CHAPTER 35 Intermediate Filaments
Intermediate filaments (Fig. 35-1) are strong but flexible polymers that provide mechanical support for cells ranging from bacteria to human tissues. These filaments were named intermediate because their diameter of about 10 nm is intermediate between the diameters of the thick and thin filaments in striated muscles (see Figs. 39-3 and 39-8). Cytoplasmic intermediate filaments tend to cluster into wavy bundles that vary in compactness, forming a branching network between the plasma membrane and the nucleus. Desmosomes anchor intermediate filaments to the plasma membrane (see Fig. 31-7) and transmit mechanical forces between adjacent cells. Hemidesmosomes connect intermediate filaments across the plasma membrane to the extracellular matrix.
The continuum of intermediate filaments and junctions prevents excessive stretching of the cells and gives tissues such as epithelia their mechanical integrity. Hair, which is built from cross-linked intermediate filaments, nicely illustrates their flexibility and high tensile strength. Molecular defects in cytoplasmic intermediate filaments or junctions associated with intermediate filaments result in rupture of skin cells and blistering diseases. Defeats in lamins cause a bewildering array of diseases (see Fig. 14-9).
The nearly 70 human genes for intermediate filament proteins are expressed selectively in various cell types. All intermediate filament proteins possess an α-helical coiled-coil domain that forms the core of the filaments, while the flanking N- and C-terminal domains vary considerably in size (Fig. 35-2). These variable domains give each type of intermediate filament distinctive features but made it difficult to appreciate the general features of intermediate filament structure and function until the sequences of the proteins were determined in the 1980s.
Structure of Intermediate Filament Subunits
Each intermediate filament protein isoform has a unique amino acid sequence, but all have a rod-like domain between head and tail domains of variable length at the two ends. The rod is a parallel coiled-coil of two α-helices, usually about 47 nm long (Fig. 35-3A). Analysis of sequences, spectroscopic data, and X-ray fiber diffraction from materials composed of intermediate filaments, like wool, established this coiled-coil structure of the rod. Like other coiled-coils (see Fig. 3-10), rod domains of intermediate filament proteins have a heptad repeat of amino acids, the first and fourth residues providing a continuous row of hydrophobic interactions along the interface of the two helices. Zones of positive and negative charge alternate along the rod. When staggered appropriately, these zones provide complementary electrostatic bonds for assembly of filaments. About 20 highly conserved residues at each end of the rod are essential for filament elongation through head-to-tail interactions between dimeric molecules. Assembly studies with mutant proteins suggest that these parts of the rod contribute to lateral associations within filaments. Amino acid sequences indicate three interruptions in the coiled-coil (Fig. 35-2).
Less is known about the structures and functions of the nonhelical N- and C-terminal domains. They can influence assembly and in some cases project from the filament surface (Fig. 35-3C) to interact with other cellular components.
Intermediate filament protein molecules are commonly referred to as dimers, as they consist of two polypeptide chains. Some intermediate filament molecules are homodimers; others are heterodimers. Lamins and class III intermediate filament molecules are parallel dimers of identical polypeptides (Fig. 35-3A), whereas keratins are obligate heterodimers of one acidic (class I) and one basic (class II) keratin polypeptide. Many intermediate filament molecules form stable, partially overlapping, antiparallel molecular dimers (referred to as tetramers; Fig. 35-3D) that are believed to be intermediates in polymer assembly.
In Bacteria, the intermediate filaments are required for the asymmetrical shape of Caulobacter crescentus (Fig. 35-1C). Genes for animal intermediate filament proteins arose in early metazoan cells, encoding nuclear lamins (see Fig. 14-7). Most metazoans including chordates, mollusks, insects, and nematodes (see Fig. 2-9) retain genes for lamins. An invertebrate organism in the lineage leading to chordates duplicated a lamin gene, which was subsequently modified by deletion of the nuclear localization sequence and the CAAX box (a C-terminal prenylation site, see Fig. 7-9) giving rise to cytoplasmic intermediate filaments. (Cytoplasmic intermediate filaments of mollusks and nematodes retain more features of lamins, so they seem to have evolved separately.) After deletion of the codons for 42 residues of the coiled-coil in early chordates, further gene duplication and divergence produced the four families of genes for cytoplasmic intermediate filaments of vertebrates (Table 35-1).
