CHAPTER 101 Instability: Clinical Manifestations and Assessment
INTRODUCTION
Lumbar segmental instability is an important but often unrecognized cause of chronic low back pain (LBP). It has been a controversial and poorly understood topic, primarily because of the varying definitions and usage among the several disciplines involved in the treatment of spinal disorders.1
Traditionally, one of the most obvious manifestations of lumbar instability has been the radiographic diagnosis of spondylolisthesis,2,3 with increased segmental motion reported in patients with chronic LBP.4–7 However, lumbar segmental instability (‘microinstability’) also has been cited as a cause of chronic LBP in patients without an injury to the osseous spine,8 with a number of studies reporting increased and abnormal intersegmental motion in these patients.9–11
DEFINITION OF SPINAL INSTABILITY
Numerous biomechanical and clinical definitions have been developed to describe spinal instability; however, most, including the one adopted by the American Academy of Orthopaedic Surgeons, imply excessive motion beyond normal constraints.12–16 Despite a multitude of biomechanical, radiographic, and clinical studies, ‘normal’ motion of the lumbar vertebral segments continues to be a topic of debate because of the lack of standardized measurements in normal subjects.17
Correlating clinical instability and radiographic instability also has been difficult because of the overlap of symptomatic and asymptomatic motion patterns. Additionally, conventional radiography often is insensitive and unreliable in detecting abnormal or excessive intersegmental motion.18,19 Conceptually, therefore, as with other spinal conditions, radiographic abnormalities (more specifically, abnormal motion of a single motion segment) are considered significant only if they confirm the clinical finding (of lumbar segmental instability) at the corresponding symptomatic level.14
As the pathomechanics of the lumbar spine have become better understood, definitions of spinal instability have been developed that incorporate both the mechanical aspect and the clinical consequence. In 1992, Panjabi defined spinal instability as a region of laxity around the neutral position of a spinal segment, called the neutral zone, and more precisely as a decrease in the capacity of the stabilizing systems of the spine to maintain intervertebral neutral zones within physiological limits within which there is no major deformity, no neurological deficit, and no incapacitating pain.20 This definition is useful because it describes the quality of motion throughout the range of motion rather than relying solely on the total range of motion values for diagnosis.21
THE NEUTRAL ZONE CONCEPT AND THE SPINAL STABILIZING SYSTEM
The neutral zone concept of segmental instability is based on the observation that the load-displacement curve of the spine is nonlinear (i.e. the ratio of the load applied to the displacement produced is not consistent), with minimal resistance to intervertebral motion occurring within the neutral zone and increased resistance to intervertebral motion occurring at the end-ranges of spinal motion.22 This concept has been described as a ‘ball in a bowl’ (Fig. 101.1). The ball rests in a bowl created by flipping the extension part of the load-displacement curve around the displacement axis. The ball moves easily within the base of the bowl (the neutral zone), but requires greater effort to move in the steeper part of the bowl (the end-ranges of motion). The shape of the bowl is analogous to spinal stability: a deeper bowl, such as a wine glass, represents a more stable spine, while a shallower bowl, such as a soup plate, represents a less stable spine (Fig. 101.2).22 The total range of motion of a spinal motion segment, therefore, can be divided into a neutral zone and an elastic zone. The neutral zone is the initial spinal motion which is produced against minimal internal resistance, whereas the elastic zone is motion nearer to the end-range of movement that is produced against significant internal resistance.23 In order for the stabilizing system of the spine to be effective, it must limit the excursion of spinal motion within a segment and maintain the proper ratio of neutral to elastic motion.23
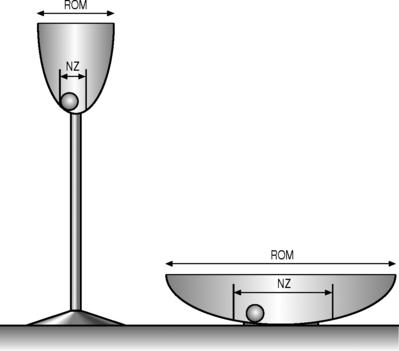
Fig. 101.2 Representation of different spinal stabilities. Using the analogy of a ball in a bowl to represent the load-displacement curve of the spine (Fig. 101.1), a deep champagne glass and a shallow soup plate represent a more and a less stable spine, respectively.
(Adapted from Panjabi MM. Clinical spinal stability and low back pain. J Electromyogr Kinesiol 2003; 13:371–379.)
Biomechanical studies of the stabilizing system of the spine have provided insight into the roles of the various components providing spinal stability. The stabilizing system of the spine is composed of three subsystems: (1) the spinal column or passive subsystem, (2) the spinal muscles or active subsystem, and (3) the neural control unit.20,22 Because, under normal conditions, the three subsystems work in harmony to provide mechanical stability, their functions are interrelated, and decreased function of one subsystem may result in increased demands on the others to maintain stability.24,25 The various components of the spinal column generate transducer information about the mechanical status of the spine, such as position, load, and motion of each vertebra, and the neural control unit computes the needed stability and generates the appropriate muscle pattern (Fig. 101.3).23 A comprehensive knowledge of the three subsystems of the lumbar spine is essential in understanding spinal stabilization and clinically evaluating patients with LBP.
