CHAPTER 48 Gastric Neuromuscular Function and Neuromuscular Disorders
Gastric neuromuscular function refers to the motility or motor activities of the stomach.
ELECTROPHYSIOLOGIC BASIS OF GASTRIC NEUROMUSCULAR FUNCTION
EXTRACELLULAR SLOW WAVES AND PLATEAU AND ACTION POTENTIALS
The stomach is a sophisticated complex sphere of smooth muscle organized into circular, longitudinal, and oblique muscle layers. Gastric myoelectrical activity, termed pacesetter potentials or slow waves, regulate, control, and pace gastric smooth muscle contractions1,2 (the term slow waves is used in this chapter). In the normal human stomach the slow waves occur at approximately 3 cycles per minute (cpm) or between 2.5 and 3.7 cpm.3,4 From the pacemaker region on the greater curvature of the stomach, between the fundus and the proximal corpus, slow waves propagate circumferentially and migrate distally toward the pylorus every 20 seconds at a velocity of approximately 14 mm/second in the distal antrum (Fig. 48-1).5,6 The gastric slow waves originate from the interstitial cells of Cajal.7,8
The depolarization upstroke of the slow wave reduces the threshold for circular smooth muscle contraction; and, in the appropriate situation, the amplitude of the circular smooth muscle contraction increases with the onset of the plateau potentials and action potentials.9,10 The aborad propagation of slow waves linked to plateau potentials (with or without action potentials) is the electrophysiologic basis of gastric peristaltic waves (Fig. 48-2). Thus, the slow waves linked with plateau or action potentials propagate through the corpus and antrum and create moving “ring contractions” that resolve in the antrum or at the pylorus in a terminal antral contraction. The pylorus provides an electrical barrier between the 3 cpm slow wave of the distal antrum and the 12 to 13 cpm slow wave of the duodenum.
INTRACELLULAR ELECTRICAL RECORDINGS FROM GASTRIC SMOOTH MUSCLE CELLS
Intracellular recordings from smooth muscle cells from the different regions of the stomach (fundus to the mid-corpus to the terminal antrum) illustrate the electrophysiologic characteristics that distinguish these regions (Fig. 48-3).1 Key features are (1) regional differences in resting membrane potential, which range from −48 to −75 millivolts (mV); (2) regional differences in threshold for contraction that vary from −52 to −40 mV; and (3) the occurrence of plateau potentials with or without spike potentials.1 The fundic smooth muscle cells are unique because their resting membrane potential lies at or above the threshold for contraction (−50 mV), a situation that promotes sustained smooth muscle contraction and ongoing fundic tone. Inhibitory vagal input to the fundus increases during swallowing and results in decreasing muscle tone associated with “receptive relaxation” and the accommodation of swallowed foodstuffs.11,12 Fundic muscle tone decreases in proportion to the intensity and duration of the inhibitory neural discharge.
In contrast to the fundus, intracellular recordings from the corpus indicate a lower resting membrane potential of −60 mV. The rapid upstroke depolarization in these cells is followed by a plateau potential that slowly returns to the baseline resting electrical potential (see Fig. 48-3F). The plateau potentials are associated with circular muscle contraction activity in the corpus and antrum.1 The plateau potential may be accompanied by action potentials in the corpus and antrum (see Fig. 48-3G and H). Extrinsic stimuli such as release of acetylcholine or stretch of the stomach wall increases the amplitude and duration of the plateau potential and the occurrence of action potentials, resulting in contractions of varying force, as seen in the muscle of the terminal antrum. Depending on the excitatory neural stimuli and the amplitude of plateau potentials and the number of action potentials, peristaltic contraction waves of the circular muscle layer vary from very-low-amplitude contractions to high-amplitude lumen-occluding contractions. At the pylorus, the plateau potentials have long durations and superimposed action potentials that result in closure of the pyloric sphincter in conjunction with the terminal antral contraction (see Fig. 48-3H and I).1
The membrane potential and the force of smooth muscle contraction also distinguish the fundus, corpus, and antrum (Fig. 48-4). The resting membrane potential of the fundus is approximately −50 mV and produces the sustained contraction and the resting tone of the fundus.1 This fundic tone ensures a sensitive response to excitatory or inhibitory stimuli for relaxation or contraction of the fundus. Receptive relaxation during ingestion of food is accomplished by these electrophysiologic attributes of smooth muscle in the fundus. In contrast, the resting membrane potentials of the corpus and antrum are −60 to −70 mV, respectively. In the presence of plateau potentials or action potentials the membrane potential reaches −45 mV or less and smooth muscle contraction occurs. If the plateau potentials have higher amplitude, then contractions of larger amplitude or force occur. When the plateau potential and action potentials are linked to the propagating slow waves in the antrum, then the moving ring contractions of the gastric peristaltic “waves” are formed (see Fig. 48-2).
INTERSTITIAL CELLS OF CAJAL
ICCs are the “pacemaker cells” for the smooth muscle apparatus of the gastrointestinal tract.7,8,13,14 ICCs originate from c-Kit–positive mesenchymal cell precursors.15 ICCs in the stomach are located in submuscular, intramuscular, myenteric, and subserosal layers of the gastric wall.16,17 Figure 48-5 shows the anatomic relationships between the ICCs in the myenteric plexus (MY-ICCs), the intramuscular ICCs (IM-ICCs), the enteric neurons, and the circular smooth muscle cells. MY-ICCs are located between the circular and longitudinal muscle layers of the stomach and are the ICCs responsible for the generation of the slow waves (see Fig. 48-5). These ICCs spontaneously generate slow waves that are conducted into adjacent smooth muscle cells and cause depolarization and contraction of the smooth muscle by activating voltage-dependent, dihydropyridine-sensitive (l-type) calcium channels (see Fig. 48-5).18–20 Increased amplitude of the plateau potential correlates with increased amplitude of smooth muscle contraction (Fig. 48-6). The slow waves propagate circumferentially and distally through the ICC network via gap junctions and entrain more distal ICCs with slower intrinsic frequencies to the higher slow wave frequency, the pacemaker frequency.
ICCs are also located within the layers of the circular smooth muscle (IM-ICCs), where they integrate and coordinate the spread of the slow wave and the smooth muscle contraction initiated by the MY-ICCs.10 Slow waves are not regenerated in the smooth muscle cells because the ion channels needed to generate and propagate slow waves are not expressed by gastric smooth muscle.
The ICCs have innate rhythmicity that is based on their unique metabolism and fluxes in intracellular and extracellular calcium.19,21 The most active area of depolarization and repolarization of the ICCs is in the pacemaker area of the stomach located between the fundus and the proximal corpus (see Fig. 48-1). The depolarization and repolarization of the ICCs is regenerated and propagated through the network of ICCs in a migrating wave front that moves from the pacemaker region on the greater curve through the corpus and antrum to the pylorus, as shown in Figures 48-1 and 48-2, proscribing the pathway of gastric peristaltic contractions. Excitatory inputs (e.g., cholinergic stimuli, stretch) to the MY-ICC results in opening of calcium channels and depolarization of the smooth muscle cells with IM-ICC activation to coordinate the contractions of the circular muscle cells in time and space. Thus, the ICC networks provide the control of frequency and propagation velocity for the circular muscle contractions that comprise gastric peristalsis waves.
The fundus of the stomach lacks slow waves (see Figs. 48-1 and 48-3A). The IM-ICCs in the fundus have a role in mechanoreception and act as sensory cells with interconnections to the vagal afferent neurons that innervate the fundus.22,23 Fundic IM-ICCs are also innervated by inhibitory vagal neurons that regulate tone in the fundus.23 Thus, the ICCs also participate in the relaxation of fundic tone that occurs during accommodation.24
Loss of ICCs in the antrum is associated with gastroparesis in patients with diabetes mellitus.25 Patients with diabetic gastroparesis and severe loss of ICCs have more gastric electrical dysrhythmias (tachygastria), more upper gastrointestinal symptoms, and poorer response to gastric electrical stimulation compared with patients with normal numbers of ICCs.26 Electrical dysrhythmias and gastroparesis were recorded in rodents with experimental diabetes.27 Interruption of ICC pathways from nondiabetic mechanisms also results in gastric dysrhythmias and ectopic pacemakers that are similar to gastric dysrhythmias found in patients with diabetes.28,29
NERVOUS SYSTEM INNERVATION
As reviewed earlier in Chapter 47, neurons of the ENS populate the stomach wall from the fundus to the pylorus.30 These neurons are located in the myenteric plexuses between the circular muscle and the longitudinal muscle layers (see Fig. 48-5). Neurons of the ENS are also located in submucosal and subserosal plexuses. The ENS provides local reflex circuits within the gastric wall: (a) sensory afferent neurons located in the mucosa linked to (b) interneurons in the myenteric plexus that are linked to (c) efferent neurons that innervate the smooth muscle and glands to perform the gastric secretomuscular functions.31,32 Release of excitatory neurotransmitters such as acetylcholine and substance P stimulates smooth muscle contractions, whereas inhibitory neurotransmitters such as nitric oxide and vasoactive intestinal polypeptide inhibit contractions. These enteric neural circuits within the gastric wall are programmed to modulate peristaltic contractions (in conjunction with ICC activity described earlier) by sequential inhibition of the distal smooth muscle segment and contraction at the immediate proximal segment of the stomach wall.33,34 Serotonin in the bowel wall has a primary role in initiating and controlling peristaltic events.32
Neurons of the ENS are located in proximity to the MY-ICCs and IM-ICCs (see Fig. 48-5).35 The ENS neurons provide additional control and modulation of contraction and relaxation of the gastric smooth muscle via cholinergic excitation and nitrergic inhibitory neurotransmission. Neurons of the ENS form gap junctions with MY-ICCs and IM-ICCs and provide an additional layer of neural control that integrates slow wave activity and smooth muscle activity. Thus, postganglionic excitatory and inhibitory neurons innervate MY-ICCs to modulate gastric neuromuscular contraction and relaxation and provide chronotropic effects on the slow waves.
The PNS and SNS modulate gastric neuromuscular activity. The vagus nerve provides the PNS input for the stomach, although approximately 80% of vagal fibers are afferent neurons. The afferent neurons are responsive to moment-to-moment contraction and relaxation (tone) of the stomach wall.36 Efferent activity of the vagus nerve increases the release of acetylcholine, which increases the amplitude of gastric contractions and stimulates secretion of gastric acid and pepsin. The SNS innervates gastric smooth muscle with neurons that travel with the splanchnic vasculature. SNS activity generally elicits inhibitory action on the smooth muscle via effects on the myenteric neurons of the ENS.37
GASTRIC NEUROMUSCULAR ACTIVITY DURING FASTING
In the fasting state, electrical and contractile events of the corpus or antrum occur in a highly regular pattern termed the migrating myoelectrical (or “motor”) complex, or MMC.38,39 The three phases of the MMC, as described by changes in intraluminal contractions, recur approximately every 90 to 120 minutes. Phase 1 is a period of quiescence wherein little or no contractile activity is recorded. Phase 1 is followed by phase 2 during which random, irregular contractions occur. Phase 3 of the MMC is a burst of regular, high-amplitude phasic contractions that last from 5 to 10 minutes (Fig. 48-7A). Phase 3 contractions are also termed the “activity front.” The activity front migrates from the antrum to the ileum, a journey of 90 to 120 minutes’ duration. The three phases of the MMC occur regularly in the small intestine, whereas approximately 50% of the phase 3 activity fronts originate in the stomach and then migrate through the small intestine.40 The MMCs that originate in the stomach or duodenum travel through the small intestine and terminate in the distal ileum. If fasting continues, then another phase 3 activity front reappears in the antrum or duodenum at the 90- to 120-minute interval. The high-amplitude, three-per-minute contractions of phase 3 that develop in the distal antrum empty nondigestible, fibrous foodstuffs that remain in the stomach after a meal.
Cyclic contractile activity associated with the onset of phase 3 also has been identified in the lower esophageal sphincter, the sphincter of Oddi, and the gallbladder. The phase 3 contractions correlate with rapid eye movement (REM) sleep and are related to a larger system of biological clocks.38,41 MMCs develop after vagotomy, indicating that nonvagal mechanisms initiate and sustain MMC neuromuscular activity. Motilin is released during the intense phase 3 contractions that occur in the proximal duodenum.42 Motilin appears to be important for phase 3 activity of the MMC because administration of a motilin-neutralizing antibody abolishes the phase 3 contractions.
GASTRIC NEUROMUSCULAR ACTIVITY AFTER A MEAL
Gastric Neuromuscular Response to the Ingestion of Solid Foods
The neuromuscular work of the stomach in mixing, milling, and emptying food depends upon the physical characteristics, volume, and the fat, protein, and carbohydrate content of the ingested food. For example, almost 240 minutes of neuromuscular work is required to empty approximately 95% of a 255-kcal low-fat, egg substitute sandwich.43 In contrast, 35 minutes of gastric neuromuscular work is required to empty almost 70% of a 20-kcal 500-mL soup broth meal that was consumed in four minutes.44 Each meal requires its own specific time to be emptied, an emptying time achieved by the distinct gastric neuromuscular “work” elicited by the specific food. Figure 48-8 illustrates gastric neuromuscular activity required to receive, mix, and empty a solid meal. The spectrum of gastric work extends from fundic relaxation to gastric peristalsis to antropyloroduodenal coordination, the work that is needed to produce chyme and empty it into the duodenum. Ingestion of food abolishes the fasted state as regular three per minute gastric peristalsis begins in the corpus and antrum to mix the food; and in the fed state, a pattern of continuous small bowel contractions with short runs of peristalsis over distances of 2 to 4 cm optimize digestion and absorption of nutrients (see Fig. 48-7B).
