Chapter 35 Hemodynamic Aspects of Cardiac Pacing
Heart Rate: Restoration of Normal Chronotropic Function
Cardiovascular Physiology
Cardiac output (CO) is the product of heart rate (HR) and stroke volume (SV), calculated as the difference between left ventricular (LV) end-diastolic (ED) and end-systolic (ES) volumes. HR is mainly under the influence of the autonomic nervous system and circulating catecholamines. LVED volume depends on the (1) circulating volume, (2) cardiac filling pressures, (3) contributions of atrial systole, and (4) ventricular relaxation, and LVES volume depends on (1) cardiac afterload and (2) ventricular contractility (Figure 35-1). The Frank-Starling law correlates the ventricular filling pressures with ventricular systolic ejection volumes as a function of the LV systolic performance (Figure 35-2).1 The respective contributions of these variables to cardiovascular performance are influenced by age; physical fitness; the presence, type, and severity of underlying heart disease; and the prescription of pharmaceuticals. In healthy individuals, during exercise, up to a 300% increase in CO is mostly contributed by an increase in HR and more modestly to an increase in stroke volume (SV).2 On the one hand, the contribution of the increase in SV is more important in endurance athletes. In the presence of LV systolic dysfunction, on the other hand, the increase in SV during exercise is limited by a reduced contractile reserve, and the increase in CO is even more dependent on variations in HR.
Chronotropic Dysfunction
Chronotropic Incompetence
The term chronotropic incompetence (CI) describes an insufficient HR relative to the metabolic needs of the organism, during exercise or under stress caused by emotional disturbance, pain, and so on. The definition of CI has not been standardized. It has been defined as the inability to reach, during an exercise test, a maximal HR more than 75% to 80% of the theoretical maximal HR calculated by Astrand’s formula (220 – Age). Others consider that the absolute peak HR during exercise must be more than 100 or 120 beats/min.3 Wilkoff determined the HR response to exercise in a mathematical model, which includes age, resting HR, intensity of exercise, and functional capacity (FC) in the equation:
The incidence of CI varies with the definition applied, though it may reach 60% among permanently paced patients. Its origin is atrial or ventricular. Sinus node dysfunction (SND) is the most frequent cause of atrial CI, observed in 25% to 40% of cases, and the incidence increases with age.4 A few patients presenting with permanent atrial fibrillation maintain a normal chronotropic function during exercise. However, they have inappropriate tachycardia, bradycardia, or both in alternans. The severity of CI in patients presenting with high-degree AV block increases with age and with the increasingly distal location of a block along the His-Purkinje system. Rarely dissociated from disorders of impulse formation or propagation, CI may be associated with autonomic dysfunction, as well as with myocardial ischemia.5 Further investigation is warranted if myocardial ischemia is suggested by clinical presentation, particularly since its incidence is threefold higher among patients with coronary artery disease.
Optimal Heart Rate
Lower Ventricular Pacing Rate
In the early days of cardiac pacing, Sowton et al studied the optimal lowest pacing rate by measuring CO and left and right filling pressures in patients presenting with complete AV block.6 The highest CO and lowest filling pressures were associated with pacing rates between 55 and 90 beats/min and a mean of 71 beats/min. CO/ventricular pacing rate curves showed roughly two distinct profiles. Flat curves (Figure 35-3, left) were most often associated with normal myocardial contractility and a stable CO as HR increased. Peaking curves (see Figure 35-3, right) were often observed in the presence of LV dysfunction, showing greater variations in CO with changes in HR. At the fastest HR, CO levels fell, reflecting the shortened diastolic filling time and alteration in LV compliance.
Maximal Ventricular Pacing Rate
The upper ventricular pacing rate, whether atrial tracking driven or sensor driven, must also be tailored on the basis of the patient’s habitual activities and underlying cardiovascular status. An excessively slow upper pacing rate causes exercise-induced chronotropic insufficiency, and an excessively rapid pacing rate may be deleterious. Kindermann et al ascertained the theoretical, optimal, maximal pacing rate corresponding to the increase in maximum VO2 (oxygen rate) measured during cardiorespiratory exercise testing in patients with a normal or less than 45% LV ejection fraction (EF), the majority of whom were paced in DDD mode.7 The mean optimal pacing rate was 86% of the theoretical maximal HR in patients with a normal LVEF, and 75% in patients with a depressed LVEF. It is noteworthy that programming of the upper pacing rate was excessively high in the majority of patients. Furthermore, in most patients, maximal VO2 rapidly reached a plateau during exercise testing, regardless of systolic LV function. According to Fick’s principle, a VO2 that remains stable despite an increase in HR (in presence of a stable arteriovenous O2 difference) indicates a decrease in SV.
Preserving Atrial Function and Atrioventricular Synchrony
Atrial Function
The hemodynamic importance of atrial function varies highly among individuals. In healthy subjects, the contribution of atrial systole to ventricular SV is approximately 20% at rest and 30% during exercise. The contribution of atrial systole to CO during vigorous effort is gradually supplanted by HR. However, the relative importance of atrial systole increases with age, with abnormal ventricular diastolic function, and within certain limits, with the deterioration of LV systolic function.8
Atrioventricular Synchrony
A proper AV synchronization is key for the efficacious hemodynamic contribution of atrial systole. Conversely, complete AV dissociation is hemodynamically detrimental. Atrial systole occurring when the mitral valve is closed activates (1) atrial and pulmonary neurohumoral stretch receptors, (2) vagal activity, and (3) the production of atrial natriuretic peptide. These effects may cause a fall in systemic arterial pressure and a variety of manifestations known as pacemaker syndrome when occurring in permanently paced patients. It has been described in approximately 20% of patients paced in the VVI/VVIR mode, whose atrial activity is sinus, though it may occur in any pacing mode in the presence of AV dyssynchronization. AV synchronization is electrical and mechanical, left and right. While electrical and mechanical dyssynchronies usually coexist, their correlation is not systematic (see Atrial Conduction Delay). “Electrical” AV dyssynchrony manifests as an abnormal PR interval or AV dissociation on surface ECG, and “mechanical” AV dyssynchrony is defined as a LV diastolic filling time less than 40% of the cardiac cycle, accompanied by an abnormal transmitral Doppler profile. In the case of cardiac pacing, left AV mechanical synchrony is hemodynamically the most important and is preserved by programming an appropriate AV delay. The main determinants of the AV delay are AV conduction time, intra-atrial and inter-atrial conduction times, and intraventricular and interventricular (VV) conduction times.