The passive subsystem
The passive subsystem consists primarily of the vertebral bodies, zygapophyseal joints and joint capsules, spinal ligaments, and passive tension from the musculotendinous units.20 The passive subsystem plays its most important stabilizing role in the elastic zone of the spinal motion (i.e. near the end-ranges of motion).25 Serial sectioning26,27 and mathematical modeling25,28 have been used to investigate the relative contributions of structures to segmental stability. Spinal flexion is stabilized primarily by the posterior ligaments of the spine (interspinous and supraspinous ligaments), the zygapophyseal joints and joint capsules, and the intervertebral discs,28,29 while end-range extension is stabilized primarily by the anterior longitudinal ligament, the anterior aspect of the anulus fibrosus, and the zygapophyseal joints.25,27 Rotational movements of the lumbar spine are stabilized mostly by the intervertebral discs and the zygapophyseal joints.30 Although not studied extensively, the intertransverse ligaments appear to play an important role in segmental stability during side-bending movement.26
Because afferent nerve fibers capable of conveying proprioceptive information are present in most of the structures of the passive subsystem, including the intervertebral discs, the zygapophyseal joint capsules, and the interspinous and supraspinous ligaments,24,31 these structures may function as force transducers in the neutral zone, sensing changes in position and providing feedback to the neural control subsystem.20,26,31 Injury to the passive subsystem, such as intervertebral disc degeneration or disruption of the posterior ligaments of the spine, may increase the size of the neutral zone, increasing the demands on the active and neural control subsystems to avoid the development of segmental instability.20,22,32
The active subsystem
The active subsystem of the spinal stabilizing system consists of the spinal muscles and tendons. The active and neural control subsystems are primarily responsible for spinal stability in the neutral zone, where passive resistance to movement is minimal.20,27 Without musculotendinous support, the lumbar spine is highly unstable at very low applied loads.33 The relative contributions of different muscle groups to lumbar spine stability have not been clearly delineated, despite much research.34–38
The contributions of the deeper, unisegmental muscles differ from those of the more superficial multisegmental muscles, such as the abdominal and erector spinae muscles.34 Because of their small size, their close proximity to the center of rotation for spinal movements, and their high concentration of muscle spindles,39,40 the unisegmental muscles of the lumbar spine, such as the intertransversarii and the interspinalis muscles, are believed to function primarily as force transducers, providing feedback on spinal position and movements to the neural control subsystem.20
The larger multisegmental muscles are responsible for producing and controlling movements of the lumbar spine, primarily lifting and rotational movements. The lumbar erector spinae muscle group provides most of the extensor force required for most lifting tasks,41 while rotation is produced primarily by the oblique abdominal muscles.35 Both of these muscles have few or no direct attachments to the lumbar spinal motion segments and, therefore, do not exert forces directly on individual motion segments. The multifidus muscle, which originates from the spinous process of the lumbar vertebrae and forms a series of repeating fascicles attaching to the inferior lumbar transverse process, the ilium, and the sacrum, provides segmental control. The multifidus muscle functions as a stabilizer during lifting and rotational movements of the lumbar spine.42 Although not studied extensively, the quadratus lumborum muscle is believed to be the primary active stabilizer during movements in the frontal plane.43
The role of the abdominal muscles in spinal stability has been suggested to be the generation of extensor force during lifting tasks, either by increasing intra-abdominal pressure or by creating tension in the lumbodorsal fascia.36,44 Research indicates, however, that the abdominal muscles are not capable of generating substantial extensor force through these mechanisms.37,42 The abdominal muscles are primarily flexors and rotators of the lumbar spine.45 The oblique abdominal and transverses abdominis muscles, with their more horizontal orientation, are thought to contribute to spinal stability by creating a rigid cylinder around the spine and by increasing the stiffness of the lumbar spine.38,46 Continuous activity of the transverses abdominis muscle has been demonstrated throughout flexion and extension movements of the lumbar spine.47
The neural control subsystem
The neural control subsystem receives input from structures in the passive and active subsystems that allows it to determine the specific requirements for maintaining spinal stability and to act through the spinal musculature to stabilize the spine.20,38,48 Dysfunction of the neural control system may place other spinal structures at risk for injury,20 and the risk of reinjury may be increased if proper functioning of the neural control system is not restored after injury.48
No evidence has linked poor neuromuscular control to an increased risk of initial injury to the lumbar spine, but several studies38,49–52 have shown that patients with LBP often have persistent deficits in neuromuscular control, indicating that recovery of proper function of the neural control subsystem is not automatic following an initial injury. Increased postural sway and slower reaction times have been identified in patients with LBP compared to subjects without LBP.49–51 Luoto et al.50 found in patients undergoing rehabilitation that improvements in reaction time correlated with reduced disability, indicating that neuromuscular control deficits often persist after lumbar spine injury and that reduction in these deficits correlates with improvement in functional status.
The neural control system also may help stabilize the spine in anticipation of an applied load. Hodges and Richardson38,52 reported that transverses abdominis and multifidus muscle activity consistently preceded active extremity movement in subjects without LBP, but in patients with LBP the contraction of the transverses abdominis muscles was delayed, possibly indicating deficient neural control. Further research is needed to clarify the role of neural control in patients with LBP, but these preliminary findings indicate that enhancing neural control may be an important consideration in the prevention and rehabilitation of LBP.
A HYPOTHESIS THAT RELATES MOTION TO PAIN
The relationship between abnormal intervertebral motion and low back pain is inherent in the definition of clinical spinal instability. In theory, therefore, if intervertebral motion is decreased in a patient with LBP, pain also should be decreased. This assumption is the basis for low back treatments involving surgical fusion, muscle strengthening, and muscle control training.23 Based on the hypothesis that severe LBP is caused by instability between lumbar segments, Olerud et al.53 used external fixation to produce ‘instantaneous fusion’ of selected lumbar spinal segments in 18 patients with chronic severe LBP; 17 patients experienced ‘remarkable relief’ of pain. The authors suggested that this test could be used to identify unstable segments before surgery. Panjabi et al.,54 in a biomechanical cadaver study of the cervical spine, found that after stabilization with external fixation the average range of motion decreased by 39.3%, while the neutral zone decreased by an average of 68.8%.