Solid food delivered from the esophagus into the fundus is associated with receptive relaxation of the fundus, the “work” of fundic muscle relaxation. As the fundic smooth muscle relaxes, larger amounts of solid or liquid food are accommodated in the fundus and proximal corpus with little or no increase in intraluminal pressure. Liquids, in contrast, are immediately distributed throughout the antrum and corpus (emptying of liquids is discussed in the next section). Relaxation of the fundus occurs before the work of trituration in the corpus-antrum and is a vagal nerve–mediated event that requires nitric oxide.45,46 Figure 48-9 shows an example of the changes in intragastric volume during relaxation of the fundus and proximal corpus in response to a caloric meal.47 Relaxation of the fundus and the stimulation of mechanoreceptors (stretch), mediated through IM-ICCs in the fundic wall, activate vagal afferent neurons and vagovagal reflexes. These reflexes involve the nucleus of the tractus solitarius and efferent neurons from the dorsal motor nucleus of the vagus. Vagal excitatory neurons are inhibited and the vagal inhibitory neural transmitters nitric oxide and vasoactive intestinal peptide (VIP) are released to accomplish receptive relaxation.
Other factors influence the muscle tone of the fundus. Antral distention, duodenal distention, duodenal acidification,48 intraluminal perfusion of the duodenum with lipid or protein, and colonic distention all decrease fundic tone through various reflexes. The gastric reflex is mediated through an arc initiated by capsaicin-sensitive afferent vagal nerves and is mediated by 5-hydroxytryptamine-3 (5-HT3), gastrin-releasing peptide (GRP) and CCKA receptors.49
Solid foods labeled with technetium are accommodated initially in the fundus and proximal corpus, and by obtaining frequent scintigraphic images, the distribution of the labeled solid meal can be followed over four hours using scintigraphic methods.43 Figure 48-10 shows that immediately after ingestion of this solid meal, the food is accommodated and the majority of the meal is retained in the fundus and proximal corpus. Subsequently, contractions of the fundus press portions of the food into the corpus and antrum for trituration. This early postprandial period of accommodation and trituration, that occurs before gastric emptying of the nutrients, is termed the lag phase. The lag phase may last from 45 to 60 minutes for solid foods, but the duration of the lag depends on the components of the meal, the thoroughness of chewing the food, and the time required to ingest the meal. For the 255-kcal egg substitute test meal that is ingested in a 10-minute period, the lag phase is 30 to 45 minutes.
Once portions of the meal have been triturated into 1- to 2-mm particles suspended in gastric juice, the linear phase of gastric emptying of the chyme begins. Recurrent gastric peristaltic waves mix saliva, acid, and pepsin with the chewed food and then mill the food to produce chyme. The normal peristaltic waves occur every 20 seconds, generated by 3 cpm slow waves linked to plateau and action potentials. In healthy subjects approximately 60% of the egg substitute meal is emptied in two hours and more than 95% is emptied at four hours (Fig. 48-11).43
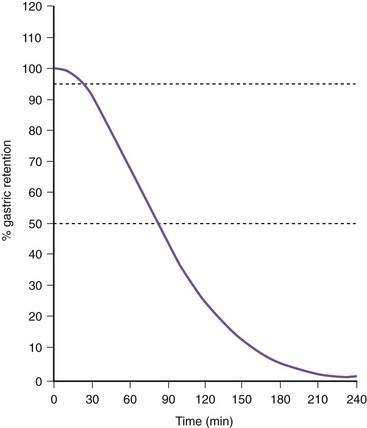
Figure 48-11. Solid phase gastric emptying curve for 123 subjects after ingestion of a 255-kcal substitute egg meal, the same meal shown in Figure 48-10. Note that only approximately 15% of the eggs are emptied in the first 45 minutes, the lag phase of gastric emptying of this meal. At 90 minutes approximately 50% of the meal has been emptied and 50% is retained. By 240 minutes more than 95% of the meal has been emptied.
(Modified from Tougas G, Eaker EY, Abell TL, et al. Assessment of gastric emptying using a low fat meal: Establishment of international control values. Am J Gastroenterol 2000; 95:1456-62.)
During the linear phase of gastric emptying, each peristaltic wave empties from 3 to 4 mL of chyme into the duodenum.50,51 Movement of chyme into the duodenum is usually, but not always, pulsatile due to the systole-like effect of antral peristaltic waves.51 The volume of chyme delivered into the duodenum by each peristaltic wave is modulated by the configuration of the peristaltic wave (e.g., depth of contraction, length of the peristaltic wave) pressure within the stomach, and resistance to flow provided by the pyloric sphincter and duodenal contractions.52,53 The gastric peristaltic wave delivers a larger stroke volume when the pylorus and the duodenum are relaxed to receive the aliquot of chyme, but the overall rate of calories delivered each minute to the duodenum is consistent at approximately 3 to 4 kcal/min.54
As time elapses after ingestion of the meal, the chewed food is continually redistributed from the fundus to the antrum for trituration (see Figs. 48-8 and 48-10). Some gastric peristaltic waves end at various points in the antrum and others end with a terminal antral contraction associated with closure of the pylorus that prevents the emptying of larger food particles or indigestible solids. These terminal antral and pyloric contractions result in retention of the solid particles in the corpus and antrum. In this manner, solid food particles that require further trituration are retained and subjected further to the milling effects of the recurrent peristaltic waves (see Figs. 48-7B and 48-8).
The intragastric pressure and gastric intraluminal pH values recorded after a healthy subject ingested an egg substitute meal are shown in Figure 48-12. During the first 2 hours after the meal the amplitude of intraluminal contractions varies from 10 to 40 mm Hg. Approximately three and a half hours after the solid meal was ingested, high amplitude contractions (>65 mm Hg) occur just before the pH increases from 1 to 6 as the motility/pH capsule is emptied from the acidic antrum into the alkaline environment of the duodenum. After the digestible components of the meal are emptied, strong antral contractions (phase 3–like contractions) empty the capsule from the stomach into the duodenum.55 Thus, fibrous and indigestible materials are emptied by high-amplitude, antral contractions, whereas the digestible nutrients in the chyme are emptied earlier by the lower-amplitude peristaltic waves during the linear phase of emptying.56
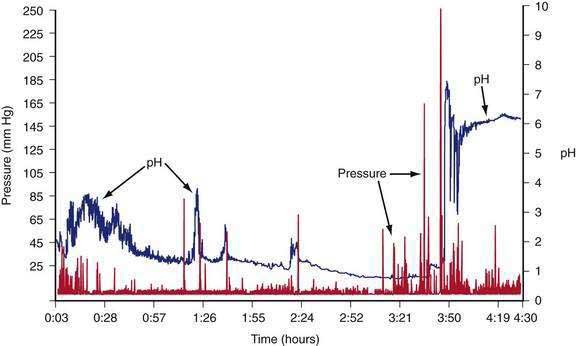
Figure 48-12. Gastric contractions and intraluminal gastric pH recordings during the emptying of a 255-kcal egg substitute meal, the same meal shown in Figure 48-10, recorded with an ambulatory capsule pH and motility device. pH is shown on the right vertical axis and pressure in mm Hg is shown on the left vertical axis. The pH increases to approximately 3 for the first 45 minutes as gastric acid is buffered by the meal. The pH then gradually decreases to 1 and remains near 1 at about 3 hours after ingestion of the meal. Stomach contractions are generally of low amplitude, less than 10 mm Hg after ingestion of the meal. At approximately 3 hours and 40 minutes after the meal, the recorded pH increases abruptly to 7 and then decreases and remains stable at around 6. Prior to the abrupt increase in pH, there is a series of clustered, high-amplitude antral contractions (pressure). These antral contractions empty the capsule from the antrum (pH 1) into the duodenum, where the pH is 6 or more. The contractions that occurred during the 3 hours and 50 minutes required to empty the meal document the neuromuscular work required to triturate and empty this meal in a healthy subject.
Gastric Neuromuscular Response to the Ingestion of Liquids
The gastric neuromuscular activity required to mix and empty liquids from the stomach is distinctly different compared with the emptying of solid foods.55–59 Figure 48-13 shows three-dimensional (3-D) ultrasound images of the stomach in a healthy subject during the fasting state and 10 minutes after the subject ingested 500 mL of soup.58 The intragastric volume was approximately 40 mL during fasting and increased almost nine-fold to 350 mL 10 minutes after ingestion of the meal, indicating the remarkable relaxation of the smooth muscle of the antrum and corpus (in addition to the fundic relaxation) that was required to accommodate this liquid volume. (In contrast, solid meals are initially accommodated and retained primarily in the fundus and proximal stomach, as shown in Figs. 48-9 and 48-10.) Once accommodated, nutrient liquids are emptied into the duodenum in a controlled but more rapid rate compared with solid foods, which require trituration. Noncaloric liquid meals empty without the lag phase in a curve described as monoexponential emptying (Fig. 48-14).60 Caloric-dense liquids on the other hand are retained for longer periods in the antrum and are emptied slower than noncaloric liquids. Liquids are emptied from the stomach by a combination of (a) pressure gradients between the stomach and the duodenum that produce flow of liquid into the duodenum; (b) antral peristaltic contractions that produce a pulsatile pattern of emptying of liquids from the antrum into the duodenum; and (c) duodenogastric reflux events that modify gastric emptying results.51,52 From a gastric myoelectrical activity viewpoint, ingestion of water until the point of fullness induces a brief “frequency dip” followed by normal 3 cpm activity on the electrogastrogram (EGG) (Fig. 48-15).61 The rate of gastric emptying of liquids is influenced by the volume, nutrient content, viscosity, and osmolarity of the ingested liquid.53,54,59,62 These factors affect the neuromuscular activity of the stomach, which ultimately produces the rate of emptying. These factors are discussed below.
REGULATION OF GASTRIC NEUROMUSCULAR ACTIVITY AFTER A MEAL
Gastric emptying rates are regulated to achieve a consistent, regular presentation of chyme to the duodenum in order to optimize secretion of pancreatic enzymes and bile appropriate for digestion of the contents of the chyme. Various gastric emptying rates are achieved by variations in the neuromuscular armamentarium of the stomach: fundic relaxation and contraction; the characteristics of gastric peristaltic contractions; temporary suspension of 3 cpm slow waves and the onset of gastric dysrhythmias; the coordination of antropyloroduodenal contractions and duodenal contractions; or resistances that promote duodenogastric reflux. The attributes of a specific meal stimulate the appropriate gastric neuromuscular responses that affect the rate of gastric emptying. Table 48-1 lists gastric neuromuscular factors, meal-related factors, and other factors that modulate the rate of gastric emptying. The rate of gastric emptying is decreased by the temporary occurrence of gastric dysrhythmias, modulation of the amplitude and the propagation distances of antral contractions, enhanced contractions of the pylorus, and reduced antropyloroduodenal coordination.
FACTORS | EFFECT ON RATE OF GASTRIC EMPTYING (SYMPTOMS) |
---|---|
Gastric Neuromuscular | |
CHO, carbohydrate; IBS, irritable bowel syndrome.