Atrial Conduction Delay
The intra-atrial and inter-atrial conduction delays are measured from the onset of the P wave to (1) the para-Hisian atrial ECG (normally 30 to 60 ms), and (2) to the distal coronary sinus atrial ECG (normal 60 to 90 ms), respectively. Prolonged atrial conduction delays may cause left AV dyssynchrony with a foreshortened mechanical AV delay, apparent on mitral Doppler as a short LV filling period and truncated A wave. The main causes of prolonged atrial conduction delays—apparent on surface ECG as a more than 120-ms P-wave duration, notching of the P wave, and a foreshortened segment between the end of the P wave and the onset of QRS (Figure 35-4)—are (1) slowing of intra-atrial conduction due to heart disease, and (2) a latency of paced atrial capture. The latter is responsible for greater conduction delays than is apparent during sinus rhythm and mandates the routine programming of a 30 ms to 40-ms longer paced than sensed AV delay. The latency of atrial capture can be considerably amplified by several factors, including type and location of the pacing lead and its interface with the myocardium, pacing rate, abnormal electrolyte or metabolic status, the effect of medications, and myocardial disease. Heart diseases most often associated with anomalous atrial conduction are valvular (rheumatic mitral disease, in particular), hypertrophic, ischemic, and hypertensive. Programmers allow a choice of separate AV intervals for sensed P waves versus paced P waves in order to offset this phenomenon. While left atrial dilation is often associated with prolonged atrial conduction time, this correlation is low, except in the case of rheumatic mitral valve disease.9
Prevention and Management of Atrial Conduction Delay
Late Atrial Sensing and Cardiac Resynchronization Therapy
The clinical effects of fused spontaneous and paced QRS in recipients of CRT systems remain uncertain. Indirect arguments suggest that fusion complexes are not necessarily adverse.11 In limited studies, at least a similar hemodynamic benefit was observed in patients at rest in whom resynchronization was achieved by LV stimulation alone, with fusion via the right bundle branch, or by biventricular stimulation.12 Furthermore, the absence of first-degree AV block predicts a response to CRT. Either the presence of AV block is a prognostic factor associated with more severe heart disease or its absence is associated with a higher likelihood of fusion or “concealed resynchronization.”13 Nevertheless, pending further studies, the current recommendation is to avoid or limit biventricular simulation with fusion to the extent possible.
Atrioventricular Synchrony During Exercise
During exercise, sympathetic activity facilitates AV nodal conduction, and a shortening of AV delay that is inversely proportional to the HR is observed. Implantable pacemakers are able to reproduce this phenomenon in patients with AV block.14 The rate-adaptive AV delay shortens AV delay, usually linearly with acceleration of the HR, up to the shortest programmable value.15 This offers several benefits: (1) improvements in hemodynamic function and cardiopulmonary performance while preserving proper AV synchrony during exercise16; (2) ability to increase the maximal synchronous HR during exercise by shortening TARP; and (3) subjective improvement in the quality of life by the adaptation of the AV delay during exercise.17
Conflicting data have been reported regarding the programming of hemodynamically optimal AV delays in recipients of CRT systems during effort, probably reflecting heterogeneous patient characteristics, including degree and type of dyssynchrony, lead positions, and underlying heart disease.18 The current recommendation is to deactivate the rate-adaptive AV delay in the absence of AV conduction disorder.
Ventricular Conduction Delay and Atrioventricular Synchrony
An additional delay is inserted between left atrial and LV activation in cases of marked slowing of intraventricular or VV conduction with left bundle branch block morphology, most often observed in the context of advanced heart disease. Despite the absence of PR interval abnormality of surface ECG (Figure 35-7), unequivocal left AV mechanical dyssynchrony is present on ultrasound Doppler examination (Figure 35-8). Although conventional DDD pacing with a short AV delay can correct LV filling (transmitral Doppler), it should not be programmed in patients with HF because of the potential adverse effects of RV pacing. Moreover, this pacing increases only left heart filling, whereas biventricular stimulation restores AV synchrony on both sides of the heart (see Figure 35-17) as well as intraventricular synchrony and improves clinical outcomes.
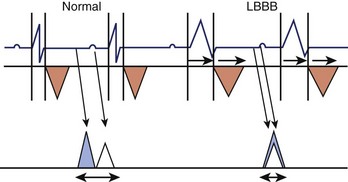
Figure 35-8 Effect of left bundle branch block (LBBB) on AV synchrony. Left ventricular outflow tract (orange triangles) and mitral Doppler (blue triangle, E wave; white triangle, A wave) are shown during the cardiac cycle. LBBB is responsible for an increased systolic and decreased diastolic interval (see also Figure 35-15) with fusion of A and E waves. The P-R interval is unchanged.
(Modified from Cazeau S, Gras D, Lazarus A, et al: Multisite stimulation for correction of cardiac asynchrony, Heart 84:579–581, 2000.)
Atrioventricular Optimization in DDD/DDDR Pacing
The purpose of an optimal AV delay is to guarantee (1) the maximal contribution of the atrium to the diastolic LV filling, (2) a long diastolic filling period, (3) a short isovolumetric contraction period, and (4) the maximal SV. Empirical programming is suboptimal because of the wide variability among patients in optimal AV delay, caused by marked interindividual differences in electrical and electromechanical intervals in the atria (intra-atrial and inter-atrial conduction times) and ventricles (VV conduction delay, in particular). In case of an excessively long AV delay, LV contraction occurs well after atrial systole, while the mitral valve is passively semi-closed, causing (1) fusion of E and A waves of the filling transmitral Doppler flow and (2) diastolic mitral regurgitation (Figure 35-9). An excessively short AV delay causes the LV contraction to close the mitral valve before the end of ventricular filling (truncated A wave). AV delay is optimized mostly in recipients of CRT systems, whereas in dual-chamber pacing, it is reserved for the rare patients presenting with symptoms attributed to AV dyssynchrony. Several methods are applicable, depending on the choices and practices of the various centers (Table 35-1). Echocardiography and programming algorithms are most often used because of their wide availability, ease of use, and low cost. Other methods are mostly investigative and will not be discussed in detail in this chapter (see Table 35-1).
Methods | Description |
---|---|
ECHOCARDIOGRAPHY | |
Optimization of Left Ventricular Filling | |
Vopt, Optimal AV delay; AV, AV delay; long QA, interval between Q of the electrocardiogram to end of mitral Doppler A wave with long AV delay; short QA, interval between Q of the electrocardiogram to end of mitral Doppler A wave with short AV delay; VTI, velocity-time integral.
Atrioventricular Optimization Methods
Ritter described an AV delay optimization formula applicable to recipients of DDD pacemakers presenting without LV dysfunction and complete AV block.19 This method allows the calculation of AV interval with a ventricular contraction immediately following the deceleration phase of atrial contraction. Its advantages are its simplicity and expeditious application (see Table 35-1). However, its use should be avoided in patients with preserved AV conduction. In recipients of the CRT system, Ritter’s formula was shown to produce hemodynamic results that were inferior to those obtained with optimization of AV delay using aortic or mitral velocity-time integral (VTI).20 Meluzin et al have also described a simple method for patients undergoing CRT.21 It requires the presence of mitral regurgitation and estimates an optimal AV delay that is closely correlated with CO.