Using the ‘ball in a bowl’ analogy, Panjabi23 formulated a hypothesis to relate motion to pain (Fig. 101.4). He postulated that in people without spinal pain the neutral zone and range of motion are normal and the ball moves freely within the pain-free zone (Fig. 101.4A). Injury to or degenerative changes in a spinal column component result in an increase in the neutral zone and the ball moves freely over a larger distance, beyond the pain-free zone (Fig. 101.4B). The spinal stabilizing system reacts to decrease the neutral zone by activating the muscles or by adaptive stiffening of the spinal column over time (e.g. formation of osteophytes) (Fig. 101.4C). The spine also may be stabilized by surgical fusion, muscle strengthening, and retraining of the neuromuscular control system. In the analogy, the ball is now anchored and the spine is again pain free. This hypothesis is unproven and must be validated by future clinical studies.23
CLINICAL ASSESSMENT OF LUMBAR INSTABILITY
General classification and evaluation
Lumbar segmental instability can be viewed as a purely movement syndrome (Table. 101.1): microinstability (without osseous injury) in which directional patterns of motion produce an observed lack of movement control and related symptoms within the neutral zone or may be associated with other conditions (Table. 101.2),55 which are discussed in other chapters. In general, the principles derived from traumatic conditions also are applicable to infections and tumors, which produce instability by mechanical weakening of the anterior and middle columns of the spine. For the evaluation of acute spinal injuries caused by trauma, tumor, or infection, White and Panjabi developed a checklist (Table. 101.3) based on anatomy, biomechanics, and clinical observations with the goal of establishing reproducible guidelines.56 In chronic degenerative clinical instability, however, the link between objective findings, which usually are radiographic, and clinical symptoms is not clear and can be confused by coexisting degenerative conditions such as stenosis or spondylolisthesis. An ongoing challenge is to develop diagnostic tests that are both sensitive and specific enough to clearly identify segmental instability.
Table 101.1 Directional patterns in syndromes causing microinstability
Flexion pattern |
Extension pattern |
Lateral shift pattern |
Multidirectional pattern |
Table 101.2 Conditions associated with lumbar segmental instability
From: Hazlett JW, Kinnard P. Lumbar apophyseal process excisions and spinal instability. Spine 1982; 7:171–176.
Table 101.3 Checklist for the diagnosis of clinical instability in the lumbar spine
Element | Point value |
---|---|
Anterior elements destroyed or unable to function | 2 |
Posterior elements destroyed or unable to function | 2 |
Radiographic criteria | |
Flexion–extension radiographs | 4 |
Sagittal plane translation >4.5 mm or 15% | |
Sagittal plane rotation >15° at L1–2 and L3–4 | |
>20° at L4–5 | |
>25° at L5–S1 | |
OR | |
Resting radiographs | |
Sagittal plane displacement >4.5 mm or 15% | |
Relative sagittal plane angulation >22° | |
Cauda equina damage | 3 |
Dangerous loading anticipated | 1 |
TOTAL OF 5 OR MORE = UNSTABLE |
From: White AA, Panjabi MM. Clinical biomechanics of the spine. Philadelphia: JB Lippiincott; 1990.
Traditionally, the diagnosis of instability has been based on radiographic observations and measurements. Although disc space narrowing, air within the disc, and calcification of the disc have been observed with spinal instability,57–60 as have hypertrophic changes of the facet joints and claw osteophytes, these are signs of degeneration, not specifically of instability, and are not consistently correlated with symptoms. Macnab61 described the traction spur, which is a horizontal osteophyte arising at the attachment of the outer fibers of the anulus on the anterior and lateral aspects of the vertebral body, 2 mm away from its disc surface. This spur is thought to result from excessive tensile stresses applied to the outer vertebral body through the annular fibers as a consequence of segmental instability. No direct, statistically valid, clinical significance of the traction spur, however, has been established.
Knutsson62 described a method for diagnosing segmental instability based on lateral radiographs of the lumbar spine taken with the patient in maximal active extension while standing and in maximal active flexion while sitting. He defined instability as 3 mm or more of anterior translation measured between flexion and extension radiographs. Flexion–extension radiographs have now become the standard by which segmental instability is diagnosed. A variety of methods have been developed to more precisely quantify these displacements63–66 either on neutral views or by comparison of flexion and extension views. The amount of anterolithesis considered indicative of instability varies from 3 mm to 6 mm or 6–15% of the width of the adjacent vertebra (Fig. 101.5).19,55 Suggested criteria for instability include a relative sagittal angulation of more than 22 degrees on resting radiographs (Fig. 101.6) or more than 15 degrees at L1–2, L2–3, and L3–4 levels, more than 20 degrees at the L4–5 level, and more than 25 degrees at the L5–S1 level on flexion–extension films (Fig. 101.7).67 Limitations in these techniques are that the ranges of normality have not been well established and the accuracy in measurement is low.68,69 Additionally, variations in the exact technique used, large variability in the motion characteristics of individuals without LBP, and high false-positive rates using the established criteria have resulted in questions about the usefulness of flexion–extension radiographs.53,69 Because of the intra- and interobserver errors in assessing instability radiographically, Spratt et al.70 recommended that a minimum of 4 mm of forward displacement at L3–4 and L4–5 and displacement of more than 5 mm at L5–S1 are necessary to accurately measure instability.
