167-174.
29 Kuzniecky R, Faught E, Morawetz R. Surgical treatment of epilepsy: initial results based upon epidural electroencephalographic recordings. South Med J. 1990;83:637-639.
30 Kuzniecky R, Faught E, Morawetz R. Electroencephalographic correlations of extracranial and epidural electrodes in temporal lobe epilepsy. Epilepsia. 1991;32:335-340.
31 Shih YH, Yiu CH, Huang CI. Role of foramen ovale electrodes in presurgical evaluation of intractable complex partial seizures. Zhonghua Yi Xue Za Zhi (Taipei). 1997;60:155-160.
32 Kim OJ, Ahn JY, Lee BI. Analysis of electrical discharges made with the foramen ovale electrode recording technique in mesial temporal lobe epilepsy patients. J Clin Neurophysiol. 2004;21:391-398.
33 Awad IA, Assirati JAJr, Burgess R, et al. A new class of electrodes of ‘intermediate invasiveness’: preliminary experience with epidural pegs and foramen ovale electrodes in the mapping of seizure foci. Neurol Res. 1991;13:177-183.
34 Carter DA, Lassiter AT, Brown JA. Cost-efficient localization of seizures of mesiotemporal onset with foramen-ovale electrodes. Neurol Res. 1998;20:153-160.
35 Pacia SV, Ebersole JS. Intracranial EEG substrates of scalp ictal patterns from temporal lobe foci. Epilepsia. 1997;38:642-654.
36 Marks DA, Katz A, Booke J, et al. Comparison and correlation of surface and sphenoidal electrodes with simultaneous intracranial recording: An interictal study. Electroencephalogr Clin Neurophysiol. 1992;82:23-29.
37 Kanner AM, Ramirez L, Jones JC. The utility of placing sphenoidal electrodes under the foramen ovale with fluoroscopic guidance. J Clin Neurophysiol. 1995;12:72-81.
38 Kanner AM, Parra J, Gil-Nagel A, et al. The localizing yield of sphenoidal and anterior temporal electrodes in ictal recordings: a comparison study. Epilepsia. 2002;43:1189-1196.