Optimizing AV delay on the basis of aortic VTI, mitral VTI, or the iterative method (see Table 35-1, Figure 35-10) showed immediate increases in CO or LV dP/dt (first derivative of pressure measured over time) in recipients of CRT systems. However, these methods are time consuming and poorly reproducible. In a comparison of mitral VTI with diastolic filling time, aortic VTI, or Ritter’s formula, Jansen et al found that the maximal mitral VTI produced the greatest increase in LV contractility, measured by dP/dt.22
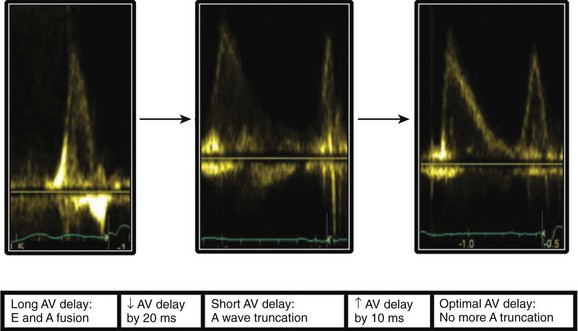
Figure 35-10 Iterative echocardiographic optimization of atrioventricular (AV) delay.
(Courtesy Dr. E. Donal, University Hospital of Rennes, France.)
Limitations of Atrioventricular Optimization
Several limitations have been identified in the optimization of AV delay. First, it varies among methods used, particularly between methods using optimization of ventricular filling and optimization of systolic function. Second, the conditions in which AV delay is usually optimized (at rest, in the decubitus position) do not reflect the real-life conditions of patients who have maintained some level of physical activity. Furthermore, despite several single-center studies reporting hemodynamic benefits conferred by optimization of AV delay, the evidence that it improves the clinical outcomes of the recipients of CRT systems is slim. In a single-center, randomized study of AV delay optimization, in which aortic VTI was used 3 months after implantation of the CRT system, Sawhney et al observed a more than 1-point decrease in New York Heart Association (NYHA) HF functional class in 75% of patients who underwent the optimization procedure compared with 45% of patients who did not undergo the procedure.23
Another challenge in the optimization of AV delay are the prominent variations during long-term follow-up, in particular because of the changes in loading conditions caused by LV reverse remodeling.24 No definitive consensus has been reached with respect to the preferred time of optimization or the long-term schedule of re-evaluations. A systematic initial optimization as well as repeated procedures, while desirable, are often time consuming and hence precluded in clinical practice. A quick initial evaluation of the morphology of the transmitral flow on Doppler ultrasound is an alternative method of confirming the absence of prominent AV dyssynchrony.25 AV delay must, nevertheless, be optimized in all nonresponders to CRT.
Optimization of Ventricular Function: Role of the Ventricular Pacing Site
Right Ventricular Apical Stimulation
Hemodynamic Consequences
Acute Effects
The RV apex is the most often chosen pacing site for ease of lead placement, its stability, and predictably low capture threshold. It is, however, the cause of a left bundle branch block–like ventricular activation sequence and electrical and structural remodeling. In 1925, Wiggers et al found that RV apical pacing decreased LV dP/dt and caused cardiac dyssynchrony. They proposed a slowing of intramyocardial conduction, compared with normal propagation through the His-Purkinje system. Since then, multiple studies have confirmed the immediate adverse hemodynamic effects of apical pacing, with or without preservation of AV synchrony.26 After cessation of ventricular pacing, abnormalities of repolarization (cardiac memory) persist for several days. The incompletely understood mechanisms implicate short-term changes in ionic channels and protein phophorylation and longer-term changes in gene expression and protein synthesis. Abnormal myocardial relaxation and a transient decrease in LVEF accompany these abnormalities of repolarization for a few hours or days after cessation of apical pacing.
Permanent Pacing
In the long term, apical RV pacing may cause major structural changes (Table 35-2), including ventricular dilation and asymmetric hypertrophy. SV and LV dP/dt are decreased, and the pressure–volume curve is shifted to the right. The LV volume is increased by both dilation and hypertrophy, and its performance depends on the Frank-Starling law to a greater extent. Short-term and long-term follow-up studies have shown an approximately 10% decrease in LVEF. Furthermore, shortening of the ventricular filling time and maximal rate of fall of LV pressure (–dP/dt) are manifestations of diastolic dysfunction. Mitral regurgitation sometimes develops or worsens, probably due to a decrease in the transmitral gradient (forces of valve closure) and in the synchrony of papillary muscles contraction.27 Asymmetric hypertrophy may become visible in the late-activated zones, reflecting inhomogeneous myocardial work and a greater parietal tension at those sites at the onset of ventricular systole. A redistribution of myocardial sympathetic innervation during RV pacing is another cause of asymmetric hypertrophy caused by the focal release of catecholamines. On histologic examination of these zones, myocytes appear hypertrophied and disarrayed, without any increase in capillary density, which results in a decreased coronary reserve. Dilation and hypertrophy further increase ventricular dyssynchrony and decrease LV systolic function, thereby initiating a vicious circle.
Table 35-2 Adverse Effects of Abnormal Ventricular Activation During Right Ventricular Apical Pacing
Hemodynamic function | Depressed left ventricular systolic and diastolic functions |
Reduced cardiac output | |
Increased filling pressure | |
Ventricular dyssynchrony | Interventricular dyssynchrony |
Intraventricular dyssynchrony | |
Remodeling | Asymmetric hypertrophy |
Left ventricular dilation | |
Valvular function | Functional mitral regurgitation |
Myocardial perfusion | Changes in regional myocardial perfusion and demand |
Autonomic nervous system | Changes in regional innervation |
Ventricular Pacing and Clinical Outcomes
Risk of Heart Failure
In patients free from structural heart disease, the prevalence of ventricular dyssynchrony induced by RV apical pacing is between 30% and 60% and is associated with alterations of systolic and diastolic functions. Dyssynchrony due to apical pacing may persist after its cessation.28 The decrease in LVEF is often modest29; only a fraction of patients develop more prominent ventricular dysfunction and overt, long-term HF, rarely (3% to 10%) before at least 3 years of permanent pacing.30 The prevalence, however, increases over time. After a mean follow-up of nearly 8 years, Zhang et al observed the development of new-onset HF in 26% of patients permanently paced for AV block.31
The risk of developing HF is considerably higher and its onset earlier in patients whose LVEF is already depressed or who have a history of HF or myocardial infarction. This risk is probably caused by slower propagation of the electrical wavefront and more prevalent and more prominent ventricular dyssynchrony in these patients than in those without heart disease.32 Studies of the relationship between dyssynchrony apparent shortly after device implantation and long-term outcomes are in progress.
Pacing Mode and Clinical Outcomes
Several pacing characteristics and their consequences, including percentage and duration of ventricular pacing, pacing mode with respect to conduction abnormalities, site of ventricular stimulation, and duration of the paced QRS, also determine the development of HF.33 An increase in the paced QRS duration in patients with SND or AV block is associated with an increased likelihood of development of HF.34 This association may be explained by a more prominent mechanical ventricular dyssynchrony at some pacing sites, though it might also be a manifestation of more severe myocardial and conduction system disease.