Many other techniques using lateral bending films, bipolar radiography, multidirectional reconstruction, computer digitization, and stereophotogrammetry have been investigated in measuring spinal motion and determining instability.59,71 These methods, however, have not achieved practical clinical use and have not established specific criteria for spinal instability. Perhaps most indicative of chronic instability is the radiographic progression of malalignment or deformity over time, the best two examples of which are degenerative spondylolisthesis and degenerative scoliosis. Newman and Stone72 determined that in degenerative spondylolisthesis progression averaged 2 mm every 4 years. Similar observations have been made about adult degenerative scoliosis73 and, to a lesser degree, retrolithitic deformities. Of all the radiographic observations and measurements mentioned, however, none has statistically proved to be specifically associated with significant clinical symptoms.57–59,69,71,74 In order to establish a diagnosis of spinal instability, therefore, the clinician must correlate the biomechanical environment and radiographic observations with the patient’s clinical history and physical examination.
To date, no diagnostic gold standard for segmental instability has been identified. Historically, patients complain of chronic and recurrent pain localized to the low back or radiating into the lower extremities and associated with high levels of functional disability.58 Because the condition is mechanically based, the pain typically worsens with greater loads on the spine such as general activity, lifting, standing, sitting, and motions such as bending or twisting. Kirkaldy-Willis and Farfan suggested that segmental instability is a condition in which ‘minor perturbations produce acute pain.’14 Conversely, the pain is relieved by lying supine, the position that places the least mechanical load on the lumbar spine.75 Patients most commonly describe their back pain as recurrent, constant, catching, locking, giving way, or associated with a feeling of instability.76 Several researchers have reported similar physical examination findings that are consistent with a movement–control problem within the neutral zone:14,56,77 (1) active spinal movement with good ranges of spinal mobility but with ‘through-range’ pain or a painful arc rather than end-of-range limitation of motion, and (2) the inability to return to erect standing from forward bending without the use of the hands to assist in this motion. Segmental shifts or hinging were frequently associated with the painful movement. Deep abdominal muscle activation during the provocative movement often reduced or eliminated pain. Neurological examination and neural tissue provocation tests were generally normal.78
Clinical syndromes of microinstability
The directional nature of instability based upon the mechanism of injury, resultant site of tissue damage, and clinical presentation is well understood in the knee and shoulder but poorly understood in the lumbar spine. Based on experimental and radiological data, Dupuis et al.64 concluded that the location of the dominant lesion in the motion segment determines the pattern of instability manifested. Because the motion within the lumbar spine is three-dimensional and involves coupled movements, tissue damage is likely to cause movement dysfunction in more than one direction.76
Common to all the microinstability syndromes are the patient’s subjective feeling of instability and an objectively observed lack of movement control and related symptoms within the neutral zone, all of which are associated with an inability to initiate co-contraction of the local muscle system within this zone. The local muscle system consists of muscles that attach directly to the lumbar vertebrae and are responsible for providing segmental stability and directly controlling the lumbar segments. The lumbar multifidus, psoas major, quadratus lumborum, the lumbar parts of the lumbar iliocostalis and longissimus, transverses abdominis, the diaphragm, and posterior fibers of the oblique abdominis internus form part of this local muscle system. Patients may develop compensatory movement strategies that ‘stabilize’ the motion segment out of the neutral zone and towards an end-range position (such as flexion, lateral shift, or extension). This is achieved by the recruitment of global system muscles and by generating high levels of intra-abdominal pressure during low-load tasks. The global muscle system consists of large torque-producing muscles that act on the trunk and spine without directly attaching to it. These muscles include the rectus abdominus, obliquus abdominus externus, and the thoracic part of the lumbar iliocostals; they provide general trunk stabilization but are not capable of exerting a direct segmental influence on the spine.76,78
Flexion pattern of microinstability
Patients with flexion pattern microinstability, the most common type, complain of central back pain and relate their injury to either a single flexion–rotation injury or to repetitive strains relating to flexion–rotation activities. Symptoms and ‘vulnerability’ are aggravated by flexion–rotation movements and patients are unable to sustain semiflexed postures (Fig. 101.8). A loss of segmental lumbar lordosis at the level of the ‘unstable motion segment’ often is noticeable when the patient is standing and is accentuated in sitting postures because patients tend to hold the pelvis in a degree of posterior pelvic tilt. Lower lumbar segmental lordosis is further decreased in flexed postures and usually is associated with increased tone in the upper lumbar and lower thoracic erector spinae muscles, with an associated increase in lordosis in this region. During forward bending, patients have a tendency to flex more at the symptomatic level than at the adjacent levels, usually producing an arc of pain and an inability to return from flexion to neutral without use of the hands to assist the movement. During backward bending, extension often is less in the affected segment than in the more proximal segments. Specific movement testing reveals an inability to differentiate anterior pelvic tilt and low lumbar spine extension independent of upper lumbar and thoracic spine extension. Movement tests such as squatting, sitting with knee extension or hip flexion, ‘sit to stand,’ and forward-loaded postures reveal an inability to control a neutral segmental lordosis, with a tendency to segmentally flex at the unstable motion segment, posteriorly tilt the pelvis, and extend the upper lumbar and thoracic spine.