Meal-related factors that affect gastric emptying include the digestible components of the solids and liquids, fat content (nutrient density), viscosity, acid content, volume, and indigestible foodstuffs. For example, foods with high fat content empty slower than foods with high protein or carbohydrate content. Triglycerides are mixed with gastric lipase during the initial intragastric phases of digestion (Chapter 49) and are broken down to fatty acids and mono- or diglycerides before emptying into the duodenum.63 The duodenum is exquisitely sensitive to diet-derived fatty acids. Longer chain fatty acids (longer than C12) exposed to the mucosa of the duodenum result in release of CCK. CCK relaxes fundic tone, decreases antral contraction, and increases pyloric tone, all of which result in delay in gastric emptying. In contrast, short- and medium-chain fatty acids (shorter than C12) do not have these neuromuscular effects on gastric emptying rates.54,64 CCK released from the duodenum also activates CCKA receptors on the vagal afferent neurons with synapses in the nucleus tractus solitarius.65 Neurons from the nucleus tractus solitarius ascend to the periventricular nucleus that participate in mechanisms of satiation, and descending vagal efferent neurons from the dorsal motor nucleus of the vagus inhibit gastric emptying and maintain fundic relaxation. The sensitivity of the duodenal mucosa to fat and other nutrients led to the concept of duodenal tasting and duodenal brake, sensorimotor events that modulate gastric emptying of nutrients.66–68
Monosaccharides in the duodenum stimulate the release of incretins such as glucagon-like polypeptide-1 (GLP-1), which promotes insulin secretion to match increasing postprandial blood glucose levels and decreases antral contractions.69–71 In order to harmonize the relationships between glucose absorption, glycemia, and insulin secretion, the gastric emptying of carbohydrates is highly regulated.72 Hasler and colleagues showed that hyperglycemia decreases antral contractions and increases gastric dysrhythmias, a “physiologic” gastric dysrhythmia that decreases the rate of gastric emptying (Fig. 48-16).6,73 Glucagon infusions also induce bradygastrias.74 Hyperglycemia increases fundic compliance and decreases sensations related to fundic distention.75 Blood glucose levels greater than 220 mg/dL result in decreased antral contractions, decreased gastric emptying, and induced gastric dysrhythmias,6,73 all of which are gastric neuromuscular activities that reduce gastric emptying and reduce further exposure of the duodenum to nutrients. Hypoglycemia on the other hand increases gastric contractility and emptying.76
The interaction between nutrients in the lumen and the regulation of the rate of gastric emptying continues in the later postprandial period as digestion and absorption of nutrients occur throughout the small intestine. For example, if diet-derived fatty acids or carbohydrates reach the lumen of the ileum, the so-called ileal break is activated and gastric emptying is delayed. Infusion of nutrients into the lumen of the ileum delays gastric emptying,77 an enterogastric reflex mediated in part by peptide YY, CCK, and GLP-1 (see Chapter 1).61,68,77
Regulation of stomach emptying also is achieved by vagus nerve and splanchnic nerve activity that modulates the neuromuscular activities of the stomach described earlier. Vagal afferent nerves “monitor” neuromuscular function in the stomach moment by moment, and interactions between afferent vagal nerve activity and the nucleus tractus solitarius and synapses with the efferent vagal nerve output from the dorsal motor nucleus produce an ongoing interaction of CNS excitatory and inhibitory effects on the stomach. Gastric emptying is delayed during stress. Corticotropin-releasing factor (CRF) plays a role in the mediation of stress and inhibits gastric emptying through central dopamine1 and 2 and vasopressin (AVP) pathways in the periventricular nucleus.78 Other factors that affect the rate of gastric emptying not already mentioned include rectocolonic distention, nausea and vomiting of pregnancy, and vection-induced motion sickness.79 Stimulation of various areas in the CNS affects gastric neuromuscular function. Illusory self-motion (vection) induces antral hypomotility, tachygastria, and decreased gastric emptying.79,80 A series of studies using the experience of illusory self-motion, a unique CNS sensory stimulation, showed that the onset of nausea was associated with tachygastria and increased levels of plasma vasopressin.80,81
Gender affects the gastric emptying rate of a standard meal. Gastric emptying is significantly slower in healthy women compared with men.43,82,83 Gender differences in gastric emptying rates may be related to fluctuations in sex hormones, but phases of the menstrual cycle (variations in estradiol and progesterone concentrations) have not shown consistent relationships with emptying measurements.84 The rate of gastric emptying increases as body mass index rises, a relationship that may be relevant to the onset and maintenance of obesity.
GASTRIC SENSORY ACTIVITIES
Free nerve endings in the stomach act as polymodal sensory receptors that respond to light touch or pressure, acid, and other chemical stimuli. Afferent neurons within the stomach are termed intrinsic primary afferent neurons, or IPANs.85 Cell bodies of IPANs reside in the submucosal or the myenteric plexus areas of the stomach wall. IPANs may be activated by serotonin release from local enterochromaffin cells.32,86 The afferent information in the IPANs is used in local reflexes and provides input to vagal and splanchnic afferent neurons for vagovagal and spinal reflexes, respectively, to subserve transmission of visceral sensory information to CNS centers. Vagal afferent neurons whose cell bodies reside in the nodose ganglia connect with the nucleus of the tractus solitarius and second-order neurons connect with higher center of the hypothalamus, and some inputs reach the cortex, where they are consciously perceived as visceral sensations (stomach emptiness or fullness) or symptoms such as nausea or abdominal pain (Fig. 48-17).
From the SNS, splanchnic or spinal primary afferent neurons in the gastric wall mediate pain sensations. Cell bodies of these neurons lie in the dorsal horn of the spinal cord with second-order neurons that ascend via the spinothalamic and spinoreticular tracks in the dorsal columns. Sensory neurons are thin, myelinated A-delta or unmyelinated C fibers. Spinal afferents include a population of unmyelinated C fibers. Capsaicin-sensitive unmyelinated fibers contain neuropeptides such as CGRP, VIP, somatostatin, substance P, and neurokinin A. These fibers are considered to be the primary route of transmission for various pain stimuli from the gut to the CNS. These nerve fibers may respond to inflammatory mediators that also awaken “silent” nociceptive fibers.86
In addition to interacting with IPANs, vagal afferent axons have multiple connections with the enteric neurons and innervate the circular muscle fiber bundles via connections with ICCs.87 Vagal afferent neurons are also sensitive to chemostimuli via mucosal neurons and mechanosensitive neurons and ICCs in the muscle layers. CCK receptors on vagal afferent neurons are primarily activated by physiologic mechanical and chemical stimuli from the stomach during fasting and fed conditions. These vagal afferents mediate the sensory response to intraluminal acid and fat. Acid may have a direct action on the nerve endings themselves.88
Nausea is a common sensation that is often attributable to stomach dysfunction. During the illusion of self-motion, gastric dysrhythmias develop as healthy individuals report nausea.89 Plasma vasopressin levels increase in the subjects who develop nausea, but do not increase in those who experience no nausea.90 This brain-gut, gut-brain interaction during illusory self-motion illustrates the temporal relationships between the onset of gastric dysrhythmias in the periphery and acute, severe nausea experience of the subject. On the other hand, mechanical or physical distention of the antrum, but not the fundus, using a balloon induces nausea sensations and gastric dysrhythmias in healthy individuals.91 These studies show that gastric dysrhythmias originate in the antrum in humans and that stretch of the antral wall is another mechanism that elicits gastric dysrhythmias and nausea sensations from the stomach. Distention of the gastric antrum and corpus by the water-load test (rather than a balloon) also elicits the gastric dysrhythmias and nausea in susceptible individuals.61
THE STOMACH AND THE REGULATION OF FOOD INTAKE, HUNGER, AND SATIETY
The volume of food ingested suppresses hunger and stimulates the sense of fullness more than the calorie content of the meal.92–94 Infusion of nutrients into the stomach induces a greater intensity of fullness or satiety compared with infusion of the same nutrients into the duodenum.67 The suppression of hunger is greater when nutrients are taken by mouth, indicating that CNS, oropharyngeal, and gastric neuromuscular factors are integrated to produce the comforts of normal postprandial stomach fullness.95
Healthy individuals usually eat until they are reasonably full. The physiologic attributes of fullness are not completely known, but the physical stretch on the stomach walls induced by the volume of food ingested and the gastric juice secreted are responsible, in part, for the sense of postprandial fullness.93,96 Subjects experience a dramatic change from the sensation of stomach emptiness at baseline to the sensation of stomach fullness after ingesting water over a five-minute period. The average volume of water ingested to achieve fullness is 600 mL of water; in contrast, patients with functional dyspepsia ingest, on the average, 350 mL of water on average and feel full, indicating a disturbance in stomach wall relaxation and/or wall tension.61 Similarly, fullness and satiety can be achieved by ingesting a nutrient drink until achieving maximum tolerated satiety.97 The presence of acid or nutrients in the duodenum or an elevated blood glucose level decreases the stomach wall tension.98,99
The physiologic mechanisms of hunger and satiety (and stomach emptiness and fullness) are under intense investigation. In the fasting state plasma motilin levels increase during the phase 3 of the MMC, but correlations between the sensation of hunger and increases of motilin or onset of phase 3 have not been described. As discussed in other chapters, ghrelin is a 28 amino acid peptide secreted from endocrine cells of the oxyntic glands in the gastric fundus.100 Ghrelin levels increase in the plasma during fasting (hunger) and stimulate food intake, probably acting via vagal afferent nerves.101 Orexins or appetite-stimulating peptides are synthesized by neurons in the lateral hypothalamus, promote food intake, and stimulate gastric contractility (in the rat) by actions on the dorsal motor nucleus of the vagus with projections to the gastric fundus and corpus.102 After ingestion of food, ghrelin levels decrease103 and are profoundly suppressed after gastric bypass surgery.104 Ghrelin also has promotility effects on the stomach and is being evaluated for the treatment of gastroparesis.105,106
Other hormones are candidates for important roles in the sensation of fullness or satiety, and these hormones are released after the ingestion of meals. CCK is released from the duodenal mucosa exposed to fatty acids as described previously. CCK receptors participate in fullness and nausea sensations elicited by intraduodenal lipid and gastric distention.107,108 Leptin is synthesized in the stomach and released after food ingestion; circulating leptin reduces food intake via CNS regulation of the arcuate nucleus.71,109 GLP-1 enhances fullness after a standard meal, reduces antral motility, and increases gastric volume.71,110 Apolipoprotein A-IV is released from the small intestine during absorption of triglycerides and decreases food intake and gastric motility, in part, via CCK and vagal afferent pathways.71,111 Polypeptide-YY (PYY) is released from the ileocolonic area after meals and is an important mediator of the “ileal brake” effect112 and appetite suppression.113,114
The brain and these gut hormones are clearly linked in the regulation of food intake and the regulation of gastric neuromuscular activity that produces stomach emptying.71,114 The cephalic phase of gastric physiology is well known but has not been re-explored for many years. The sight, smell, and taste of food stimulate central vagal efferent activity that increases gastric acid secretion, gastric contractility, and increases 3 cpm gastric myoelectrical activity.115–117 Sham feeding, during which the subject chews and spits out the test meal rather than swallowing it, elicits the cephalic-vagal reflex. Sham feeding a warm hot dog on a bun elicits enhanced 3 cpm EGG activity, whereas sham feeding a cold tofu dog, a food that the subjects considered disgusting, resulted in blunted or no increase in the 3 cpm myoelectrical activity (Fig. 48-18).117 Thus, sensory and emotional attributes of food during the cephalic phase of ingestive behavior also affect the neuromuscular activity of the stomach.
DEVELOPMENTAL ASPECTS OF GASTRIC NEUROMUSCULAR FUNCTION
Gastric peristalsis appears between 14 and 23 weeks of gestation. Grouped or clustered peristaltic waves are evident by 24 weeks.118 The neuroregulatory mechanisms responsible for the coordination of antropyloroduodenal motility in gastric emptying are well developed by 30 weeks of gestation.119 EGG recordings show normal 3 cpm activity in preterm infants delivered at 35 weeks that are similar to EGG signals recorded in full-term infants.120,121 On the other hand EGG recordings from premature infants (less than 35 weeks’ gestation) showed considerable tachygastria.120 Gastric myoelectrical activity matures further over the first 6 to 24 months of life and achieves full adult values by the end of the first decade.121,122
The development of ICCs has been studied intensely because of the interest in gastric electrical rhythmicity, smooth muscle contractions, and gastric dysrhythmias. Labels for the tyrosine kinase receptor (c-Kit) and the availability of knock-out mice lacking c-Kit have led to increased understanding of the development of ICCs.123 The ICCs demonstrate differential development, with c-Kit expression on ICCs in the MY-ICCs developing before birth, whereas ICCs in the deep muscular plexus (IM-ICCs) develop after birth.124 ENS and ICC networks are not fully developed and are poorly coupled at birth, but progressive maturity of gastric rhythmicity and contractility occur during perinatal development.120,121 The ENS and ICCs in the deep muscular plexus are closely related, whereas ICCs in the myenteric plexus can develop normally in the absence of the enteric nervous system.124 Loss of ICCs in the pylorus is associated with loss of the inhibitory neural activity that may contribute to the development of pyloric stenosis in infants (see Chapter 47).125
ASSESSMENT OF GASTRIC NEUROMUSCULAR FUNCTION
GASTRIC EMPTYING RATES
Scintigraphy
Test meals labeled with radioisotope are available to assess the rate of gastric emptying. The seminal solid-phase gastric emptying protocol was a multinational study that used a 255-kcal technetium-99m (99mTc)–labeled egg substitute with bread and jam as the standard meal.43 Scans were obtained for 1 minute immediately after ingestion of the meal and at 30 minutes, 60 minutes, 120 minutes, 180 minutes, and 240 minutes in 123 healthy individuals. Delayed gastric emptying was defined as greater than 60% retention of the meal at 120 minutes and greater than 10% retention of the meal in the stomach 240 minutes after ingestion (see Figs. 48-10 and 48-11). The four-hour emptying test was superior to the two-hour test because almost 20% of patients with suspected gastroparesis had normal emptying at two hours, but abnormal emptying at four hours.126
Pitfalls in the scintigraphic method for solid-phase gastric emptying studies include improper binding of the isotope with the test meal, which results in rapid or normal emptying and continuance of medications that may stimulate (e.g., metoclopramide) or inhibit (e.g., narcotics, anticholinergic agents) gastric smooth muscle contractions. These medications should be stopped five to seven days before all gastric neuromuscular tests, if possible. Radiation exposure for the subject occurs with the scintigraphic tests, and multiple tests in the same subject are not advisable. Liquid-phase gastric emptying tests can be performed with indium 111-diethylenetriaminepentaacetic acid (111In-DTPA) 99mTc-labeled water or other liquids. Patients with unexplained nausea symptoms may have altered emptying of liquid meals, even if solid phase emptying is normal.127,128
Capsule Technology
Gastric emptying time of test meals is obtained from a small capsule that measures intraluminal pH and contractions (see Fig. 48-12). The capsule is swallowed with a standard test meal. During the postprandial period, measurements of luminal pH and contractions are transmitted to a receiver worn by the subject. In healthy subjects the capsule is emptied from the stomach into the duodenum approximately five hours after ingestion of the egg substitute meal. Emptying of the capsule correlated with 90% emptying of the technetium-labeled egg substitute solid meal. The test had very good sensitivity and specificity in detecting gastroparesis.83
Breath Tests
Breath tests indirectly reflect gastric emptying of solid and liquid test meals. The solid meals are labeled with C13 and include C13 octanoic acid, C13 acetate, or C13 Spirulina platensis. The C13 octanoic acid breath test has been performed in many experimental protocols and is used widely in Europe for research and clinical studies.129,130 The C13-labeled food is emptied from the stomach and absorbed in the small intestine. The labeled nutrients are metabolized in the liver to C13O2, excreted in the lungs, and detected in breath samples. Breath samples are collected at 45, 90, 120, 150, and 180 minutes after the meal in the C13 Spirulina test. C13 is a stable isotope with no radiation risks. The C13 breath tests are generally comparable to scintigraphy.131 Pitfalls include spurious results in patients with malabsorptive conditions, liver diseases or lung diseases that may preclude normal oxidation and excretion of the C13-labeled foods.