Choosing the right pacing mode is crucial. In the DAVID trial, which enrolled patients presenting with severe LV dysfunction and a dual-chamber implantable cardioverter defibrillator (ICD), the incidence of hospitalizations for management of HF was higher among those paced in the DDDR mode, at a basic pacing rate of 70 beats/min with a short AV delay, than in patients paced in the VVI mode, at a backup rate of 40 beats/min, which was associated with a higher percentage of ventricular pacing in the former group.35
Furthermore, a post hoc analysis of the Mode Selection Trial (MOST) (Figures 35-11 and 35-12) revealed that in patients paced in DDDR or VVIR for SND, both the rates of atrial fibrillation and hospitalizations for HF increased proportionally to the percentage of ventricular pacing.36,37 This underscored the importance of limiting RV apical pacing and the risk of loss of benefits conferred by pacing modes that preserve AV synchrony.
Cardiac Pacing and Obstructive Hypertrophic Cardiomyopathy
Despite its attractive premise, RV apical stimulation for OHCM has yielded mixed results. The PIC trial observed a 50% decrease in left intraventricular gradient and a 20% increase in exercise capacity, though the alleviation of dyspnea and chest pain was similar in DDD and AAI modes, suggesting a placebo effect.38 Except in a subgroup of patients older than 65 years, the Multicenter Pacing Therapy (M-PATHY) trial found neither subjective nor objective differences in exercise capacity between RV-paced patients versus non–RV-paced patients.39 In the absence of more convincing evidence—and given the availability of other, more effective treatments, including surgery or even alcohol ablation of the septum—the role played by cardiac stimulation in OHCM is currently limited (class IIb recommendation in professional guidelines).40
Preventing Unnecessary Ventricular Stimulation
Pacing Mode Selection
The influence of RV pacing minimization algorithms on clinical outcome is still controversial. The randomized INTRINSIC RV study, which included recipients of ICDs, programmed either in the VVIR mode with a backup rate set at 40 beats/min or in the DDDR mode with the AV hysteresis algorithm, found no difference in clinical outcomes between the two groups.41 The SAVE PACe study, which enrolled patients with SND paced in the DDDR mode with or without an algorithm of RV pacing minimization, found a 9.1% median percentage of ventricular pacing with the algorithm compared with 99% without the algorithm. While a 40% decrease in relative risk in the incidence of persistent AF was observed, the rates of death and hospitalizations related to HF were similar in both groups.42
Alternative Right Ventricular Pacing Sites
When permanent ventricular capture is needed, the use of alternative RV pacing sites should be considered to prevent the adverse effects of RV apical pacing and improve ventricular function. The technical feasibility and safety of implanting the RV lead onto the septum have been confirmed in several studies. The short-term to intermediate-term capture threshold, impedance, and sensing characteristics of leads implanted in the apical, septal, or RV outflow tract (RVOT) positions are similar.43,44 The mean procedural times, complication rates, and risks of lead dislodgment are also similar. The risk of perforation seems low, and the extraction procedures are of similar complexity. The results of studies that compared the short-term and long-term hemodynamic benefits conferred by alternative RV pacing sites versus apical pacing are conflicting for various reasons, including the imprecise definition of the site of lead implant (RVOT versus septum), heterogeneous patient populations and evaluation methods, and, sometimes, insufficient duration of follow-up.45
Right Ventricular Upgrade
CRT has been used as an upgrade for patients presenting with severe LV dysfunction and worsening HF due to RV pacing. Several small controlled studies have documented improvements in invasively measured hemodynamic function conferred by CRT upgrades, as well as the alleviation of ventricular dyssynchrony and mitigation of LV remodeling (decrease in ED and ES diameters), increase in LVEF, and decrease in NYHA functional class and increase in distance covered during 6-min walk.53,54 The effects on survival remain unknown, and long-term clinical results have been mixed. Several multicenter studies are ongoing to further address these uncertainties.55
Left Ventricular and Biventricular Stimulation
Optimal Left Ventricular Stimulation Site
In contrast to the implantation of the previously described LV lead, optimal placement of the RV lead in biventricular stimulation is poorly understood. Lecoq et al have analyzed the various electrical characteristics associated with CRT.56 The placement of the RV lead was modified to obtain the thinnest QRS after the implantation of the LV lead. A midseptal position was chosen in the majority of cases. Only the shortening of the QRS (not the baseline QRS duration) predicted the response to CRT. However, the predictive value of QRS shortening remains controversial, and more detailed information regarding the placement of the RV lead is awaited.57
Left Ventricular Epicardial Stimulation Versus Endocardial Stimulation
The original intent to stimulate the LV endocardial surface was based on the normal endocardium-to-epicardium electrical activation sequence, which is associated with a speedy and synchronous ventricular depolarization (Figure 35-13). Van Deursen et al found, in an animal model, a shorter ventricular depolarization and less dispersion of repolarization with endocardial stimulation than with epicardial biventricular stimulation.58 They also observed an acute hemodynamic benefit (Figure 35-14), which seemed less dependent on site or timing than with epicardial stimulation. Derval et al compared invasive and noninvasive acute hemodynamic measurements made in 35 patients presenting with nonischemic, dilated cardiomyopathies during stimulation from 10 endocardial and 1 epicardial LV sites and found wide interindividual variability in the hemodynamically optimal stimulation site.59 Stimulating at the optimal site increased LV dP/dt significantly, compared with (1) standard LV epicardial stimulation via the coronary venous system, (2) lateral LV endocardial wall stimulation, or (3) stimulation at the latest site of LV activation ascertained echocardiographically. Stimulation of the endocardial site immediately opposite to the transvenously identified epicardial site had no significant effects on either dP/dtmax or LVES pressure. However, compared with epicardial stimulation, endocardial stimulation improved LV diastolic function, as ascertained by measurements of LV – dP/dt.
Trans-aortic, trans-septal, and trans-apical approaches have been described for permanent LV endocardial stimulation in humans. The first feasibility study was carried out by atrial trans-septal lead implantation, by Jais et al.60 In several studies of small numbers of typical CRT system recipients, biventricular endocardial stimulation lowered NYHA functional class and increased LVEF. These data, based on small numbers of observations, need additional studies to clarify the risk/benefit ratio from the perspectives of long-term anticoagulation and complications associated with the various lead implantation techniques.