Polymer Structure
Intermediate filaments are about 10 nm in diameter with wavy profiles in electron micrographs of thin sections of cells (Fig. 35-1B) or after negative staining isolated filaments (Fig. 35-3B). In some cases, the N- or C-terminal domains project from the surface (Fig. 35-3C). The most carefully studied intermediate filaments are built from four-chain, antiparallel molecular dimers that associate end to end in protofibrils like the strands of a rope (Fig. 35-3D). A cross section has up to 16 coiled-coils, but their internal arrangement is not known. Since tetramers lack polarity, intermediate filaments are considered to be apolar (i.e., both ends of the filament are equivalent; Fig. 35-3D). This is a striking difference from actin filaments (see Fig. 33-8) and microtubules (see Fig. 34-5), which depend on their polarity for many functions, including the unidirectional motion of motor proteins.
Assembly and Dynamics of Intermediate Filaments
Intermediate filaments are among the most chemically stable cellular components, resisting solubilization by extremes of temperature as well as high concentrations of salt and detergents (Fig. 35-4). Nevertheless, intermediate filaments in some cells exchange their subunits within minutes to hours during interphase. For example, if vimentin is labeled with a fluorescent dye and injected into live cells, fluorescent vimentin incorporates into cytoplasmic filaments (Fig. 35-5). After a spot of fluorescent filaments is photobleached with a laser, the fluorescence recovers over a period of several minutes, indicating that subunits along the length of the filaments exchange with a pool of unpolymerized molecules. (Fig. 38-9 shows a similar experiment with actin.) Observations on cells expressing vimentin fused to GFP confirm these properties. Vimentin and lamin filaments, but not all intermediate filaments, disassemble reversibly during mitosis in response to phosphorylation by mitotic kinases in some cells (Fig. 35-4C; also see Fig. 44-6). Other intermediate filaments appear to be very stable, including keratin filaments in epithelial cells.
Although no known motors move on intermediate filaments, motor proteins move the filaments along microtubules. A spectacular example is found in nerve cells (see Fig. 37-5C).
Posttranslational Modifications
In several cases, phosphorylation destabilizes the filaments and blocks assembly. The best examples are phosphorylation of lamins and vimentin by Cdkl: cyclin B kinase during mitosis. The enzyme phosphorylates serine residues near the ends of the rod domain. This destabilizes the filaments and contributes to the breakdown of the nuclear lamina (see Figs. 16-7 and 44-6) and depolymerization of cytoplasmic vimentin filaments (Fig. 35-5B). Keratins are also phosphorylated during mitosis but not directly by Cdkl: cyclin B kinase. During mitosis, the organization of keratins changes subtly without complete disassembly as in other intermediate filaments. The role of phosphorylation of intermediate filaments during interphase is less clear, but it might influence the structure of the cytoskeleton in response to various signals.
Neurofilaments, abundant intermediate filaments in nerve axons and dendrites (Fig. 35-9), are an exception to the rule that phosphorylation destabilizes intermediate filaments. The most stable neurofilaments are heavily phosphorylated in the large C-terminal end domain (Fig. 35-2), whereas the pool of unpolymerized molecules is not phosphorylated. The end domain containing the phosphorylation sites is not essential for assembly, so phosphorylation might influence other functions of these intermediate filaments.
Expression of Intermediate Filaments in Specialized Cells
With rare exceptions, animal and plant cells express nuclear lamins, whereas the repertoire of cytoplasmic intermediate filaments varies greatly in different cell types (Table 35-1). It is assumed that each isoform has unique properties appropriate for cells that use them. Most cells express predominantly one class of cytoplasmic intermediate filament. For example, epithelial cells express keratin and muscle cells express desmin. A few cells, such as the basal myoepithelial cells of the mammary gland, express two types of intermediate filament subunits that sort into separate filaments with different distributions in the cytoplasm. Similarly, microinjection or expression of foreign intermediate filament subunits usually (but not invariably) results in correct sorting to the homologous class of filaments.
In tissues such as skin and brain, cells express a succession of intermediate filament isoforms as they mature and differentiate. Human epidermis and its appendages (hair and glands) express 12 different keratin isoforms as they differentiate. Dividing cells at the base of the epidermis express mainly keratins 5 and 14, whereas terminally differentiating cells express keratins 1 and 10 (Fig. 35-6). The switch in keratin expression is associated with a marked increase in filament bundling, a feature that might contribute to the resistance of the surface layers of the skin to chemical dissociation. In the nervous system, supporting glial cells use a class III intermediate filament, whereas embryonic neurons first express α-internexin and later express the three different neurofilament isoforms (Table 35-1). Although the smallest neurofilament isoform (NFL) can assemble on its own in vitro, NFL plus one of the larger isoforms (NFM or NFH) is required to form intermediate filaments in neurons.