Ultrasonography
Transabdominal ultrasonographic techniques are used to measure antral diameter and antropyloroduodenal function.132,133 Three-dimensional ultrasound methods show the intragastric distribution of the test meal and regional variations in gastric volume (see Fig. 48-13).58 The technique is ideal with a liquid meal, but solids can also be identified and gastric emptying rates can be determined. The clinical application is limited by the high level of expertise required by the ultrasound operators.
Computed Tomography and Magnetic Resonance Imaging
These two techniques have been used to measure gastric emptying and demonstrate intragastric distribution of test meals. Computed tomography (CT) and magnetic resonance imaging (MRI) technologies offer unique anatomic and functional views of the stomach in the fasting and postprandial periods.134 Sequential antral contractions can be visualized. Because of expense and availability, these techniques are not used in clinical practice.
GASTRIC CONTRACTIONS
Antroduodenal Manometry
Antroduodenal manometry is an invasive technique wherein a water-perfused multilumen catheter is placed either through the nose or the mouth and advanced to a position where the proximal catheter ports are in the distal antrum and the distal ports are in the duodenum.40 Placement of the catheter requires endoscopic or fluoroscopic aid. The recordings typically last for several hours in order to record phases 1, 2, and 3 of the MMC and several more hours to record postprandial contractions after the subject ingests a test meal (see Fig. 48-7A and B). Antroduodenal manometry testing is not only invasive but also time intensive and requires extensive assistant or physician time for performance of the test and interpretation of the data. Intraluminal manometry catheters detect only lumen-occluding contractions.136 Intraluminal pressure transducer devices fail to record almost 50% of contractions in the corpus and antrum because the majority of postprandial peristaltic waves are not lumen-occluding contractions. Manometry catheters positioned in the duodenum can detect patterns of neuropathic or myopathic dysfunction.
Capsule Technology
The ingestible capsule described previously measures contractions of the stomach wall. After a standard test meal, irregular contractions occur at one to three per minute and are consistent with manometric recordings from the antrum.137 Several minutes of sustained high-amplitude, antral contractions occur prior to the emptying of the capsule into the duodenum (see Fig. 48-12) and some patterns are consistent with phase 3–like contractions recorded by antroduodenal manometry. In patients with gastroparesis, capsule studies showed a decreased motility index during the 20 to 30 minutes before emptying of the capsule, but a normal motility index in the 10 minutes before the capsule was emptied into the duodenum. These studies suggest that normal terminal antral contractions may be maintained even in patients with gastroparesis, whereas antral contractility required for trituration of digestible foodstuffs is abnormal.138 Determination of the unique gastric contraction patterns after ingestion of a variety of common foods is possible using the capsule motility device.
GASTRIC MYOELECTRICAL ACTIVITY
Electrogastrography (EGG) refers to the recording methods used to noninvasively measure gastric myoelectrical activity using electrodes positioned on the abdominal surface.139,140 The EGG signal summates the ongoing gastric myoelectrical activity. The EGG reflects the slow wave activity during fasting and the summation of slow wave activity linked to plateau and action potential activity during the postprandial period (see Figs. 48-1 and 48-2).140 In response to a water load or a nutrient load, the amplitude of the EGG signal increases in the normal 2.5 to 3.7 cpm range, as determined by visual and computer analysis (e.g., an increase in the percentage of EGG power or in the postprandial power ratio in the normal frequency range) (see Fig. 48-15).60,140,141 Pitfalls for recording and analyzing EGGs include failure to identify artifact in the signal and harmonics in the computer analyses.142
Changes in the EGG frequency and amplitude are key measures. After ingestion of most solid or liquid meals, a so-called frequency dip occurs in the first 10 to 15 minutes after the meal. The frequency dip reflects changes resulting from marked gastric relaxation and accommodation of the test meal related to the volume or the temperature of the meal.143,144 Several minutes after ingestion of the meal, the frequency of the EGG signal returns to the middle of the normal 2.5 to 3.7 cpm range (see Fig. 48-15).
Gastric dysrhythmias are associated with symptoms of nausea in subjects with motion sickness,89 nausea and vomiting of pregnancy,145,146 functional dyspepsia,60,147 and gastroparesis.148–150 Gastric dysrhythmias include 0.5 to 2.5 cpm signals termed bradygastrias and 3.7 to 10 cpm signals termed tachygastrias (Fig. 48-19). Recordings that have combinations of tachygastria and bradygastrias are termed mixed or nonspecific gastric dysrhythmias.151 Gastric myoelectrical activity can also be recorded from serosal electrodes placed during surgery or with mucosal electrodes placed during endoscopy.6,74,152
GASTRIC RELAXATION, ACCOMMODATION, AND VOLUME
Barostat Tests
Due to the spherical shape of the fundus and the proximal stomach, manometric catheters to record intraluminal pressures after meals are not useful. The barostat balloon was designed to measure changes in tone (or gastric relaxation) and volume in the more spherical areas of the proximal stomach.153 Barostat methods involve a large, collapsed thin-walled balloon that is mounted on a catheter and passed through the mouth into the stomach. Intraballoon pressure is maintained with infused air during the baseline or fasting period with the balloon slightly distended. A test meal is then ingested. As the fundus and proximal stomach relax in response to the meal, more air is concomitantly infused into the balloon to maintain the established baseline intraballoon pressure (see Fig. 48-9).154 The volume of air that is infused to maintain baseline pressure is measured and is an estimate of the increased gastric volume that occurs as the proximal stomach relaxes.
Barostat studies demonstrate abnormalities in fundic relaxation in almost 30% of patients with functional dyspepsia.155 The failure of fundic relaxation correlates with early satiety. Failure of fundic relaxation has also been recorded in patients with gastroparesis of diverse causes.156,157 Because the barostat method is invasive and uncomfortable for patients, these studies have been limited to the research laboratory.
Scintigraphy and Other Tests
Excessive or poor fundic relaxation in response to liquids and solids can be demonstrated with scintigraphy, ultrasound, and MRI. Single photon emission computed tomography (SPECT) is a method that outlines the gastric wall before and after ingestion of a meal to determine changes in volume of the stomach. This method requires intravenous injection of 99mTc-pertechnetate to outline the gastric wall. The accommodation response can be identified with SPECT.158,159
Non-Nutrient Liquid and Nutrient Drink Tests
Non-nutrient liquids (the water-load test) and nutrient drink tests are used to assess overall gastric volume or gastric capacity and visceral sensations such as nausea, stomach fullness, or satiety in response to ingestion of these liquids,60,97,160 often in conjunction with measures of gastric myoelectrical activity, accommodation, or emptying. In the water-load test, water is consumed over a 5-minute period until the subject feels full. In the typical caloric drink test, subjects drink 150 mL of the liquid (e.g., Ensure) every 5 minutes until maximum tolerated satiety is achieved, an endpoint that requires almost 30 minutes and the consumption of 800 to 1000 mL of the nutrient drink.161 In many healthy subjects, nausea and gastric dysrhythmia are evoked by the satiety drink test. Subjects with functional dyspepsia or gastroparesis ingest much smaller volumes of water or nutrient drink and report fullness, indicating impaired relaxation and accommodation of the stomach.60,97
HISTOPATHOLOGIC STUDIES IN GASTRIC NEUROMUSCULAR DISORDERS
Efforts to define the histopathologic basis of gastric neuromuscular disorders have provided basic knowledge for the evolving field of neurogastroenterology. Most of the full-thickness specimens from the gastric wall have been harvested during the placement of gastric electrical stimulation devices or the placement of jejunostomy feeding tubes in patients with severe gastroparesis. Mucosal biopsies do not typically contain smooth muscle, ICCs, or ENS neurons, but an endoscopic biopsy technique has been described that provides a full-thickness specimen that contains elements of circular muscle, ENS neurons, and ICCs.162 Histologic abnormalities in smooth muscle, neurons of the ENS, number or location of ICCs, and distribution of key neural or muscular receptors are possible underlying mechanisms of disordered gastric smooth muscle relaxation, peristaltic contraction, and gastric slow wave activity.
Loss of ICCs (MY-ICCs) was reported in patients with type 1 and type 2 diabetic gastroparesis.25,163,164 Interestingly, similar loss of ICCs was shown in diabetic mice, and the ICCs were restored with intense insulin therapy, suggesting that the ICCs are not entirely destroyed in diabetes but dedifferentiate into immature myoblasts during prolonged hyperglycemia.164 Damage to ICCs, ENS, and smooth muscle can also be seen in inflammatory and neoplastic conditions.165–168 Other studies in humans have shown fibrosis of smooth muscle layers but intact myenteric plexus and vagus nerve in patients with diabetic gastroparesis, loss of ICCs but minimal smooth muscle fibrosis, and inflammatory T lymphocyte infiltration in myenteric neurons.169–171 Pitfalls with neurohistologic studies include tissue sampling of variably affected regions of the stomach, with patchy distribution abnormalities of ICCs, ENS neurons, or smooth muscle. Nevertheless, results from histochemical studies are needed to provide new directions for understanding the neuromuscular dysfunction of the stomach and to stimulate ideas for novel therapeutic approaches.
NEUROMUSCULAR DISORDERS OF THE STOMACH
Gastric neuromuscular disorders encompass a continuum of electrical and contractile dysfunction.172 At one end of the spectrum are gastric dysrhythmias, which are subtle electrical disturbances associated with mild to severe nausea symptoms (Fig. 48-20). Abnormalities in relaxation of the fundus are associated with early satiety. At the severe end of the spectrum, antral hypomotility and profound gastroparesis are associated with prolonged postprandial fullness, vomiting, bloating, weight loss, and malnutrition that may require enteral or parenteral nutritional support. Patients with gastroparesis may also have gastric dysrhythmias, a dilated antrum, poor fundic relaxation, and gastric hyper- or hyposensitivity due to vagal or sphlanchnic nerve dysfunction.172,173 Clinical tests currently approved by the U.S. Food and Drug Administration (FDA) to assess gastric neuromuscular function are the scintigraphy tests to measure the rate of gastric emptying, the capsule motility device to measure gastric emptying, gastric pH, and contraction patterns of the stomach, and electrogastrography devices to measure gastric myoelectrical activity before and after provocative test meals. These tests provide objective assessments of different aspects of the neuromuscular activity of the stomach in health and disease. Results of gastric emptying and gastric myoelectrical activity tests provide objective diagnoses of gastric dysrhythmias and gastroparesis.
GASTROPARESIS
Diabetic Gastroparesis
Fifty percent of patients with long-standing type 1 diabetes mellitus develop gastroparesis. These patients often have diabetes for more than 10 years, erratic and elevated glucose levels, peripheral neuropathy, nephropathy, and cardiovascular disease.174–176 In a minority of patients the gastroparesis is the initial complication of their diabetes. Upper GI symptoms, however, may be minimal and nonspecific. This is not surprising because patients with diabetes often have ischemic heart disease with minimal chest discomfort or cholecystitis with minimal or no right upper quadrant pain.
Acute hyperglycemia (>220 mg/dL) is associated with tachygastrias and delayed gastric emptying in healthy subjects and in patients with diabetes.73,175,177–179 Hyperglycemia is also associated with loss of ICCs,25,163 antral hypomotility,180 isolated pyloric contractions,181 gastric dysrhythmias,6,177,178 and impaired prokinetic action of prokinetic drugs like erythromycin.182 Relatively minor increases in the blood sugar level, even elevations within the physiologic range, delay gastric emptying in normal volunteers and diabetic patients.179 Acute hyperglycemia produced by glucose clamp methodology elicits fullness, decreased antral contractility, blunts the contractile pyloric response to intraduodenal lipid infusion, and modifies upper GI sensations.183,184
Gastric neuromuscular abnormalities documented in patients with chronic type 1 diabetes include abnormal intragastric distribution of food,185 reduced receptive relaxation and accommodation,186 reduced incidence of the antral component of the MMC, antral dilation, postprandial antral hypomotility,176 and electrical dysrhythmias.148,177,187 Loss of the antral phase 3 contractions results in poor emptying of fibrous debris in the stomach and is the neuromuscular basis for the formation of bezoars (see Chapter 25). Thus, the progressive neuromuscular dysfunction of the diabetic stomach reflects the effects of chronic hyperglycemia and superimposed, intermittent hyperglycemia episodes that occur repeatedly over many years.