Single Left Ventricular Stimulation
CRT using a single LV lead is based on the expectation of fusion between stimulated LV and spontaneous RV depolarizations, resulting in a synchronous biventricular activation sequence (see Figure 35-20). Immediate hemodynamic and clinical improvements were observed in typical candidates for CRT. Furthermore, a fused QRS seemed to be associated with a higher LV dP/dtmax. Device Evaluation of CONTAK RENEWAL 2 and EASYTRACK 2: Assessment of Safety and Effectiveness in Heart Failure (DECREASE-HF), a randomized study, compared the 6-month echocardiographic outcomes in patients who underwent (1) synchronous biventricular, (2) sequential biventricular, or (3) single-site LV stimulation.61 A trend toward a greater decrease in LV dimensions with biventricular LV stimulation than with single-site LV stimulation was observed. In addition, mitral regurgitation worsened in 18% of patients who underwent single-site LV stimulation, perhaps because of greater LV dilation and less complete resynchronization, since ventricular fusion complexes were not required in that study. Furthermore, this means of stimulation is not applicable to ICD recipients or pacemaker-dependent patients, who need an RV lead, because of the notorious instability of the LV epicardial leads and the high capture threshold often associated with them. Finally, despite the availability of a dynamic AV delay function, the programming of synchronous LV-RV activation during spontaneous variations in AV conduction may be challenging, for example, during exercise.
Multiple-Site Left Ventricular Stimulation
CRT using a single LV lead may be insufficient to produce optimal ventricular resynchronization, particularly in the presence of prominent LV dilation. It has been hypothesized that stimulating the LV at two or more distant sites would further improve LV performance and increase the rate of response to CRT. While the feasibility and safety of this strategy has been confirmed, it requires longer procedures and fluoroscopic exposure. In experienced hands, the procedural success rate approaches 85%. In short-term hemodynamic studies in patients with HF, Pappone et al found that dual-site LV stimulation increases LV dP/dt and shortens QRS duration compared with single-site stimulation.62 In contrast, Padeletti et al observed no further hemodynamic improvement conferred by CRT delivered via dual-site LV stimulation compared with single-site LV stimulation.63 The multicenter, single-blind, crossover TRIP HF trial included 40 patients who had indications for both CRT and for ventricular pacing because of atrial fibrillation and slow ventricular response.64 After 3 months of biventricular stimulation, the patients were randomly assigned to biventricular stimulation versus triple-site ventricular stimulation; then they crossed over to the alternative assignment for three additional months. While no difference was observed in clinical outcomes between the two groups, a significantly greater decrease in LVED diameter and increase in LVEF were observed during triple-site ventricular stimulation. Furthermore, 40% of nonresponders to biventricular stimulation (ascertained on the basis of LVES diameter) became responders during dual-site LV stimulation.
Cardiac Resynchronization Therapy
Rationale and Mechanisms
Abnormal intraventricular conduction, usually of left bundle branch block morphology, is present in 30% of patients presenting with severe LV systolic dysfunction. This causes asynchronous LV contraction, a decrease in SV, and ventricular remodeling. Ventricular activation propagates from the right ventricle to the septum and then to the LV free wall. The duration of contraction and isovolumetric relaxation are lengthened, and the ventricular diastolic filling time is shortened proportionally to the degree of ventricular dyssynchrony and QRS duration (Figure 35-15).65 CRT simultaneously stimulates the LV free wall and the right ventricle during ventricular systole, fusing the two activation wavefronts, improving electrical (narrow QRS) and mechanical (synchronous segmental contraction) synchrony, according to the rapid electromechanical coupling of the myocardium.
Short-Term Hemodynamic Effects
In patients presenting with HF and left bundle branch block, Leclercq et al observed an immediate hemodynamic improvement during biventricular stimulation compared with spontaneous conduction or DDD RV apical pacing (Figure 35-16).66 Instead of an increase in ventricular preload, the increase in CO was caused by an increase in myocardial contractility, since the filling pressures had decreased during CRT. Other studies confirmed improvements in hemodynamic function, which manifested as increases in LV dP/dtmax, LVEF, and pulse pressure, and in LV diastolic function, which manifested as a longer ventricular filling time, higher –dP/dt, and lower filling pressures.
Multiple mechanisms are behind these hemodynamic benefits. On the one hand, CRT resynchronizes at the AV, VV, and intraventricular levels, with the main hemodynamic benefit brought about by the correction of LV activation and resynchronization of segmental contraction. On the other hand, as Nelson et al observed, a greater efficiency of the cardiac pump is achieved by LV stimulation, which decreases to a modest extent the myocardial consumption of energy.67 Improvement in VV coupling is another hemodynamically favorable and immediate mechanism of CRT (Figure 35-17).68 LV activation and onset of diastole are expedited and do not limit LV filling by the space occupied by the right ventricle inside the pericardium when the filling pressures are high.69
Long-Term Hemodynamic Effects
The hemodynamic benefits described earlier persist over the long term as well. Furthermore, as a result of improvements in LV pump function and lower filling pressures during CRT, the heart works with lower LV volumes (Figure 35-18).70 The decrease in wall tension limits the concomitant neurohumoral activation. These combined effects promote reverse remodeling (Figure 35-19), most reliably manifesting as a decrease in LVED diameter. The resynchronized contraction of the subvalvular apparatus and the smaller LV dimensions also facilitate the regression of functional mitral regurgitation. Yu et al observed the persistence of LV reverse remodeling for up to 1 month after the interruption of CRT.71
Both the morbidity and the survival benefits conferred by CRT are important. However, currently no evidence indicates that a hemodynamic improvement observed in the short term predicts a favorable long-term outcome. Mullens et al found that biventricular stimulation continues to acutely improve hemodynamic function in the failing heart in most patients, including those in whom cardiac remodeling has progressed during CRT and who are hospitalized for the management of HF.72 Mullens et al suggested that the progression of disease is not attributable to a diminishing hemodynamic benefit contributed by successful resynchronization. A decrease in LVES diameter is the marker of remodeling that best predicts long-term outcome, though this marker is imperfect since, among CRT recipients whose LVES diameter has decreased by more than 10%, approximately 30% do not derive any survival benefit. Finally, the absence of correlation between (1) functional characteristics such as NYHA functional class and quality of life and (2) survival or ventricular remodeling continues to complicate the identification of potential responders to CRT.73
Optimization of Ventricular Timing
Electrical Optimization
Attempts in some studies to obtain the thinnest QRS during CRT by changing the lead(s) position(s) has been associated with higher rates of therapeutic response, though this is still debated. This strategy is clearly limited by the presence of zones of slow conduction situated near the stimulation site, which might conceal components of the QRS, rendering them isoelectric and spuriously shortening the duration of the complex. The morphology of fusion complexes may also help in assessing the effects of CRT. A QRS identical to that produced with left-sided or right-sided stimulation raises the suspicion of conduction delays and latency of capture at the right-sided and left-sided leads, respectively. The programming of sequential LV-RV stimulation may allow a more synchronous activation of the ventricles (Figure 35-20). A change in the bipolar, extended bipolar, or inverted bipolar LV stimulation vector is effective in cases of high capture threshold or phrenic stimulation. The clinical merits of such programming remains to be shown.