Proteins Associated with Intermediate Filaments
A number of proteins bind intermediate filaments and link them to membranes and other cytoskeletal polymers (Table 35-2). Integral membrane proteins anchor nuclear lamins to the nuclear membrane. Filaggrin helps to aggregate keratin filaments in the upper layers of skin.
Plakins are the largest family of proteins that interact with intermediate filaments. These giant proteins typically have binding sites for other cytoskeletal polymers and certain adhesive junctions, so they can link the various elements of the cytoskeleton to each other and membranes. Like several other plakins, plectin has globular domains on both ends of a 200-nm coiled-coil. Binding sites in the globular domains allow plectin to serve as an all-purpose cytoskeletal glue, cross-linking intermediate filaments to each other, to actin filaments and microtubules (Fig. 35-7), and to b4 integrins in hemidesmosomes (see Fig. 31-7). Recessive mutations in human plectin cause a rare form of muscular dystrophy associated with skin blisters. BPAG1e also links intermediate filaments to another transmembrane protein in hemidesmosomes and can bind actin filaments. Mice that lack BPAG1 have skin blisters secondary to compromised hemidesmosomes, as well as disorganized neuronal intermediate filaments that result in the death of sensory neurons. Desmoplakin links keratin to desmosomes (see Fig. 31-7).
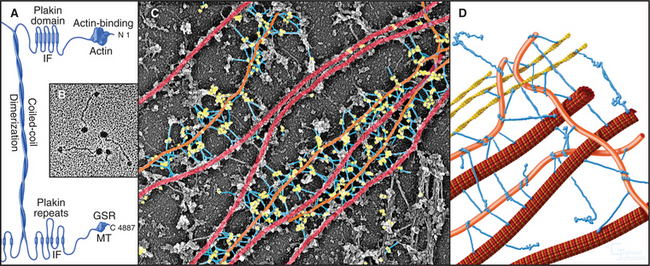
Figure 35-7 plectin structure and activities. A, Domain structure of plectin: the N-terminal domain, similar to the ABD of α-actinin (see Fig. 33-16), binds actin and intermediate filaments; the 200-nm long coiled-coil forms dimers; six C-terminal plakin repeats include a second binding site for intermediate filaments; the C-terminal GSR domain binds microtubules (MT).B, Electron micrograph of plectin molecules. C, Electron micrograph of an extracted fibroblast cell reacted with gold-labeled antibodies to plectin. Gold particles (yellow) identify plectin molecules (blue) as linkers between intermediate filaments (orange) and microtubules (red). The specimen was prepared by rotary shadowing. The molecules are pseudocolored for clarity. D, Drawing of plectin (blue) connecting cytoskeletal polymers to each other.
(B, Courtesy of G. Wiche, University of Vienna, Austria. C, Courtesy of G. Borisy, University of Wisconsin, Madison.)
Functions of Intermediate Filaments in Cells
Intermediate filaments function primarily as flexible but inextensible intracellular tendons that prevent excessive stretching of cells that are subjected to external or internal physical forces. This function is facilitated by interactions with microtubules, actin filaments, and membranes. For example, if a relaxed smooth muscle is stretched, the intracellular network of desmin filaments between cytoplasmic dense bodies and the plasma membrane (see Fig. 39-20) reorganizes from a polygonal three-dimensional network into a continuous strap that runs the length of the cell (Fig. 35-8). Up to the point at which this network is taut, the cell offers little resistance to stretching. Once the network is taut, the cell strongly resists further stretching. Actin filaments anchored to dense bodies apply contractile force to the network of intermediate filaments.
Although the geometry is different in striated muscles, the concept is remarkably similar to smooth muscle. Desmin filaments surround the Z disks in addition to forming a looser, longitudinal basket around the myofibrils (see Fig. 39-8). The ends of both skeletal and cardiac muscle cells must be anchored to transmit their contractile forces. This is accomplished by intercellular junctions that combine features of desmosomes or hemidesmosomes (anchoring intermediate filaments) and adherens junctions (anchoring actin filaments).