Gastric smooth muscle dysfunction is another mechanism of delayed gastric emptying in some patients with diabetes. In rats with diabetes gastric smooth muscle contractility is reduced in response to electrical stimulation.188 The inhibitory effect of hyperglycemia on stem cell factor has a role in the reduction in ICCs and smooth muscle contractility.189 Pylorospasm as a cause of gastroparesis was documented in one study.190 Some patients with pylorospasm have a form of obstructive gastroparesis; that is, the neuromuscular function of the corpus and antrum is intact, but persistent functional obstruction at the pylorus (e.g., pylorospasm) causes delayed emptying. These patients have normal or increased 3 cpm myoelectrical activity, a discordant finding in patients with gastroparesis that also suggests the possibility of gastric outlet obstruction (Fig. 48-21A).149
In type 2 diabetes mellitus the incidence of gastroparesis ranges from 30% to almost 50%.191 Patients with type 2 diabetes differ significantly from patients with type 1 diabetes in that they have insulin resistance, the diabetes appears later in life, and the hyperglycemia has been present longer before diagnosis compared with patients with type 1 diabetes.193 Almost 20 million people in the United States have type 2 diabetes mellitus, and many have unsuspected gastroparesis.192 Gastroparesis in patients with type 2 diabetes presents with subtle dyspepsia-like symptoms, but a minority of patients may present acutely with nausea, vomiting, and severe gastroparesis. Gastric dysrhythmias have been recorded in up to 75% of patients with type 2 diabetes with an average hemoglobin A1C of 8.2.193 In the early stages of type 2 diabetes mellitus gastric emptying may be accelerated.194
Gastroparesis has been also described in experimental diabetes, specifically in nonobese diabetic mice and in the type 2–like diabetes db/db mouse. Studies by Watkins and associates showed that neuronal nitric oxide synthase (nNOS) was reduced in the diabetic mouse stomach and was associated with antropylorospasm and gastroparesis.195 Treatment with insulin or sildenafil restored NOS and was associated with reversal of the delay in gastric emptying. Hypomotility of the fundus and hypercontractility of the pylorus were found in db/db mice.196 In other studies of diabetic mice the loss of ICCs was associated with electrical dysrhythmias, delayed gastric emptying, and reduced neurotransmission in gastric smooth muscle.197 Chronic hyperglycemia also leads to glycosolation metabolic end products that interfere with neural function and smooth muscle contraction.
Postsurgical Gastroparesis
Gastroparesis occurs in a subset of patients undergoing subtle or radical stomach operations that range from vagotomy to fundoplication to antrectomy. Truncal vagotomy produces complex effects on the neuromuscular function of the stomach. After vagotomy, the fundus fails to relax normally after meals, resulting in rapid filling of the antrum.198 Vagotomy is also associated with gastric dysrhythmias,3 decreased antral contractions, and poor antropyloroduodenal coordination.199 Truncal vagotomy performed during ulcer operations required a pyloroplasty to reduce sphincteric resistance to outflow because antral contractions were weak and peristalsis was disrupted.200 Most patients recover from the effects of vagotomy, but in patients undergoing extensive resection of the antrum and corpus, prolonged symptoms and chronic gastric neuromuscular dysfunction are likely.
During fundectomy and lower esophageal resection for esophageal cancer, the vagus nerves are transected and the fundic reservoir is lost. Although pyloroplasty is performed to facilitate gastric emptying, the loss of the fundus and variable amounts of the corpus (pacemaker region) often leads to chronic nausea, gastric dysrhythmias, and gastroparesis. Antral resections with Billroth I, Billroth II, or Roux-en-Y gastrojejunostomy are performed to treat gastric tumors or peptic ulcer disease, but these operations may lead to profound neuromuscular dysfunction of the stomach.201,202 Critical amounts of the corpus-antrum (including unknown amounts of the gastric wall containing the pacemaker region) required for normal gastric neuromuscular activity may be resected and the efficacy of trituration by the corpus and antrum may be markedly reduced. Ingested food is retained in the remnant fundus and fails to empty into the corpus203; the corpus fails to mix and empty gastric contents even though the anastomosis is widely patent. Liquids empty poorly into the duodenal or jejunal segments if no peristaltic contractions are present. The Roux-en-Y gastroenterostomy operation may result in the Roux syndrome in which postprandial pain, bloating, and nausea develop. Delayed gastric emptying is due to “functional obstruction” by the Roux limb as the neuromuscular dyssynchrony within the Roux limb prevents emptying of the stomach.204,205 After vagotomy and antrectomy, a minority of patients develop the dumping syndrome described below.
Fundoplication is commonly performed to treat gastroesophageal reflux disease that fails to respond to medical therapy (see Chapter 43). Postfundoplication gastroparesis and early satiety, bloating, prolonged fullness, and nausea occur in a minority of patients.206 These patients have altered fundic relaxation, delayed gastric emptying, and gastric dysrhythmias, possibly on the basis of vagal nerve injury during or after the fundoplication procedure.207–209 Because gastric emptying studies are infrequently performed before this operation, it is not known how many of the patients already had gastroparesis before the fundoplication. In some patients the fundoplication results in rapid filing of the antrum (due to poor relaxation of the fundus) and the rate of gastric emptying is increased.210
Ischemic Gastroparesis
Chronic mesenteric ischemia may cause ischemic gastroparesis.211 These patients present with chronic symptoms of gastroparesis. Ischemic gastroparesis is distinct from acute mesenteric ischemia, which presents as an abdominal catastrophe with an acute abdomen and gangrenous small intestine (see Chapter 114). Chronic mesenteric ischemia is usually due to progressive atherosclerosis or hyperplasia of the intima of the arteries of the celiac, superior mesenteric, or inferior mesenteric artery. Collaterals of these obstructed arteries form over time so that neuromuscular function of the stomach is preserved, at least for some time. Bypass graft surgery or dilatation of the stenotic arteries results in resolution of symptoms, eradication of gastric dysrhythmias, and reversal of gastroparesis.211 Thus, ischemic gastroparesis is a reversible form of gastroparesis and should be suspected in patients with gastroparesis, weight loss, and a history of peripheral vascular disease, cerebral vascular disease, or myocardial infarction. An abdominal bruit is present in approximately 50% of patients.
There are other uncommon forms of mesenteric vascular compromise (e.g., the median arcuate ligament syndrome) that may result in decreased blood flow to the stomach.212 Release of the arcuate ligament and restoration of blood flow has been associated with improvement in gastric emptying. On the other hand, superior mesenteric artery syndrome is not accepted as a cause of mechanical obstruction that leads to gastroparesis, nausea, and vomiting.
Obstructive Gastroparesis
Obstructive gastroparesis refers to mechanical obstruction at the pylorus, duodenum, or postduodenal area by tumor, chronic peptic ulcer or inflammation, rings, or webs.149 These patients have documented gastroparesis, but the EGG signal is normal and the amplitude of the 3 cpm EGG wave after the water-load test is increased.149 Figure 48-21A shows an example of high-amplitude 3 cpm EGG waves in a patient with pyloric outlet obstruction due to chronic peptic ulcer disease and fixed narrowing of the pylorus. In these patients the smooth muscle, ENS, and ICCs of the antrum are intact, but the recurrent gastric peristaltic waves meet sustained resistance at the point of obstruction (the pylorus) and the emptying of solid food is delayed. In contrast, patients with idiopathic gastroparesis have bradygastria, tachygastria, or mixed gastric dysrhythmias indicating electrical and contractile dysfunction (see Fig. 48-21B). Surgical correction of the obstruction with Billroth I or Billroth II gastrojejunostomy is necessary to correct obstructive gastroparesis, although some patients may respond to balloon dilation of strictures (see Chapter 53). In patients who have had prolonged obstruction, the stomach may dilate, smooth muscle contractions are weak, and gastric dysrhythmias are present.
A more subtle type of gastric outlet obstruction occurs in pylorospasm. The sustained pyloric “spasm” or tone may cause right upper quadrant abdominal pain and prevents normal gastric peristaltic waves from empting chyme into the duodenum. Thus, the rate of emptying is delayed and gastroparesis is present. These patients also have normal 3 cpm EGG rhythm because the neuromuscular apparatus of the corpus-antrum is intact. Dilatation of the pylorus with a 20-mm balloon for two minutes decreased postprandial symptoms and improved the rate of gastric emptying.213 Finally, some patients have normal 3 cpm EGG signals and poor gastric emptying on the basis of “electromechanical dissociation.” In these situations it is possible that MY-ICCs that generate slow waves may be normal, but IM-ICCs and/or smooth muscle dysfunction is present.
Idiopathic Gastroparesis
Almost one third of patients with gastroparesis have idiopathic gastroparesis.214 In many of these patients an acute febrile illness preceded the diagnosis of gastroparesis by many months.215,216 These “herald” illnesses are frequently described as flu-like with fever and nausea and vomiting. Patients often date the onset of their nausea from that point. Norwalk virus, herpes simplex virus, and Epstein-Barr virus infections have been documented in patients with sudden onset gastroparesis and normal immune systems, whereas other patients are immunocompromised.217 Postviral gastroparesis may resolve completely over one to two years. It is unclear if the offending virus affects ICCs, smooth muscle or the ENS of the stomach, or vagal or splanchnic nerves, but these cases are analogous to postviral cardiomypathy or postviral neuropathy. Degeneration and loss of ICCs has been reported in patients with severe idiopathic gastroparesis.165,218 Loss of ICCs in knockout mice is associated with gastric dysrhythmias.219 Gastric dysrhythmias are common in patients with idiopathic gastroparesis (see Fig. 48-21B).149 Other historical clues to the genesis of idiopathic gastroparesis are exposures to (1) multiple courses of antibiotics (for example, treatments for chronic ear or sinus infections), (2) anesthetic agents for a variety of common operations, and (3) food poisoning–like illnesses of unknown cause.
GASTRIC NEUROMUSCULAR DYSFUNCTION ASSOCIATED WITH OTHER GASTROINTESTINAL DISORDERS
Functional Dyspepsia
Functional dyspepsia (FD) symptoms include epigastric discomfort, early satiety, fullness, nausea, and vomiting in the setting of normal upper endoscopy and routine laboratory tests. FD is divided further into an epigastric pain syndrome and postprandial distress syndrome, the latter comprising 80% of the FD patients.220 One of the dominant symptoms that patients with FD have is recurrent and unexplained nausea. This is a debilitating symptom with a large differential diagnosis (Table 48-2) and these disease categories should be considered in the evaluation of the patient. If the patient has one of these disorders, then FD is not the diagnosis.
Table 48-2 Differential Diagnosis of Chronic Nausea and Vomiting
The pathophysiology of the FD symptoms remains an area of intensive investigation (see Chapter 13). The FD symptoms may be caused by one of several neuromuscular disorders of the stomach as shown in Figure 48-20. Gastroparesis is found in 17% to 40% and gastric dysrhythmias in 40% to 55% of patients with FD symptoms.60,147 If secondary causes for gastroparesis have been excluded, then these patients have idiopathic gastroparesis and/or gastric dysrhythmias, not FD.
The rate of gastric emptying is normal in the majority of patients with FD, but gastric dysrhythmias may be present in these patients. In one series of patients with FD, 35% had gastric dysrhythmias and normal gastric emptying.63 Improvement in gastric dysrhythmia and FD symptoms was reported in patients treated with cisapride,221,222 and similar results were reported in children and adults with dyspepsia.223,224 Thus, gastric dysrhythmias themselves are the cause of some of the FD symptoms and are a therapeutic target.
Gastric neuromuscular disorders such as abnormalities in gastric accommodation or gastric hypersensitivity to distention may account for FD symptoms in other patients.225–230 Thus, a variety of neuromuscular dysfunctions in different regions of the stomach may be present in patients with FD symptoms.231 Exposure of the duodenal mucosa to acid is also a proposed mechanism of FD symptoms.232 Increasing data that link certain dyspepsia symptoms such as nausea or bloating or discomfort to distinct neuromuscular abnormalities of the stomach will aid in improved diagnosis and treatment of these patients.
Gastroesophageal Reflux Disease
Gastroesophageal reflux disease (GERD) typically presents with heartburn and regurgitation, but many patients have additional symptoms of early satiety, postprandial fullness, and nausea (see Chapter 43). Approximately 30% of patients with GERD also have FD and represent an “overlap syndrome.”233 Furthermore, 20% to 30% of patients with GERD have delayed gastric emptying.234,235 Twenty-five percent of patients with GERD and dyspepsia had delayed gastric emptying and almost 70% had gastric dysrhythmias.236 The FD-like symptoms do not resolve when the GERD symptoms are treated with proton pump inhibitors (PPIs) because the gastric neuromuscular disorder is not treated by PPIs.
Lower esophageal sphincter abnormalities, including transient lower esophageal sphincter relaxations, may contribute to inefficient gastric emptying and gastric dysrhythmias reported in these patients.237 In response to a meal, the fundus relaxes more in GERD patients compared with controls.238 GERD patients also tended to retain solids and liquids in the proximal stomach compared with control subjects,239 an abnormality that may stimulate additional transient lower esophageal sphincter relaxations.