Echocardiographic Optimization
In advanced heart disease, intraventricular and interventricular conduction delays are sometimes markedly lengthened, and a sequential activation of the ventricles may be necessary to achieve the best resynchronization. Echocardiography is the most commonly applied method of VV delay optimization, mostly based on aortic VTI as a surrogate measurement of LVEF. It is an iterative method, which consists of changing AV delay while searching for the highest VTI. Despite immediate improvements in LV dP/dt, SVEF, and LVEF conferred by optimization of VV delay, the multicenter Insync III Marquis (Medtronic, Minneapolis, MN), DECREASE-HF, and Resynchronization for Hemodynamic Treatment for Heart Failure Management II (RHYTHM II) ICD trials did not find clear clinical benefits compared with simultaneous biventricular stimulation.61,74,75 In clinical practice, in the absence of a proven clinical advantage, the systematic optimization of the VV interval is not recommended after implantation of CRT systems.
Barold B, Ilercil A, Herweg B. Echocardiographic optimization of the atrioventricular and interventricular intervals during cardiac resynchronization. Europace. 2008;10:iii88-iii95.
Daubert JC, Pavin D, Jauvert G, Mabo P. Intra- and interatrial conduction delay: Implications for cardiac pacing. Pacing Clin Electrophysiol. 2004;27:507-525.
Daubert C, Ritter P, Mabo P, et al. Physiological relationship between A-V interval and heart rate in healthy subjects: Applications to dual-chamber pacing. PACE. 1986;9:1032-1039.
Grines CL, Bashore TM, Boudoulas H, et al. Functional abnormalities in isolated left bundle branch block. The effect of interventricular asynchrony. Circulation. 1989;79:845-853.
Guyton AC, Hall JE. Textbook of medical physiology, ed 11. St. Louis: Elsevier; 2005.
Kappenberger L, Linde C, Daubert C, et al. Pacing in hypertrophic obstructive cardiomyopathy. A randomized crossover study. PIC Study Group. Eur Heart J. 1997;18:1249-1256.
Leclercq C, Cazeau S, Le Breton H, et al. Acute hemodynamic effects of biventricular DDD pacing in patients with end-stage heart failure. J Am Coll Cardiol. 1998;32:1825-1831.
Sowton E. Haemodynamic studies in patients with artificial pacemakers. Br Heart J. 1964;26:737-746.
Steendijk P, Tulner S, Bax JJ, et al. Hemodynamic effects of long-term cardiac resynchronization therapy: analysis by pressure-volume loops. Circulation. 2006;113:1295-1304.
Sweeney MO, Bank AJ, Nsah E, et al. Search AV Extension and Managed Ventricular Pacing for Promoting Atrioventricular Conduction (SAVE PACe) Trial. Minimizing ventricular pacing to reduce atrial fibrillation in sinus-node disease. N Engl J Med. 2007;357:1000-1008.
Tops LF, Schalij MJ, Bax JJ. The effects of right ventricular apical pacing on ventricular function and dyssynchrony implications for therapy. J Am Coll Cardiol. 2009;54:764-776.
Van Deursen C, van Geldorp IE, Rademakers LM, et al. Left ventricular endocardial pacing improves resynchronization therapy in canine left bundle-branch hearts. Circ Arrhythm Electrophysiol. 2009;2:580-587.
Victor F, Mabo P, Mansour H, et al. A randomized comparison of permanent septal versus apical right ventricular pacing: Short-term results. J Cardiovasc Electrophysiol. 2006;17:238-242.
Wilkoff BL, Cook JR, Epstein AE, et al. Dual Chamber and VVI Implantable Defibrillator Trial Investigators. Dual-chamber pacing or ventricular backup pacing in patients with an implantable defibrillator: The Dual Chamber and VVI Implantable Defibrillator (DAVID) Trial. JAMA. 2002;288:3115-3123.
Wilkoff BL, Corey J, Blackburn G. A mathematical model of the cardiac chronotropic response to exercise. J Electrophysiol. 1989;3:176-180.
1 Guyton AC, Hall JE. Textbook of medical physiology, ed 11. St. Louis: Elsevier; 2005.
2 Bruce RA, McDonough JR. Cardiac output at rest and during exercise. Cardiovasc Clin. 1975;6(3):353-369.
3 Wilkoff BL, Corey J, Blackburn G. A mathematical model of the cardiac chronotropic response to exercise. J Electrophysiol. 1989;3:176-180.
4 Vardas PE, Fitzpatrick A, Ingram A, et al. Natural history of sinus node chronotropy in paced patients. Pacing Clin Electrophysiol. 1991;14:155-160.
5 Barold S, Mugica J. Recent advances in cardiac pacing: goals for the 21st century. Mt. Kisco, NY: Futura publishing; 1998.
6 Sowton E. Haemodynamic studies in patients with artificial pacemakers. Brit Heart J. 1964;26:737-746.
7 Kindermann M, Schwaab B, Finkler N, et al. Defining the optimum upper heart rate limit during exercise: A study in pacemaker patients with heart failure. Eur Heart J. 2002;23:1301-1308.
8 Reiter MJ, Hindman MC. Hemodynamic effects of acute atrioventricular sequential pacing in patients with left ventricular dysfunction. Am J Cardiol. 1982;49:687-692.
9 Josephson ME, Kastor JA, Morganroth J. Electrocardiographic left atrial enlargement: Electrophysiologic, echocardiographic and hemodynamic correlates. Am J Cardiol. 1977;39:967-971.
10 Daubert JC, Pavin D, Jauvert G, Mabo P. Intra- and interatrial conduction delay: Implications for cardiac pacing. Pacing Clin Electrophysiol. 2004;27:507-525.
11 Pires LA, Abraham WT, Young JB, et aland the MIRACLE and MIRACLE-ICD Investigators. Clinical predictors and timing of New York Heart Association class improvement with cardiac resynchronization therapy in patients with advanced chronic heart failure: Results from the Multicenter InSync Randomized Clinical Evaluation (MIRACLE) and Multicenter InSync ICD Randomized Clinical Evaluation (MIRACLE-ICD) trials. Am Heart J. 2006;151:837-843.
12 Van Gelder BM, Bracke FA, Meijer A, Pijls NH. The hemodynamic effect of intrinsic conduction during left ventricular pacing as compared to biventricular pacing. J Am Coll Cardiol. 2005;46:2305-2310.
13 Kurzidim K, Reinke H, Sperzel J, et al. Invasive optimization of cardiac resynchronization therapy: Role of sequential ventricular and left ventricular pacing. Pacing Clin Electrophysiol. 2005;28:754-761.
14 Ellenbogen KA, Wilkoff B, Kay GN, Pak Lau C. Clinical cardiac pacing, defibrillation and resynchronisation therapy, ed 3. St. Louis: Saunders; 2006.
15 Daubert C, Ritter P, Mabo P, Ollitrault J, et al. Physiological relationship between A-V interval and heart rate in healthy subjects: Applications to dual-chamber pacing. PACE. 1986;9:1032-1039.