Extension pattern of microinstability
Patients with extension pattern microinstability relate their injury to an extension–rotation incident or repetitive trauma usually associated with sporting activities involving extension–rotation. Their symptoms are aggravated by extension and extension–rotation movements and activities such as standing, fast walking, running, swimming, and overhead activities such as throwing (Fig. 101.9). When the patient is standing, segmental lordosis at the unstable motion segment usually is increased, sometimes with an increased level of segmental muscle activity at this level and anterior pelvic tilt. Extension activities reveal segmental hinging at the affected segment with a loss of segmental lordosis above this level and associated postural ‘sway.’ With the patient prone, hip extension and knee flexion movement tests reveal a loss of co-contraction of the deep abdominal muscles and dominant patterns of activation of the lumbar erector spinae that cause excessive segmental extension–rotation at the unstable level. During forward bending, these patients tend to hold the lumbar spine in lordosis (particularly at the level of the unstable motion segment), with a sudden loss of lordosis at the midrange of flexion that usually is associated with an arc of pain. During return-to-neutral, they tend to hyperlordose the spine segmentally before the upright position is achieved, with pain on returning to the erect posture and the necessity to assist the movement with the use of the hands. Specific movement tests reveal an inability to initiate posterior pelvic tilt independent of hip flexion and activation of the gluteals, rectus abdominis, and external obliques.
Lateral shift pattern of microinstability
The recurrent lateral shift pattern of microinstability usually is unidirectional and is associated with unilateral low back pain, usually reported to occur during reaching or rotating in one direction with the spine flexed (Fig. 101.10). This is the same movement direction that patients report as ‘injuring’ their back.
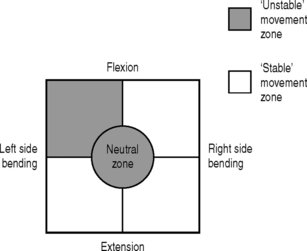
Fig. 101.10 Unstable movement zone: lateral shift pattern.
(Adapted from O’Sullivan PB. Lumbar segmental instability: clinical presentation and specific stabilizing exercise management. Manual Therapy 2000; 5:2–12.)
Their standing posture is similar to that of patients with flexion pattern microinstability, with a loss of lumbar segmental lordosis at the affected level, but with an associated lateral shift at the same level. Palpation of the lumbar multifidus muscles with the patient standing commonly reveals resting muscle tone on the side of the shift, and atrophy and low tone on the contralateral side. The lateral shift is accentuated when the patient stands on the foot ipsilateral to the shift. When walking, the patient has a tendency to transfer weight through the trunk and upper body rather than through the pelvis. Sagittal spinal movements cause a further lateral shift at the midrange of flexion, usually associated with an arc of pain. With the patient supine, rotary and lateral trunk control is lost in the direction of the shift, with asymmetrical leg loading and unilateral bridging, and during four-point kneeling trunk control is lost when one arm is flexed. Sitting-to-standing and squatting movements usually reveal a tendency towards lateral trunk shift with increased weight-bearing on the side of the shift.
Multidirectional pattern of microinstability
The most serious and debilitating of the clinical presentations, multidirectional microinstability frequently is associated with a traumatic injury and high levels of pain and functional disability. Provocative movements are described as multidirectional (Fig. 101.11), all weight-bearing postures are painful, and pain-relieving positions during weight-bearing are difficult to obtain. Locking of the spine commonly is reported after sustained flexion, rotation, and extension postures, and patients may assume a flexed, extended, or laterally shifted spinal posture. Excessive segmental shifting and hinging patterns may be present in all movement directions, with ‘jabbing’ pain and associated back muscle spasm. Assuming neutral lordotic spinal positions is very difficult, and attempts to facilitate lumbar multifidus and transverses abdominis co-contraction (especially during weight bearing) usually are associated with a tendency to flex, extend, or laterally shift the spine segmentally, with associated global muscle substitution, bracing of the abdominal wall, and pain. Palpation reveals multidirectional increased intersegmental motion at the symptomatic level. High levels of irritability and an inability to tolerate compression loading in any position indicate a poor prognosis for conservative exercise management.
REHABILITATION OF INSTABILITY
A recent focus in the rehabilitation of patients with chronic low back pain has been the training of specific muscles, such as the transverses abdominis, diaphragm, and lumbar multifidus, involved in the dynamic stability and segmental control of the spine based on the identification of specific motor control deficits in these muscles.79–81 Once the faulty movement pattern or patterns are identified, the individual components of the movement are isolated and the muscles are retrained for functional tasks specific to the patient’s needs. O’Sullivan et al. have reported reductions in pain and functional disability with this exercise training approach in patients with chronic low back pain and a diagnosis of lumbar segmental instability.76,80,82 In its simplest form, this exercise program represents the process of motor learning; three stages have been described in the learning of a new motor skill (Fig. 101.12).76,83
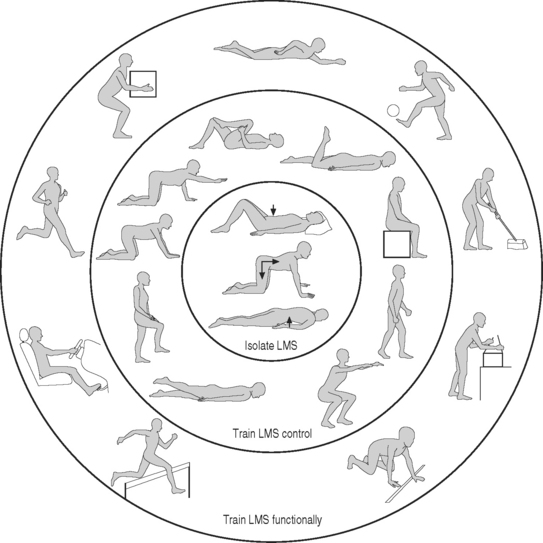
Fig. 101.12 Stages of rehabilitation based on a motor learning model (LMS, local muscle system).