Radiofrequency ablation (RFA) treatment at the lower esophageal sphincter region in patients with heartburn and dyspepsia improved heartburn, gastric emptying rates, and gastric dysrhythmias.240,241 These data suggest in some patients with GERD and gastroparesis, treatments of GERD by RFA or fundoplication improves the gastric electrical rhythms and the rate of gastric emptying.
Constipation, Irritable Bowel Syndrome, and Pseudo-Obstruction
Gastroparesis and gastric dysrhythmias after the water-load test have been reported in 20% to 30% and 60% of patients with constipation-predominant irritable bowel syndrome, respectively.242,243 These patients represent another overlap syndrome of gastrointestinal neuromuscular disorders (see Chapter 118). GERD, gastroparesis/gastric dysrhythmias, and irritable bowel syndrome are all present in some patients and indicate a disorder of diffuse gastrointestinal neuromuscular dysfunction.233
Patients with intestinal pseudo-obstruction syndromes often have GERD, gastroparesis, small bowel dysmotility, and colonic inertia and reflect the severest form of generalized GI neuromuscular disorders.244,245 Pseudo-obstruction secondary to scleroderma commonly involves the esophagus and stomach but may also cause small bowel dysmotility and subsequent bacterial overgrowth.246 Intestinal pseudo-obstruction may be due to idiopathic degenerative or inflammatory processes involving the smooth muscle or enteric nervous system, as discussed in Chapter 120.
A variety of neurologic diseases may also involve the stomach or other regions of the digestive tract and cause pseudo-obstruction–like symptoms. These neurologic disorders include spinal cord injuries, head injuries, amyotrophic lateral sclerosis, myasthenia gravis, a variety of muscular dystrophies, and Parkinson’s disease. Chapter 35 discusses stomach neuromuscular dysfunction and autonomic neuropathies.
Miscellaneous Conditions
Patients with FD symptoms and cirrhosis with portal hypertension,247 chronic kidney disease,248 chronic pancreatitis,249 or advanced human immunodeficiency virus (HIV) infections250 may have underlying gastroparesis. Patients with the Rett syndrome, which includes lack of development, autistic behavior, ataxia, and dementia in young girls, frequently have failure to thrive and significant gastroparesis and esophageal contraction abnormalities.251 Patients with a variety of cancers may have local and systemic effects on the stomach neuromuscular apparatus that results in gastroparesis.252,253 The neoplastic neuropathic syndromes and the effects of cytotoxic chemotherapy and radiation may result in gastroparesis and affect the patient’s nutrition and intravascular volume status.
H. pylori infection does not affect the rate of gastric emptying. On the other hand, abnormal gastric myoelectrical activity has been reported in patients with H. pylori infection, and the dysrhythmias disappeared after eradication of H. pylori.254 Gastric emptying is delayed in patients with anorexia nervosa,255 but is normal in patients with bulimia.256 Cyclic vomiting syndrome (CVS) is an unusual entity in that days of profound and unremitting nausea and vomiting (that requires hospitalization) are followed by many days or months with virtually no GI symptoms.257 CVS occurs in adults as well as in infants.257,258 When patients are well, EGG abnormalities are present and some of these patients have gastroparesis.
DUMPING SYNDROME AND RAPID GASTRIC EMPTYING
Dumping syndrome occurs in some patients who have had vagotomy and pyloroplasty or Billroth I or Billroth II gastrojejunostomy.259 In these patients the ingested foods are not accommodated and retained in the fundus (because of poor fundic relaxation) and the foods are not normally triturated because the corpus and antrum have been resected. Thus, liquid and solid nutrients are rapidly emptied or “dumped” into the duodenum or jejunum. Symptoms of dumping syndrome include nonspecific abdominal discomfort, bloating, and nausea that may precipitate vomiting. These symptoms are usually experienced in the first hour after ingestion of foods and can mimic symptoms of gastroparesis. Sweating and lightheadedness, however, may occur and be followed by abdominal cramps and diarrhea that occur two to four hours after the meal and are clues to the dumping syndrome. Early symptoms are due to the distention of the small bowel, whereas symptoms that occur later are due to rapid absorption of carbohydrates and hyperglycemia that is poorly matched in time with secretion of insulin. This mismatch of plasma glucose and insulin results in symptomatic hypoglycemia. The rapid small bowel transit and poor absorption of the ingested nutrients lead to an osmotic form of diarrhea.260
Patients with no history of gastric operations may also have rapid gastric emptying (dumping syndrome),259 including patients with FD.260 Rapid gastric emptying is present when more than 30% of the meal (a low-fat egg substitute meal) is emptied in 30 minutes, or more than 70% is emptied at 60 minutes.128 Idiopathic abnormally rapid emptying is diagnosed in patients with no history of gastric operations or other causes. Rapid gastric emptying is also associated with early stages of type 2 diabetes mellitus (see earlier), Zollinger-Ellison syndrome, and the variety of gastric surgeries described earlier. Details of these entities is reviewed under the postsurgical syndromes in Chapter 53.
DIAGNOSIS
SYMPTOMS
Most affected patients have few upper GI symptoms when they are fasting. However, the ingestion of meals stimulates the disordered gastric neuromuscular apparatus, and early satiety, prolonged epigastric fullness, nonspecific epigastric discomfort, mild to severe nausea, and vomiting are experienced.233 These are also the symptoms associated with functional dyspepsia (Chapter 13). Vomitus that contains undigested, chewed food is strong evidence for gastroparesis. Prolonged postprandial fullness, weight loss, and female gender are predictive factors for gastroparesis.261 By adjusting their diet, patients learn to reduce these postprandial symptoms and carry on for months or years before they seek medical attention or their physicians recognize the possibility of gastroparesis. The Gastroparesis Cardinal Symptom Index is a useful validated questionnaire to quantify gastroparesis symptoms.262 Persistent nausea is one of the most noxious symptoms of the gastric neuromuscular disorders. A thorough review of the causes of nausea and vomiting is required (Chapter 14) and an appropriate differential diagnosis should be considered (see Table 48-2).263
In the evaluation of unexplained nausea and vomiting, gastric neuromuscular dysfunction must be distinguished from esophageal diseases and rumination syndrome. Occult GERD may present as unexplained nausea because these patients report little or no heartburn.264 Regurgitation is the gentle delivery of gastric content into the esophagus and pharynx (and the content is sometimes reswallowed), whereas vomiting is the forceful ejection of gastric contents from the mouth. Rumination refers to the effortless return of ingested liquids and solid foods into the mouth without burning, bitter taste, or nausea. Patients with rumination have impaired gastric accommodation and a more sensitive relaxation of the lower esophageal sphincter pressure in response to gastric distention.265–267 Rumination occurs in healthy adolescents and young adults, but was previously recognized among children with neural and developmental disorders.
Abdominal pain, in contrast to the abdominal discomfort of bloating and nausea, occurs infrequently in patients with gastric neuromuscular disorders. Any recurrent abdominal pain syndrome should be worked up extensively because the nausea and vomiting may be secondary to the specific cause of the pain (e.g., burning epigastric pain associated with nausea and vomiting may be due to active peptic ulcer disease). Once the peptic ulcer disease is diagnosed and treated, the pain and nausea disappear. A specific pain syndrome may suggest diagnoses such as cholecystitis, pancreatitis, or sphincter of Oddi dysfunction. On the other hand, the epigastric discomfort or pain in some patients may originate from excessive muscle tone of the fundus, high-amplitude antral contractions, pylorospasm, or hypersensitivity of the stomach.268 Recurrent retching and vomiting may result in abdominal pain, which is a result of abdominal muscle and rib tenderness or the abdominal wall syndrome.269,270
PHYSICAL EXAMINATION
The general examination may be normal or reveal signs of volume depletion, weight loss, and poor nutrition. Inspection of dentition may show erosion of enamel associated with chronic GERD or bulimia. Abdominal examination may detect masses, organomegaly, and areas of tenderness. Abdominal distention and succession splash may be present. Auscultation over the epigastrium may detect bruits that indicate stenoses of the celiac or superior mesenteric arteries. Tenderness that is well localized at healed incisions and persists when the anterior abdominal muscles are contracted (Carnett’s sign) suggests an abdominal wall syndrome.269,270 Neurologic examination may reveal nystagmus, facial weakness, ataxia, or other abnormalities.
STANDARD TESTS
Common causes of nausea and vomiting and dyspepsia symptoms are excluded by normal upper gastrointestinal (GI) series, CT of the abdomen and head, routine laboratory studies, and upper endoscopy.271 In patients with dyspepsia, gastric cancers are detected in less than 1% of the patients undergoing upper endoscopy.272 Most patients seen by gastroenterologists for dyspepsia or chronic nausea have already received acid-suppression therapy with PPIs, but symptoms persist. In such patients, gastric neuromuscular disorders should be considered.
SPECIALIZED NONINVASIVE TESTS
An objective diagnosis of neuromuscular disorders of the stomach can be established by the results of gastric emptying tests and EGG. Gastric emptying tests and EGG are complementary in defining different aspects of gastric neuromuscular disorders (Table 48-3).147,271 By combining the results of gastric emptying tests and EGG, four pathophysiologic categories of gastric neuromuscular function are defined and rational treatment approaches can be designed. The categories are (1) gastroparesis with gastric dysrhythmia; (2) normal gastric emptying with gastric dysrhythmia; (3) normal gastric emptying with normal gastric electrical rhythm; and (4) gastroparesis with normal gastric electrical rhythm. The EGG testing device is cleared by the FDA, but the combination of EGG and gastric emptying testing is infrequently performed. Nevertheless, the four categories provide a conceptual framework for understanding the spectrum of gastric neuromuscular disorders and providing an approach to therapy (see Table 48-3).
Table 48-3 Categories of Gastric Neuromuscular Disorders and Treatment Approaches Based on Gastric Electrical and Emptying Test Results
Category 1 patients have severe neuromuscular dysfunction with gastroparesis and gastric dysrhythmias such as tachygastria. Among patients with “functional dyspepsia,” 17% to 32% have gastric dysrhythmias and gastroparesis.60,147,271 Patients in category 1 often have more severe symptoms, require many drugs, and may require venting gastrostomies, enteral feeding, and gastric pacing, as discussed following.
Among patients with functional dyspepsia, 40% to 60% have gastric dysrhythmias despite normal gastric emptying. Such category 2 patients had a significantly better response to the prokinetic agent cisapride than patients with normal EGG recordings.221
In patients with normal gastric myoelectrical activity and normal gastric emptying (category 3), the nausea symptoms are likely due to visceral hypersensitivity or nongastric causes. Nongastric diagnoses should be considered in this patient group. Atypical GERD may cause nausea, and a 24-hour pH study will confirm the relationship.264 A CCK-stimulated gallbladder emptying study may document gallbladder dysfunction in the absence of cholelithiasis. If postprandial abdominal discomfort and disturbed bowel function components are present, then irritable bowel syndrome should be considered. Central nervous system causes of nausea also should be assessed.
Patients who have gastroparesis and normal or high-amplitude 3 cpm EGG signals (category 4) may have mechanical obstructions of the stomach and duodenum that are reversible with operation.149 Alternatively, patients with a normal-amplitude 3 cpm pattern and gastroparesis may have a form of “electromechanical dissociation,” requiring medical therapy.
TREATMENT
By grouping patients on the basis of the gastric emptying and myoelectrical results, the pathophysiologic findings can help in the understanding of symptoms and assist in developing an approach to treatment (see Table 48-3). If delayed gastric emptying is confirmed, then the major causes of gastroparesis should be reviewed to specifically define the cause of the gastroparesis (see Table 48-2). The reversible causes of gastroparesis, gastric outlet obstruction, and chronic mesenteric ischemia, should be excluded. If gastric emptying is normal, then gastric dysrhythmias and gastric accommodation disorders may be the neuromuscular disorders that are relevant to the symptoms. Treatment of gastric neuromuscular disorders is problematic. Scant specific therapies are available to address specific pathophysiologic disorders. Treatments listed in Table 48-4 reflect the broad but limited armamentarium that ranges from prokinetic agents to diet counseling and electrical therapies.
Table 48-4 Drug and Nondrug Therapies Used to Treat Symptoms in Patients with Gastric Neuromuscular Disorders
DRUG THERAPY
Prokinetic Agents for Corpus-Antrum
Drugs with prokinetic effects on gastric contractility and gastric dysrhythmias are usually prescribed for patients in categories 1 and 2 (see Table 48-4). Patients who have gastroparesis and tachygastria have severe electrical and contractile abnormalities of the stomach. The treatment includes prokinetic, antinauseant therapies, and dietary counseling.271
Erythromycin is a macrolide antibiotic and motilin-like molecule that increases gastric emptying by stimulating strong phase 3–like antral contractions.273,274 Erythromycin, however, often increases nausea and vomiting symptoms. Metoclopramide, a substituted benzamide related to procainamide, is a useful prokinetic antiemetic. However, metoclopramide also causes depression, extrapyramidal side effects, and irreversible tardive dyskinesia.275,276 Domperidone is a dopamine antagonist that decreases nausea, corrects gastric dysrhythmias, and increases gastric emptying rates.277,278 Domperidone may be obtained through a new drug application process with the FDA. Cisapride and tegaserod are 5-HT4 agonists and were not approved for gastric emptying disorders, but these drugs increase gastric emptying rates and decrease dyspepsia symptoms in some patients.279–281 Cisapride and tegaserod were withdrawn from the market but are available through special FDA programs.