16 Ritter P, Vai F, Bonnet JL, et al. Rate-adaptive atrioventricular delay improves cardiopulmonary performance in patients implanted with a dual chamber pacemaker for complete heart block. Eur J Card Pacing Electrophysiol. 1991;1:1051-1062.
17 Sulke AN, Chambers JB, Sowton E. The effect of atrio-ventricular delay programming in patients with DDDR pacemakers. Eur Heart J. 1992;13:464-472.
18 Scharf C, Li P, Muntwyler J, et al. Rate-dependent AV delay optimization in cardiac resynchronization therapy. Pacing Clin Electrophysiol. 2005;28:279-284.
19 Ritter PH, Dib JC, Mahaux V. New method for determining the optimal atrio-ventricular delay in patient paced in DDD mode for complete atrioventricular block. Pacing Clin Electrophysiol. 1995;18(Part II):237.
20 Barold B, Ilercil A, Herweg B. Echocardiographic optimization of the atrioventricular and interventricular intervals during cardiac resynchronization. Europace. 2008;10:iii88-iii95.
21 Meluzín J, Novák M, Müllerová J, et al. A fast and simple echocardiographic method of determination of the optimal atrioventricular delay in patients after biventricular stimulation. Pacing Clin Electrophysiol. 2004;27:58-64.
22 Jansen AH, Bracke FA, van Dantzig JM, et al. Correlation of echo-Doppler optimization of atrioventricular delay in cardiac resynchronization therapy with invasive hemodynamics in patients with heart failure secondary to ischemic or idiopathic dilated cardiomyopathy. Am J Cardiol. 2006:552-557.
23 Sawhney NS, Waggoner AD, Garhwal S, et al. Randomized prospective trial of atrioventricular delay programming for cardiac resynchronization therapy. Heart Rhythm. 2004;1:562-567.
24 Bertini M, Delgado V, Bax JJ, Van de Veire NR. Why, how and when do we need to optimize the setting of cardiac resynchronization therapy? Europace. 2009;11:v46-v57.
25 Burri H, Sunthorn H, Shah D, Lerch R. Optimization of device programming for cardiac resynchronization therapy. Pacing Clin Electrophysiol. 2006;29:1416-1425.
26 Little WC, Reeves RC, Arciniegas J, et al. Mechanism of abnormal interventricular septal motion during delayed left ventricular activation. Circulation. 1982;65:1486-1491.
27 Tops LF, Schalij MJ, Bax JJ. The effects of right ventricular apical pacing on ventricular function and dyssynchrony implications for therapy. J Am Coll Cardiol. 2009;54:764-776.
28 Nahlawi M, Waligora M, Spies SM, et al. Left ventricular function during and after right ventricular pacing. J Am Coll Cardiol. 2004;44:1883-1888.
29 Delgado V, Tops LF, Trines SA, et al. Acute effects of right ventricular apical pacing on left ventricular synchrony and mechanics. Circ Arrhythmia Electrophysiol. 2009;2:135-145.
30 Chen L, Hodge D, Jahangir A, et al. Preserved left ventricular ejection fraction following atrioventricular junction ablation and pacing for atrial fibrillation. J Cardiovasc Electrophysiol. 2008;19:19-27.
31 Zhang XH, Chen H, Siu CW, et al. New-onset heart failure after permanent right ventricular apical pacing in patients with acquired high-grade atrioventricular block and normal left ventricular function. J Cardiovasc Electrophysiol. 2008;19:136-141.
32 Varma N. Left ventricular conduction delays induced by right ventricular apical pacing: effect of left ventricular dysfunction and bundle branch block. J Cardiovasc Electrophysiol. 2008;19:114-122.
33 Pap R, Furge P, Bencsik G, et al. Native QRS complex duration predicts paced QRS width in patients with normal left ventricular function and right ventricular pacing for atrioventricular block. J Electrocardiol. 2007;40:360-364.
34 Shukla HH, Hellkamp AS, James EA, et alMode Selection Trial (MOST) Investigators. Heart failure hospitalization is more common in pacemaker patients with sinus node dysfunction and a prolonged paced QRS duration. Heart Rhythm. 2005;2:245-251.
35 Wilkoff BL, Cook JR, Epstein AE, et alDual Chamber and VVI Implantable Defibrillator Trial Investigators. Dual-chamber pacing or ventricular backup pacing in patients with an implantable defibrillator: The Dual Chamber and VVI Implantable Defibrillator (DAVID) Trial. JAMA. 2002;288:3115-3123.
36 Lamas GA, Lee KL, Sweeney MO, et al. Mode Selection Trial in Sinus-Node Dysfunction. Ventricular pacing or dual chamber pacing for sinus node dysfunction. N Engl J Med. 2002;346:1854-1862.
37 Sweeney MO, Hellkamp AS, Ellenbogen KA, et alMode Selection Trial Investigators. Adverse effect of ventricular pacing on heart failure and atrial fibrillation among patients with normal baseline QRS duration in a clinical trial of pacemaker therapy for sinus node dysfunction. Circulation. 2003;107:2932-2937.
38 Kappenberger L, Linde C, Daubert C, et al. Pacing in hypertrophic obstructive cardiomyopathy. A randomized crossover study. PIC Study Group. Eur Heart J. 1997;18:1249-1256.
39 Maron BJ, Nishimura RA, McKenna WJ, et al. Assessment of permanent dual-chamber pacing as a treatment for drug-refractory symptomatic patients with obstructive hypertrophic cardiomyopathy. A randomized, double-blind, crossover study (M-PATHY). Circulation. 1999;99:2927-2933.
40 Vardas PE, Auricchio A, Blanc JJ, et al. European Society of Cardiology; European Heart Rhythm Association: Guidelines for cardiac pacing and cardiac resynchronization therapy: The Task Force for Cardiac Pacing and Cardiac Resynchronization Therapy of the European Society of Cardiology. Developed in collaboration with the European Heart Rhythm Association. Eur Heart J. 2007;28:2256-2295.
41 Olshansky B, Day JD, Moor S, et al. Is dual-chamber programming inferior to single-chamber programming in an implantable cardioverter-defibrillator? Results of the INTRINSIC RV (Inhibition of Unnecessary RV Pacing With AVSH in ICDs) study. Circulation. 2007;115:9-16.
42 Sweeney MO, Bank AJ, Nsah E, et alSearch AV Extension and Managed Ventricular Pacing for Promoting Atrioventricular Conduction (SAVE PACe) Trial. Minimizing ventricular pacing to reduce atrial fibrillation in sinus-node disease. N Engl J Med. 2007;357:1000-1008.
43 Victor F, Leclercq C, Mabo P, et al. Optimal right ventricular pacing site in chronically implanted patients: A prospective randomized crossover comparison of apical and outflow tract pacing. J Am Coll Cardiol. 1999;33:311-316.
44 Medi C, Mond HG. Right ventricular outflow tract septal pacing: long-term follow-up of ventricular lead performance. Pacing Clin Electrophysiol. 2009;32:172-176.