(Adapted from O’Sullivan PB. Lumbar segmental instability: clinical presentation and specific stabilizing exercise management. Manual Therapy 2000; 5:2–12.)
First stage of rehabilitation
The inhibition of global muscle substitution is mandatory if appropriate co-contraction is to be obtained. The actions of the obliquus externus abdominis and rectus abdominis muscles can be inhibited by having the patient focus on the pelvic floor contraction and on optimal postural alignment during weight-bearing; ensuring upper lumbar lordosis and lateral costal diaphragm breathing also are beneficial to open the sternal angle. The thoracolumbar erector spinae muscles can be inhibited by having the patient avoid thoracic spine extension and excessive lumbar spine lordosis, focus on independence of pelvic and low lumbar spine movement from thoracic spine and hip movement, and continue lateral costal diaphragm breathing. Palpatory and electromyogram (EMG) biofeedback and muscle release techniques also are helpful.
Third stage of rehabilitation
The third stage of rehabilitation is the autonomous stage, in which correct performance of motor tasks requires only a low degree of attention.83 At this stage, patients can automatically stabilize their spine during functional demands of daily living. The success of specific exercise intervention in achieving automatic patterns of muscle recruitment has been validated by surface EMG data and reports of long-term good outcomes in patients who completed the protocol.80,82,84
SUMMARY
Lumbar segmental instability continues to be a diagnostic challenge. Correlating clinical instability and radiographic instability has been difficult because of the overlap of symptomatic and asymptomatic motion patterns. Additionally, conventional radiography often is insensitive and unreliable in detecting abnormal or excessive intersegmental motion. As the pathomechanics of the lumbar spine have become better understood, however, defining instability in terms of quality of motion throughout the range of motion rather than relying solely on the traditional total range of motion values for diagnosis has been useful in providing a basis by which patients can be evaluated in a segment-specific and individual manner (Fig. 101.13). Lumbar segmental instability can be viewed as a purely movement syndrome: microinstability (without osseous injury) in which directional patterns of motion produce an observed lack of movement control and relative symptoms within the neutral zone or may be associated with other conditions. With increasing understanding of the relative involvement of different muscle groups contributing to lumbar stability, diagnosis-specific rehabilitation protocols are being developed to assist in the assessment and treatment of patients with lumbar instability. Good results have been reported with such an approach using a motor learning model in which the faulty movement pattern or patterns are identified and the components of the movement are isolated and retrained into functional tasks specific to the patient’s individual needs. Scientific trials comparing this approach to other treatment methods are required to validate its efficacy.
1 Eisenstein SM. Instability and low back pain. A way out of the semantic maze. In: Szpalski M, Gunzburg R, Pope MH, editors. Lumbar segmental instability. Philadelphia: Lippincott Williams & Wilkins; 1999:39-44.
2 Nachemson A. Instability of the lumbar spine. Neurosurg Clin North Am. 1991;2:785-790.
3 Pope M, Frymoyer J, Krag M. Diagnosing instability. Clin Orthop. 1992;296:606-667.
4 Friberg D. Functional radiography of the lumbar spine. Ann Med. 1939;21:341-346.
5 Mimura M. Rotational instability of the lumbar spine; a three-dimensional motion study using biplane X-ray analysis system. Nippon Seikeigeka Gakkai Zasshi. 1990;64:546-559.
6 Montgomery D, Fischgrund J. Passive reduction of spondylolisthesis on the operating room table: a retrospective study. J Spinal Disord. 1994;7:167-172.
7 Wood K, Popp C, Transfeldt E, et al. Radiographic evaluation of instability in spondylolisthesis. Spine. 1994;19:1697-1703.
8 Long D, BenDebba M, Torgenson W. Persistent back pain and sciatica in the United States: patient characteristics. J Spinal Disord. 1996;9:40-58.
9 Sihvonen T, Partanen J. Segmental hypermobility in lumbar spine and entrapment of dorsal rami. Electromyogr Clin Neurophysiol. 1990;30:175-180.
10 Gertzbein S. Segmental instability of the lumbar spine. Semin Spinal Surg. 1991;3:130-135.
11 Lindgren K, Sihvonen T, Leino E, et al. Exercise therapy effects on functional radiographic findings and segmental electromyographic activity in lumbar spine instability. Arch Physical Med Rehab. 1993;74:933-939.
12 Gertzbein SD, Seligman J, Holtby R, et al. Centrode patterns and segmental instability in degenerative disc disease. Spine. 1985;10:257-261.
13 Kirkaldy-Willis WH. Presidential symposium on instability of the lumbar spine. Introduction. Spine. 1985;10:254.
14 Kirkaldy-Willis WH, Farfan HF. Instability of the lumbar spine. Clin Orthop. 1982;165:110-123.
15 Panjabi MM, Thibodeau LL, Crisco JJ, et al. What constitutes spinal instability? Clin Neurosurg. 1988;34:313-339.
16 Pope MH, Panjabi M. Biomechanical definition of spinal instability. Spine. 1985;10:255-256.
17 Boden SD, Wiesel SA. Lumbosacral segmental motion in normal individuals. Have we been measuring instability properly? Spine. 1989;15:571-576.