Prorelaxant Agents for Fundus and Pylorus
Drugs that relax the fundus are few. Sumitriptan, a 5-HT1 antagonist, decreases fundic tone but was not better than placebo in reducing symptoms in patients with FD.282 Trials of dicyclomine or calcium channel blockers to decrease fundic tone have not been reported. Botulinum toxin relaxes the pyloric sphincter pressure and is described below.
Antinauseant Therapy
Nonspecific approaches to treating nausea and vomiting from gastric neuromuscular disorders include 5-HT3 serotonin antagonists such as ondansetron and granisetron (see Table 48-4). These agents, as well as the phenothiazines and antihistamines such as promethazine, dimenhydrinate, and cyclizine, are often used for these symptoms, but there are no controlled trials in patients with gastric neuromuscular disorders. Lorazepam or alprazolam or other antianxiety medications reduce nausea in some patients.283 An uncontrolled trial of tricyclic antidepressants such as amitriptyline alleviated nausea in approximately 70% of patients with unexplained nausea.284
ELECTRICAL THERAPY
Acustimulation
Acustimulation (mild electrical stimulation of acupuncture points) reduces nausea of pregnancy, nausea due to chemotherapy agents, postoperative nausea, and the nausea of motion sickness.285,286 These treatment methods have not been systematically studied in patients with gastric neuromuscular disorders.
Gastric Electrical Therapies
Gastric Electrical Stimulation
GES at 12 cpm during a 12-month period of continuous treatment significantly decreased nausea and vomiting in patients with refractory, idiopathic, diabetic gastroparesis or postsurgical gastroparesis.287–289 Stimulating the stomach at 12 cpm (four times the normal slow wave frequency) resulted in improvement in nausea and vomiting in patients with gastroparesis.290 No placebo-controlled studies are available to document these benefits of GES. Gastric emptying rates and gastric dysrhythmias did not improve in those patients reporting improvement in symptoms. Vagal afferent nerve stimulation and CNS changes in response to the GES have been proposed as the mechanisms of benefit. Uncontrolled studies from several centers indicate that 50% to 70% of patients treated GES report a decrease in their chronic nausea and vomiting symptoms. Complications include a 10% incidence of infections in the subcutaneous pocket where the device is positioned. There is also a small incidence of fracture of the electrode wires.
Gastric Pacing
Lin and coleagues291 studied low-frequency gastric stimulation using a 3 cpm stimulus to pace or entrain the normal slow wave rhythm in patients with gastroparesis. Gastric pacing seeks to stimulate three gastric peristaltic contractions per minute and improve gastric emptying. Electrodes were positioned on the serosal surface of the stomach during surgery in 13 patients. Stimulation at a frequency up to 10% higher than the normal 3 cpm, at an amplitude of 4mA and a pulse width of 300 milliseconds was used to entrain the slow waves. Gastric dysrhythmias were eradicated in some patients. In a similar study of 9 additional patients, electrical stimulation was used to entrain the slow wave and tachygastria was converted to normal 3 cpm rhythms in 2 patients.292 After one month of gastric pacing treatment, gastric emptying was significantly improved, symptoms of gastroparesis were significantly reduced, and 8 of 9 patients no longer required jejunostomy tube feedings. These pacing devices require more electrical energy compared with GES; thus, battery life is a major limitation at this time. Adverse events due to gastric pacing devices are discomfort at the site of electrical stimulation and fracture or dislodgement of the electrodes.
Sequential Neural Electrical Stimulation
Sequential neural electrical gastric stimulation is gastric pacing that uses a microprocessor to sequentially activate a series of electrodes that encircle the distal two thirds of the stomach. The stimulation sequence induces propagated contractions that cause a forceful emptying of the gastric content.293
ENDOSCOPIC THERAPY
Endoscopic therapies refer to drug or device therapies delivered to the relevant regions of the stomach via endoscopes. The injection of botulinum toxin into the pylorus to decrease sphincter pressure and to improve gastric emptying and nausea and vomiting in patients with gastroparesis produced results similar to placebo injections.293–296 Balloon dilation of the pylorus improves gastric emptying in patients with gastroparesis and normal 3 cpm EGG patterns.213 RFA treatment applied to the lower esophageal sphincter (LES) area in patients with GERD and dyspepsia improve gastric dysrhythmias and emptying.240,241
DIET THERAPY
Dietary Counseling
Many patients with acute or chronic nausea and vomiting from gastric neuromuscular disorders do not know what to eat and may benefit from dietary counseling.297 In the Nausea/Vomiting (Gastroparesis) Diet, liquid and solid foods that are easy for the stomach to mix and empty are prescribed.271 The Nausea/Vomiting Diet is based on gastric emptying principles and is a three-step diet that requires minimal neuromuscular work of the stomach as the diet is advanced (Table 48-5).
Table 48-5 Diet for Nausea and Vomiting in Patients with Gastric Neuromuscular Disorders
DIET | GOAL | AVOID |
---|---|---|
Step 1: Sports Drinks and Bouillon | ||
Modified from Koch KL. Nausea and vomiting. In Wolfe MM, editor. Therapy of Digestive Disorders. 2nd ed. Philadelphia: Elsevier; 2006. pp 1003-17.
Nutraceuticals
Liquid protein meals with or without ginger decrease nausea associated with motion sickness and gastric dysrhythmias, nausea of pregnancy, and delayed nausea after chemotherapy.298–301 These protein-based meal therapies have not been formally evaluated in patients with gastroparesis or gastric dysrhythmias. A rationale for the nutraceutical approach to treatment of nausea in patients with gastric neuromuscular disorders has evolved and deserves further study.
Other Approaches to Nutritional Support
For patients with chronic nausea and vomiting, percutaneous endoscopic gastrostomy (PEG) tubes may be placed for venting gastric contents in order to avoid frequent vomiting episodes and thereby improve quality of life.302 The venting PEG does not treat the underlying gastric neuromuscular disorder, but it allows the patients to empty the stomach rather than suffer repeated episodes of emesis and discomfort. Medications and some nutritional liquids may be tolerated when given through the gastrostomy tube. Jejunal feeding tubes for enteral nutrition may be needed to provide basic caloric support for patients with severe nausea and vomiting from gastric neuromuscular disorders. A PEG with J-tube extension usually fails because a single vomiting episode may propel the extension tube into the stomach. A J-tube placed endoscopically or surgically is required (see Chapters 4 and 5).303 Total parenteral nutrition (TPN) via central intravenous catheters should be avoided if at all possible because of the frequent development of line sepsis and occasional venous thrombosis.
Abell T, McCallum R, Hocking M, et al. Gastric electrical stimulation for medically refractory gastroparesis. Gastroenterology. 2003;125:421-8. (Ref 287.)
Brzana RJ, Koch KL, Bingaman S. Gastric myoelectrical activity in patients with gastric outlet obstruction and idiopathic gastroparesis. Am J Gastroenterol. 1998;93:1803-9. (Ref 149.)
Camilleri M. Integrated upper gastrointestinal response to food intake. Gastroenterology. 2006;131:640-58. (Ref 54.)
De Giorgio R, Sarnelli G, Corinaldesi R, Stranghellini V. Advances in our understanding of the pathology of chronic intestinal pseudo-obstruction. Gut. 2004;53:1549-52. (Ref 245.)
Hinder RA, Kelley KA. Human gastric pacesetter potential: Site of origin, spread, and response to gastric transection and proximal vagotomy. Am J Surg. 1997;133:29-33. (Ref 3.)
Huizinga JD. Physiology and pathophysiology of the interstitial cell of Cajal: From bench to bedside II. Gastric motility: Lessons from mutant mice on slow waves and innervation. Am J Physiol. 2001;281:G1129-34. (Ref 17.)
Ladabaum U, Koshy SS, Woods ML, et al. Differential symptomatic and electrogastrographic effects of distal and proximal human gastric distension. Am J Physiol. 1998;275:G418-24. (Ref 91.)
Murray CD, Martin NM, Patterson M, et al. Ghrelin enhances gastric emptying in diabetic gastroparesis: a double-blind, placebo-controlled, crossover study. Gut. 2005;54:1693-8. (Ref 105.)
Ordog T. Interstitial cells of Cajal in diabetic gastroenteropathy. Neurogastroenterol Motil. 2008;20:8-18. (Ref 164.)
Owyang C, Hasler WL. Physiology and pathophysiology of the interstitial cells of Cajal: From bench to bedside. VI. Pathogenesis and therapeutic approaches to human gastric dysrhythmias. Am J Physiol. 2002;283:G8-15. (Ref 29.)
Sanders KM, Koh SD, Ward SM. Interstitial cells of Cajal as pacemakers in the gastrointestinal tract. Annu Rev Physiol. 2006;68:307-43. (Ref 2.)
Schulze K. Imaging and modeling of digestion in the stomach and the duodenum. Neurogastroenterol Motil. 2006;18:172-83. (Ref 53.)
Stern RM, Jokerst MD, Levine ME, Koch KL. The stomach’s response to unappetizing food: Cephalic-vagal effects on gastric myoelectrical activity. Neurogastroenterol Motil. 2001;13:151-4. (Ref 117.)
Tougas G, Eaker EY, Abell TL, et al. Assessment of gastric emptying using a low fat meal: Establishment of international control values. Am J Gastroenterol. 2000;95:1456-62. (Ref 43.)
Woods SC. Gastrointestinal satiety signals. I. An overview of gastrointestinal signals that influence food intake. Am J Physiol Gastrointest Liver Physiol. 2004;286:G7-13. (Ref 114.)
1. Szurszewski JH. Electrophysiological basis of gastrointestinal motility. In: Johnson LR, editor. Physiology of the gastrointestinal tract. 2nd ed. New York: Raven Press; 1986:383.
2. Sanders KM, Koh SD, Ward SM. Interstitial cells of Cajal as pacemakers in the gastrointestinal tract. Annu Rev Physiol. 2006;68:307-43.
3. Hinder RA, Kelley KA. Human gastric pacesetter potential: Site of origin, spread, and response to gastric transection and proximal vagotomy. Am J Surg. 1997;133:29-33.
4. Koch KL. Electrogastrography. In: Schuster M, Crowel M, Koch KL, editors. Atlas of gastrointestinal motility. Ontario, Canada: BC Decker; 2002:185-201.
5. Bauer AJ, Publicover NG, Sanders KM. Origin and spread of slow waves in canine gastric antral circular muscle. Am J Physiol. 1985;249:G800-6.
6. Coleski R, Hasler WL. Coupling and propagation of normal and dysrhythmic gastric slow waves during acute hyperglycemia in healthy humans. Neurogastroenterol Motil. 2009;21:492-9.
7. Ordog T, Ward SM, Sanders KM. Interstitial cells of Cajal generate electrical slow waves in the murine stomach. J Physiol (Lond). 1999;518:257-69.
8. Sanders KM, Ordog T, Koh SD, Ward SM. A novel pacemaker mechanism drives gastrointestinal rhythmicity. News Physiol Sci. 2000;15:291-8.
9. Kraichely RE, Farrugia G. Mechanosensitive ion channels in interstitial cells of Cajal and smooth muscle of the gastrointestinal tract. Neurogastroenterol Motil. 2007;19:245-52.
10. Lee HT, Hennig GW, Fleming NW, et al. Septal interstitial cells of Cajal conduct pacemaker activity to excite muscle bundles in human jejunum. Gastroenterology. 2007;133:907-17.
11. Cannon WB, Leib CW. The receptive relaxation of the stomach. Am J Physiol. 1911;29:267-73.
12. Distrutti E, Azpiroz F, Soldevilla A, Malagelada JR. Gastric wall tension determines perception of gastric distention. Gastroenterology. 1999;116:1035-42.
13. Sanders KM, Ward SM. Kit mutants and gastrointestinal physiology. J Physiol. 2007;578:33-42.
14. Horowitz B, Ward SM, Sanders KM. Cellular and molecular basis for electrical rhythmicity in gastrointestinal muscles. Annu Rev Physiol. 1999;61:19-43.
15. Young HM, Ciampoli D, Southwell BR, Newgreen DF. Origin of interstitial cells of Cajal in the mouse intestine. Dev Biol. 1996;180:97-107.
16. Komuro T. Structure and organization of interstitial cells of Cajal in the gastrointestinal tract. J Physiol. 2006;576:653-8.
17. Huizinga JD. Physiology and pathophysiology of the interstitial cell of Cajal: From bench to bedside II. Gastric motility: Lessons from mutant mice on slow waves and innervation. Am J Physiol. 2001;281:G1129-34.
18. Sanders KM, Ordog T, Koh SD, Ward SM. Properties of electrical rhythmicity in the stomach. In: Koch KL, Stern RM, editors. Handbook of electrogastrography. New York: Oxford Press; 2004:13-36.
19. Ward SM, Ordog T, Koh SD, et al. Pacemaking in interstitial cells of Cajal depends upon calcium handling by endoplasmic reticulum and mitochondria. J Physiol (Lond). 2000;525:355-61.
20. Sanders KM, Koh SD, Ward SM. Interstitial cells of Cajal as pacemakers in the gastrointestinal tract. Annu Rev Physiol. 2006;68:307-43.
21. Suzuki H, Takano H, Yamamoto Y, et al. Properties of gastric smooth muscles obtained from mice which lack inositol trisphosphate receptor. J Physiol (Lond). 2000;525:105-11.