45 Giudici MC, Karpawich PP. Alternative site pacing: it’s time to define terms. Pacing Clin Electrophysiol. 1999;22:551-553.
46 Stambler BS, Ellenbogen K, Zhang X, et alROVA Investigators. Right ventricular outflow versus apical pacing in pacemaker patients with congestive heart failure and atrial fibrillation. J Cardiovasc Electrophysiol. 2003;14:1180-1186.
47 Victor F, Mabo P, Mansour H, et al. A randomized comparison of permanent septal versus apical right ventricular pacing: Short-term results. J Cardiovasc Electrophysiol. 2006;17:238-242.
48 Tse HF, Wong KK, Siu CW, et al. Upgrading pacemaker patients with right ventricular apical pacing to right ventricular septal pacing improves left ventricular performance and functional capacity. J Cardiovasc Electrophysiol. 2009;20:901-905.
49 Schwaab B, Fröhlig G, Alexander C, et al. Influence of right ventricular stimulation site on left ventricular function in atrial synchronous ventricular pacing. J Am Coll Cardiol. 1999;33:317-323.
50 Mond HG, Hillock RJ, Stevenson IH, McGavigan AD. The right ventricular outflow tract: The road to septal pacing. Pacing Clin Electrophysiol. 2007;30:482-491.
51 Lieberman R, Grenz D, Mond HG, Gammage MD. Selective site pacing: Defining and reaching the selected site. Pacing Clin Electrophysiol. 2004;27:883-886.
52 Kaye G, Stambler BS, Yee R. Search for the optimal right ventricular pacing site: Design and implementation of three randomized multicenter clinical trials. Pacing Clin Electrophysiol. 2009;32:426-433.
53 Shimano M, Tsuji Y, Yoshida Y, et al. Acute and chronic effects of cardiac resynchronization in patients developing heart failure with long-term pacemaker therapy for acquired complete atrioventricular block. Europace. 2007;9:869-874.
54 Leclercq C, Cazeau S, Lellouche D, et al. Upgrading from single chamber right ventricular to biventricular pacing in permanently paced patients with worsening heart failure: The RD-CHF study. Pacing Clin Electrophysiol. 2007;30:S23-S30.
55 Curtis AB, Adamson PB, Chung E, et al. Biventricular versus right ventricular pacing in patients with AV block (BLOCK HF): Clinical study design and rationale. J Cardiovasc Electrophysiol. 2007;18:965-971.
56 Lecoq G, Leclercq C, Leray E, et al. Clinical and electrocardiographic predictors of a positive response to cardiac resynchronization therapy in advanced heart failure. Eur Heart J. 2005;26:1094-1100.
57 Reuter S, Garrigue S, Barold SS, et al. Comparison of characteristics in responders versus nonresponders with biventricular pacing for drug-resistant congestive heart failure. Am J Cardiol. 2002;89:346-350.
58 Van Deursen C, van Geldorp IE, Rademakers LM, et al. Left ventricular endocardial pacing improves resynchronization therapy in canine left bundle-branch hearts. Circ Arrhythm Electrophysiol. 2009;2:580-587.
59 Derval N, Steendijk P, Gula LJ, et al. Optimizing hemodynamics in heart failure patients by systematic screening of left ventricular pacing sites: The lateral left ventricular wall and the coronary sinus are rarely the best sites. J Am Coll Cardiol. 2010;55:566-575.
60 Jaïs P, Douard H, Shah DC, et al. Endocardial biventricular pacing. Pacing Clin Electrophysiol. 1998;21(11 Pt 1):2128-2131.
61 Rao RK, Kumar UN, Schafer J, et al. Reduced ventricular volumes and improved systolic function with cardiac resynchronization therapy: A randomized trial comparing simultaneous biventricular pacing, sequential biventricular pacing, and left ventricular pacing. Circulation. 2007;115:2136-2144.
62 Pappone C, Rosanio S, Oreto G, et al. Cardiac pacing in heart failure patients with left bundle branch block: Impact of pacing site for optimizing left ventricular resynchronization. Ital Heart J. 2000;1:464-469.
63 Padeletti L, Colella A, Michelucci A, et al. Dual-site left ventricular cardiac resynchronization therapy. Am J Cardiol. 2008;102:1687-1692.
64 Leclercq C, Gadler F, Kranig W, et al. TRIP-HF (Triple Resynchronization In Paced Heart Failure Patients) Study Group: A randomized comparison of triple-site versus dual-site ventricular stimulation in patients with congestive heart failure. J Am Coll Cardiol. 2008;51:1455-1462.
65 Grines CL, Bashore TM, Boudoulas H, et al. Functional abnormalities in isolated left bundle branch block. The effect of interventricular asynchrony. Circulation. 1989;79:845-853.
66 Leclercq C, Cazeau S, Le Breton H, et al. Acute hemodynamic effects of biventricular DDD pacing in patients with end-stage heart failure. J Am Coll Cardiol. 1998;32:1825-1831.
67 Nelson G, Berger RD, Fetics BJ, et al. Left ventricular or biventricular pacing improves cardiac function at diminished energy cost in patients with dilated cardiomyopathy and left bundle-branch block. Circulation. 2000;102:3053-3059.
68 Cazeau S, Gras D, Lazarus A, et al. Multisite stimulation for correction of cardiac asynchrony. Heart. 2000;84:579-581.
69 Bleasdale RA, Turner MS, Mumford CE, et al. Left ventricular pacing minimizes diastolic ventricular interaction, allowing improved preload-dependent systolic performance. Circulation. 2004;110:2395-2400.
70 Steendijk P, Tulner S, Bax JJ, et al. Hemodynamic effects of long-term cardiac resynchronization therapy: Analysis by pressure-volume loops. Circulation. 2006;113:1295-1304.
71 Yu CM, Chau E, Sanderson JE, et al. Tissue Doppler echocardiographic evidence of reverse remodeling and improved synchronicity by simultaneously delaying regional contraction after biventricular pacing therapy in heart failure. Circulation. 2002;105:438-445.
72 Mullens W, Verga T, Grimm RA, et al. Persistent hemodynamic benefits of cardiac resynchronization therapy with disease progression in advanced heart failure. J Am Coll Cardiol. 2009;53:600-607.
73 Yu CM, Bleeker GB, Fung JW, et al. Left ventricular reverse remodeling but not clinical improvement predicts long-term survival after cardiac resynchronization therapy. Circulation. 2005;112:1580-1586.
74 Abraham WT, Leon AR, Hannon C, et al. Results of the InSync III Marquis clinical trial. Heart Rhythm. 2005;2:S65.
75 Boriani G, Biffi M, Muller CP, et alResynchronization for HemodYnamic Treatment for Heart Failure Management II (Rhythm II) Investigators. A prospective randomized evaluation of VV delay optimization in CRT-D recipients: Echocardiographic observations from the RHYTHM II ICD study. Pacing Clin Electrophysiol. 2009;32:S120-S125.