18 Dvorak J, Panjabi MM, Novotny JE, et al. Clinical validation of functional flexion–extension roentgenograms of the lumbar spine. Spine. 1991;16:943-950.
19 Pope M, Frymoyer J, Krag M. Diagnosing instability. Clin Orthop. 1992;296:60-67.
20 Panjabi MM. The stabilizing system of the spine. Part I. Function, dysfunction, adaptation, and enhancement. J Spinal Disord. 1992;5:383-389.
21 Fritz JM, Erhard RE, Hagen BF. Segmental instability of the lumbar spine. Phys Ther. 1998;78:889-896.
22 Panjabi MM. The stabilizing system of the spine. Part II. Neutral zone and instability hypothesis. J Spinal Disord. 1992;5:390-396.
23 Panjabi MM. Clinical spinal instability and low back pain. J Electromyogr Kinesiol. 2003;13:371-379.
24 Indahl A, Kaigle AM, Reikeras O, et al. Interaction between the porcine lumbar intervertebral disc, zygapophyseal joints, and paraspinal muscles. Spine. 1997;22:2834-2842.
25 Haher TR, O’Brien M, Dryer JW, et al. The role of the lumbar facet joints in spinal stability: identification of a alternative paths of loading. Spine. 1994;19:2667-2670.
26 Panjabi MM, Goel VK, Takata K. Physiologic strains in the lumbar spinal segments: an in vitro biomechanical study. Spine. 1982;7:192-203.
27 Sharma M, Langrana NA, Rodriguea J. Role of ligaments and facets in lumbar spinal instability. Spine. 1995;20:887-900.
28 McGill SM. Estimation of force and extensor moment contributions of the disc and ligaments at L4–L5. Spine. 1988;13:1395-1402.
29 Adams MA, Hutton MC, Stott JRR. The resistance to flexion of the lumbar intervertebral joint. Spine. 1980;5:245-253.
30 Farfan HF, Cossette JW, Robertson GW, et al. The effects of torsion on the lumbar intervertebral joints: the role of torsion in the production of disc degeneration. J Bone Joint Surg [Am]. 1970;52:468-497.
31 Jiang H, Russell G, Raso J, et al. The nature and distribution of human supraspinal and interspinal ligaments. Spine. 1995;20:869-876.
32 Panjabi MM, Abumi K, Durancaeu J, et al. Spinal stability and intersegmental muscle forces: a biomechanical model. Spine. 1989;14:194-199.
33 Nachemson A, Evans J. Some mechanical properties of the third lumbar interlaminar ligament (ligamentum flavum). J Biomech. 1968;1:211-220.
34 Crisco JJIII, Panjabi MM. The intersegmental and multisegmental muscles of the lumbar spine: a biomechanical model comparing lateral stabilizing potential. Spine. 1991;16:793-799.
35 Macintosh JE, Pearcy MJ, Bogduk N. The axial torque of the lumbar back muscles: torsion strength of the back muscles. Aust NZ J Surg. 1993;63:205-212.
36 Gracovetsky S, Farfan HF, Helleur C. The abdominal mechanism. Spine. 1985;10:317-324.
37 Tesh KM, Dunn JS, Evans JH. The abdominal muscles and vertebral stability. Spine. 1987;12:507-508.
38 Hodges PW, Richardson A. Inefficient muscular stabilization of the lumbar spine associated with low back pain. Spine. 1996;21:2640-2650.
39 Bogduk N. Clinical anatomy of the lumbar spine and sacrum, 3rd edn., New York: Churchill Livingstone; 1997:67-69.
40 Peck D, Buxton DF, Nitz A. A comparison of spindle concentrations in large and small muscles acting in parallel combinations. J Morphol. 1984;180:243-252.
41 Bogduk N, Macintosh JE, Pearcy MJ. A universal model of the lumbar back muscles in the upright position. Spine. 1992;17:897-913.
42 Macintosh JE, Bogduk N. The biomechanics of the lumbar multifidus. Clin Biomech. 1986;1:205-213.
43 McGill SM, Juker D, Kropf P. Quantitative intramuscular myoelectric activity of quadratus lumborum during a wide variety of tasks. Clin Biomech. 1996;11:170-172.
44 Bartelink DL. The role of abdominal pressure in relieving the pressure on the lumbar intervertebral discs. J Bone Joint Surg [Br]. 1957;39:718-725.
45 McGill SM, Norman RW. Potential of lumbodorsal fascia forces to generate back extension moments during squat lifts. J Biomed Eng. 1988;10:312-318.
46 Gardner-Morse MG, Stokes IAF. The effects of abdominal muscle coactivation on lumbar spine stability. Spine. 1998;23:86-91.
47 Cresswell AG, Gundstrom H, Thorstensson A. Observations on intra-abdominal pressure and patterns of abdominal intramuscular activity in man. Acta Physiol Scand. 1992;144:409-481.
48 Gardner-Morse MG, Stokes IAF, Laible JP. Role of muscles in lumbar spine stability in maximum extensor efforts. J Orthop Res. 1995;13:802-808.
49 Luoto S, Taimela S, Hurri H, et al. Psychomotor speed and postural control in chronic low back pain patients: a controlled follow-up study. Spine. 1996;21:2621-2627.
50 Luoto S, Hurri H, Alaranta H. Reaction times in patients with chronic low-back pain. Eur J Phys Med Rehabil. 1995;5:47-50.
51 Nies N, Sinnott PL. Variations in balance and body sway in middle-aged adults: subjects with healthy backs compared with subjects with low-back dysfunction. Spine. 1991;16:325-330.