Keratin intermediate filaments are the major proteins in skin, where they form a dense network connected to numerous desmosomes and hemidesmosomes (Figs. 35-1 and 35-6). These junctions anchor a physically continuous network of intermediate filaments, imparting mechanical stability to the epithelium. If either the junctions or keratin filaments fail, cells pull apart or rupture, and the skin blisters. Mutations that compromise intermediate filament assembly or their anchoring junctions illustrate the importance of this network. Point mutations near the ends of the keratin rod cause especially severe forms of skin diseases (such as epidermolysis bullosa simplex) characterized by blistering and sensitivity to mechanical stress. Similar mutations engineered in transgenic mice reproduce the human disease. Which epithelial cells are affected depends on the expression pattern of the defective keratin. For example, a mutation in the rod domain of keratin 14 or keratin 5 leads to disruption of the basal cells in the epidermis where these keratins are ex-pressed. Similarly, mutations in keratin 10 or keratin 1 cause cellular rupture at higher levels in the epider-mis where these keratins are found. Mutations in keratin 12 or keratin 3 cause sores on the cornea of the eye where they are expressed.
In contrast to these dominant negative keratin mutations, complete loss of an intermediate filament protein can be less severe (Fig. 35-6D). Mice and humans that lack keratin 14 suffer from milder blistering than do patients with dominant negative point mutations. Mice without functional keratin 8 or keratin 18 genes may die during embryonic development, but some survive with only modest defects in their colon and liver. Remarkably, mice also survive deletion of both copies of the genes for class III intermediate filaments. Mice that lack desmin are viable but with mildly disorganized muscle architecture that is aggravated by vigorous exercise. Humans who are heterozygous for desmin mutations can suffer severely from generalized muscle failure, including signs of heart disease.
Neurofilaments have a second function that is equal in importance to their mechanical properties. Once a nerve cell forms synapses (see Figs. 11-8 and 11-9), it produces neurofilaments to fill the axon and expand its diameter (Fig. 35-9). This enhances electrical communication in the nervous system because the velocity of action potentials (see Fig. 11-6) depends on the diameter of axons.
Lamins were originally thought to be a simple support network for the nuclear envelope, but they have other important functions. For example, perturbation of lamin assembly by expressing toxic fragments of lamins in cells can interfere with DNA replication. This may reflect a role for the lamina in organizing the chromosomal architecture in the interphase nucleus. Mutations in the lamin A/C gene cause diverse human diseases, including premature aging (see Fig. 14-9), the Emery-Dreifuss form of muscular dystrophy as well as disorders of fat tissue and nerves. These high tissue-specific deficiencies are remarkable given the expression of lamins A and C in all tissues.
Braun S, Panatel K, Muller P, et al. Cytokeratin-positive cells in the bone marrow and survival of patients with stage I, II or III breast cancer. N Engl J Med. 2000;342:525-533.
Erber A, Riemer D, Bovenschulte M, Weber K. Molecular phylogeny of metazoan intermediate filament proteins. J Mol Evol. 1998;47:751-762.
Fuchs E, Cleveland DW. A structural scaffolding of intermediate filaments in health and disease. Science. 1998;279:514-519.
Helfand BT, Chang L, Goldman RD. The dynamic and motile properties of intermediate filaments. Annu Rev Cell Dev Biol. 2003;19:445-467.
Herrmann H, Aebi U. Intermediate filaments: Molecular structure, assembly mechanism, and integration into functionally distinct intracellular scaffolds. Annu Rev Biochem. 2004;73:749-789.
Hutchison CJ. Lamins, building blocks or regulators of gene expression? Nat Rev Mol Cell Biol. 2002;3:848-858.
Leung CL, Green KJ, Liem RKH. Plakins: A family of versatile cytolinker proteins. Trends Cell Biol. 2002;12:37-45.
Moller-Jensen J, Löwe J. Increasing complexity of the bacterial cytoskeleton. Curr Opin Cell Biol. 2005;17:75-81.
Omary MB, Coulombe PA, McLean WHI. Intermediate filament proteins and their associated diseases. New Engl J Med. 2004;351:2087-2100.
Wiche G. Role of plectin in cytoskeleton organization and dynamics. J Cell Sci. 1998;111:2477-2486.
Worman HJ, Courvalin J-C. The nuclear lamina and inherited disease. Trends Cell Biol. 2002;12:591-598.