52 Hodges PW, Richardson CA. Contraction of the abdominal muscles associated with movement of the lower limb. Phys Ther. 1997;77:132-142.
53 Olerud S, Sjostrom L, Karlstrom G, et al. Spontaneous effect of increased stability of the lower lumbar spine in cases of severe chronic back pain. The answer of an external transpeduncular fixation text. Clin Orthop. 1986;203:67-74.
54 Panjabi MM, Lydon C, Vasavada A, et al. On the understanding of clinical instability. Spine. 1994;19:2643-2650.
55 Hazlett JW, Kinnard P. Lumbar apophyseal process excisions and spinal instability. Spine. 1982;7:171-176.
56 White AA, Panjabi MM. Clinical biomechanics of the spine, 2nd edn. Philadelphia: JB Lippincott, 1990.
57 Boden SD, Weisel SW, Laws EJr, et al. The aging spine. Philadelphia: WB Saunders, 1991.
58 Boden SD, Frymoyer JW. Segmental instability: overview and classification. In Frymoyer JW, editor: The adult spine: principles and practices, 2nd edn., New York: Raven Press, 1997.
59 Mirkovic S, Garfin SR. ‘Segmental’ instability as related to the degenerative disc. Semin Spine Surg. 1991;3:119-123.
60 Vo P, MacMillan M. The aging spine: clinical instability. South Med J. 1994;1987:S26-S35.
61 Macnab I. The traction spur: an indicator of segmental instability. J Bone Joint Surg [Am]. 1971;53:663-670.
62 Knutsson F. The instability associated with disk degeneration in the lumbar spine. Acta Radiol. 1944;25:593-609.
63 Arkin AM. The mechanism of rotation in combination with lateral deviation in the normal spine. J Bone Joint Surg [Am]. 1950;32:180-188.
64 Dupuis P, Yong-Ming K, Cassidy D, et al. Radiological diagnosis of degenerative spinal instability. Spine. 1985;10:262-276.
65 Frymoyer JW, Hanley ENJr, Howe J, et al. A comparison of radiographic findings in fusion and nonfusion patients, ten or more years following lumbar disc surgery. Spine. 1979;4:435-440.
66 Lindahl O. Determination of the sagittal mobility of the lumbar spine. Acta Orthop Scand. 1966;37:241-254.
67 Bernhardt M, White AAIII, Panjabi MM, et al. Biomechanical considerations of spinal stability. In: Rothman RH, Simeone FA, editors. The spine. Philadelphia: WB Saunders; 1992:1167-1196.
68 Rutkow IM. Orthopaedic operations in the United States, 1979 through 1983. J Bone Joint Surg [Am]. 1986;68:716-719.
69 Hayes MA, Howard TC, Gruel CR, et al. Roentgenographic evaluation of lumbar spine flexion–extension in asymptomatic individuals. Spine. 1989;14:327-381.
70 Spratt KF, Weinstein JN, Lehmann TR, et al. Efficacy of flexion and extension treatments incorporating braces for low back pain patients with retrodisplacement, spondylolisthesis, or normal sagittal translation. Spine. 1993;18:1839-1849.
71 Shaffer WD, Weinstein J. Segmental spinal instability. A survey of measurement techniques. Semin Spine Surg. 1991;3:124-149.
72 Newman PH, Stone KM. The etiology of spondylolisthesis. J Bone Joint Surg [Br]. 1963;45:39-59.
73 Grubb SA, Lipscomb MJ, Conrad RW. Degenerative adult-onset scoliosis. Spine. 1988;13:241-245.
74 Hayes MA, Tompkins SF, Herndon WA, et al. Clinical and radiological evaluation of lumbosacral motion below fusion levels in idiopathic scoliosis. Spine. 1988;13:1161-1167.
75 Nachemson AL. The lumbar spine: an orthopaedic challenge. Spine. 1976;1:59-71.
76 O’Sullivan PB. The efficacy of specific stabilizing exercise in the management of chronic low back pain with radiological diagnosis of lumbar segmental instability. PhD thesis, Curtin University of Technology, Western Australia, 1997.
77 Paris S. Physical signs of instability. Spine. 1985;10:277-278.
78 O’Sullivan PB. Lumbar segmental instability: clinical presentation and specific stabilizing exercise management. Manual Therapy. 2000;5:2-12.
79 O’Sullivan P, Twomey L, Allison G. Dynamic stabilization of the lumbar spine. Crit Rev Phys Rehab Med. 1997;9:315-330.
80 O’Sullivan P, Twomey L, Allison G. Evaluation of specific stabilizing exercise in the treatment of chronic low back pain with radiological diagnosis of spondylolysis and spondylolisthesis. Spine. 1997;15:2959-2967.
81 Richardson C, Jull G. Muscle control – pain control. What exercise would you prescribe? Manual Therapy. 1995;1:2-10.
82 O’Sullivan P, Twomey L, Allison G, et al. Specific stabilizing exercise in the treatment of chronic low back pain with clinical and radiological diagnosis of lumbar segmental instability. Third Interdisciplinary World Congress on Low Back Pain and Pelvic Pain, Vienna, Austria, 1998.
83 Shumway-Cook A, Woollacott M. Motor control – theory and practical applications. Baltimore: Williams & Wilkins, 1995.
84 O’Sullivan P, Twomey L, Allison G. Altered abdominal muscle recruitment in back patients following specific exercise intervention. J Orthop Sports Phys Ther. 1998;27:1-11.