22. Won KJ, Sanders KM, Ward SM. Interstitial cells of Cajal mediate mechanosensitive responses in the stomach. Proc Natl Acad Sci U S A. 2005;102:14913-18.
23. Sanders KM. Postjunctional electrical mechanisms of enteric neurotransmission. Gut. 2000;47:iv23-5.
24. Dixit D, Zarate N, Liu LW, et al. Interstitial cells of Cajal and adaptive relaxation in the mouse stomach. Am J Physiol Gastrointest Liver Physiol. 2006;291:G1129-36.
25. He CL, Soffer EE, Ferris CD, et al. Loss of interstitial cells of Cajal and inhibitory innervation in insulin-dependent diabetes. Gastroenterology. 2001;121:427-34.
26. Forster J, Damjanov I, Lin Z, et al. Absence of interstitial cells of Cajal in patients with gastroparesis and correlation with clinical findings. J Gastrointest Surg. 2005;9:102-8.
27. Sanders KM, Ordog T, Koh SD, et al. Development of plasticity of interstitial cells of Cajal. Neurogastroenterol Motil. 1999;11:311-38.
28. Long QL, Fang DC, Shi HT, Luo YH. Gastro-electric dysrhythm and lack of gastric interstitial cells of Cajal. World J Gastroenterol. 2004;10:1227-30.
29. Owyang C, Hasler WL. Physiology and pathophysiology of the interstitial cells of Cajal: From bench to bedside. VI. Pathogenesis and therapeutic approaches to human gastric dysrhythmias. Am J Physiol. 2002;283:G8-15.
30. Grundy D, Schemann M. Enteric nervous system. Curr Opin Gastroenterol. 2007;23:121-6.
31. Benarroch EE. Enteric nervous system. Functional organization and neurologic implications. Neurology. 2007;69:1953-7.
32. Gershon MD, Tack J. The serotonin signaling system: From basic understanding to drug development for functional GI disorders. Gastroenterology. 2007;132:397-414.
33. Huizinga JD. Gastrointestinal peristalsis: Joint action of enteric nerves, smooth muscle, and interstitial cells of Cajal. Microsc Res Tech. 1999;47:239-47.
34. Horiguchi K, Sanders KM, Ward SM. Enteric motor neurons form synaptic-like junctions with interstitial cells of Cajal and the canine gastric antrum. Cell Tissue Res. 2000;311:299-313.
35. Ward SM, Sanders KM. Interstitial cells of Cajal: Primary targets of enteric motor innervation. Anat Rec. 2001;262:125-35.
36. Anand P, Aziz Q, Willert R, van Oudenhove L. Peripheral and central mechanisms of visceral sensitization in man. Neurogastroenterol Motil. 2007;19:29-46.
37. Gebhart GF. Pathobiology of visceral pain: Molecular mechanisms and therapeutic implications IV. Visceral afferent contributions to the pathobiology of visceral pain. Am J Physiol Gastrointest Liver Physiol. 2000;278:G834-8.
38. Kumar D, Wingate D, Ruckebusch Y. Circadian variation in the propagation velocity of the migrating motor complex. Gastroenterology. 1986;91:926-30.
39. Kellow JE, Borody TJ, Phillips SF, et al. Human interdigestive motility: Variations in patterns from esophagus to colon. Gastroenterology. 1986;91:386-95.
40. Lacey BE, Koch KL, Crowell MD. The Stomach. Manometry. In: Schuster M, Crowell M, Koch KL, editors. Atlas of gastrointestinal motility. Ontario, Canada: BC Decker; 2002:35-150.
41. Orr WC, Chen CL. Sleep and the gastrointestinal tract. Neurol Clin. 2005;23:1007-24.
42. Vantrappen G, Janssens J, Peeters TL, et al. Motilin and the interdigestive migrating motor complex in man. Dig Dis Sci. 1979;24:497-500.
43. Tougas G, Eaker EY, Abell TL, et al. Assessment of gastric emptying using a low fat meal: Establishment of international control values. Am J Gastroenterol. 2000;95(6):1456-62.
44. Gilija OH, Detmer PR, Jong JM, et al. Intragastric distribution and gastric emptying assessed by three-dimensional ultrasonography. Gastroenterology. 1997;113:38-49.
45. Kuiken SD, Vergeer M, Heisterkamp SH, et al. Role of nitric oxide in gastric motor and sensory functions in healthy subjects. Gut. 2002;51:212-18.
46. Tack J, Demedts I, Meulemans A, et al. Role of nitric oxide in the gastric accommodation reflex and in meal induced satiety in humans. Gut. 2002;51:219-24.
47. Tack J, Piessevaux H, Coulie B, et al. Role of impaired gastric accommodation to a meal in functional dyspepsia. Gastroenterology. 1998;115:1346-52.
48. Lu YX, Owyang C. Duodenal acid-induced gastric relaxation is mediated by multiple pathways. Am J Physiol. 1999;276:G1501-6.
49. Bozkurt A, Oktar BK, Kurtel H, et al. Capsaicin-sensitive vagal fibres and 5-HT3-, gastrin releasing peptide- and cholecystokinin A-receptors are involved in distension-induced inhibition of gastric emptying in the rat. Regul Pept. 1999;83:81-6.
50. Moran TH, Wirth JB, Schwartz GJ, McHugh PR. Interactions between gastric volume and duodenal nutrients in the control of liquid gastric emptying. Am J Physiol. 1999;276:R997-1002.
51. Indireshkumar K, Brasseur JG, Faas H, et al. Relative contributions of “pressure pump” and “peristaltic pump” to gastric emptying. Am J Physiol. 2000;278:G604-16.
52. Savoye-Collet C, Savoye G, Smout A. Determinants of transpyloric fluid transport: A study using combined real-time ultrasound, manometry, and impedance recording. Am J Physiol. 2003;285:G1147-52.
53. Schulze K. Imaging and modeling of digestion in the stomach and the duodenum. Neurogastroenterol Motil. 2006;18:172-83.
54. Camilleri M. Integrated upper gastrointestinal response to food intake. Gastroenterology. 2006;131:640-58.
55. Hasler WL, Coleski R, Chey WD, et al. Differences in intragastric pH in diabetic vs idiopathic gastroparesis: Relation to degree of gastric retention. Am J Physiol Gastrointest Liver Physiol. 2008;294:G1384-91.
56. Camilleri M, Malagelada J-R, Brown ML, et al. Relation between antral motility and gastric emptying of solids and liquids in humans. Am J Physiol. 1985;249:G580-5.
57. Collins PJ, Houghton LA, Read NW, et al. Role of the proximal and distal stomach in mixed solid and liquid meal emptying. Gut. 1991;32:615-19.
58. Gilja OH, Detmer PR, Jong JM, et al. Intragastric distribution and gastric emptying assessed by three-dimensional ultrasonography. Gastroenterology. 1997;113:38-49.
59. Tamas S, Dumitrascu DL, Andreica V, Cotul S. Gastric sequential scintigraphy: Methodology with liquid isotonic meal. Physiologie. 1988;25:47-51.
60. Maurer AH, Parkman HP, Knight LC, Fisher RS. Scintigraphy. In: Schuster M, Crowel M, Koch KL, editors. Atlas of gastrointestinal motility. Ontario, Canada: BC Decker; 2002:171-84.
61. Koch KL, Hong S-P, Xu L. Reproducibility of gastric myoelectrical activity and the water load test in patients with dysmotility-like dyspepsia symptoms and in control subjects. J Clin Gastroenterol. 2000;31:125-9.
62. Raybould HE, Meyer JH, Tabrizi Y, et al. Inhibition of gastric emptying in response to intestinal lipid is dependent on chylomicron formation. Am J Physiol. 1998;274:R1834-8.
63. Carriere F, Barrowman JA, Verger R, Laugier R. Secretion and contribution to lipolysis of gastric and pancreatic lipases during a test meal in humans. Gastroenterology. 1993;105:876-88.
64. McLaughlin J, Luca MG, Jones MN, et al. Fatty acid chain length determines cholecystokinin secretion and effect on gastric motility. Gastroenterology. 1999;116:46-53.
65. Feinle C, D’Amato M, Read NW. Cholecystokinin-A receptors modulate gastric sensory and motor responses to gastric distension and duodenal lipid. Gastroenterology. 1996;110:1379-85.
66. Rao SS, Safadi R, Lu C, Schulze-Delrieu K. Manometric responses of human duodenum during infusion of HCl, hyperosmolar saline, bile, and oleic acid. Neurogastroenterol Motil. 1996;8:35-43.
67. Rao SS, Lu C, Schulze-Delrieu K. Duodenum as an immediate brake to gastric outflow: A video fluoroscopic and manometric assessment. Gastroenterology. 1996;110:740-7.
68. Feinle C, Grundy D, Read NW. Effects of duodenal nutrients on sensory and motor responses of the human stomach to distention. Am J Physiol. 1997;273:G721-6.
69. Imeryuz N, Yegen BC, Bozkurt A, et al. Glucagon-like peptide-1 inhibits gastric emptying via vagal afferent-mediated central mechanisms. Am J Physiol. 1997;273:G920-7.
70. Delgado-Aros S, Kim DY, Burton DD, et al. Effects of GLP-1 on gastric volume, emptying, maximum volume ingested, and postprandial symptoms in humans. Am J Physiol. 2002;282:G424-31.
71. Strader AD, Woods SC. Gastrointestinal hormones and food intake. Gastroenterology. 2005;128:175-91.
72. Rayner CK, Samsom M, Jones KL, Horowitz M. Relationships of upper gastrointestinal motor and sensory function with glycemic control. Diabetes Care. 2001;24:371-81.
73. Hasler WL, Soudah HC, Dulai G, Owyang C. Mediation of hyperglycemia-evoked gastric slow-wave dysrhythmias by endogenous prostaglandins. Gastroenterology. 1995;108:726-36.
74. Coleski R, Hasler WL. Directed endoscopic mucosal mapping of normal and dysrhythmic gastric slow waves in healthy subjects. Neurogastroenterol Motil. 2004;16:557-65.
75. Rayner CK, Verhagen MA, Hebbard GG, et al. Proximal gastric compliance and perception of distension in type 1 diabetes mellitus: Effects of hyperglycemia. Am J Gastroenterol. 2000;95:1175-83.
76. Shi M, Jones AR, Niedringhaus MS, et al. Glucose acts in the CNS to regulate gastric motility during hypoglycemia. Am J Physiol Regul Integr Comp Physiol. 2003;285:R1192-1202.
77. Lin HC, Kim BH, Elashoff JD, et al. Gastric emptying of solid food is most potently inhibited by carbohydrate in the canine distal ileum. Gastroenterology. 1992;102:793-801.
78. Beglinger C, Degen L. Role of thyrotropin releasing hormone and corticotrophin releasing factor in stress related alterations of gastrointestinal motor function. Gut. 2002;51:i45-9.
79. Muth ER, Stern RM, Koch KL. Effects of vection-induced motion sickness on gastric myoelectric activity and oral-cecal transit time. Dig Dis Sci. 1996;41:330-4.
80. Xu LH, Koch KL, Summy-Long J, et al. Hypothalamic and gastric myoelectrical responses during vection-induced nausea in healthy Chinese subject. Am J Physiol. 1993;265:E578-84.
81. Koch KL, Summy-Long J, Bingaman S, et al. Vasopressin and oxytocin responses to illusory self-motion and nausea in man. J Clin Endocrinol Metab. 1990;71:1269-75.
82. Bennink R, Peeters M, Van Den Maegdenbergh V, et al. Comparison of total and compartmental gastric emptying and antral motility between healthy men and women. Eur J Nucl Med. 1998;25:1293-9.
83. Kuo B, McCallum RW, Koch KL, et al. Comparison of gastric emptying of a non-digestible capsule to a radio-labeled meal in healthy and gastroparetic subjects. Aliment Pharmacol Ther. 2008;27:186-96.
84. Caballero-Plasencia AM, Valenzuela-Barranco M, Martin-Ruiz JL, et al. Are there changes in gastric emptying during the menstrual cycle? Scand J Gastroenterol. 1999;34:772-6.
85. Costa M, Brookes SJ, Hennig GW. Anatomy and physiology of the enteric nervous system. Gut. 2000;47(Supple IV):iv15-iv19.
86. Gebhart GF. Visceral pain-peripheral sensitisation. Gut. 2000;47:iv54-5.
87. Powley TL. Vagal input to the enteric nervous system. Gut. 2000;47:iv30-2.
88. Lu YX, Owyang C. Duodenal acid-induced gastric relaxation is mediated by multiple pathways. Am J Physiol. 1999;276:G1501-6.
89. Stern RM, Koch KL, Stewart WR, Lindblad IM. Spectral analysis of tachygastria recorded during motion sickness. Gastroenterology. 1987;92:92-7.
90. Koch KL, Stern RM, Vasey MJ, et al. Neuroendocrine and gastric myoelectrical responses to illusory self-motion in humans. Am J Physiol. 1990;258:E304-10.
91. Ladabaum U, Koshy SS, Woods ML, et al. Differential symptomatic and electrogastrographic effects of distal and proximal human gastric distension. Am J Physiol. 1998;275:G418-24.
92. Rolls B, Barnett RA, editors. Volumetrics. Feel full on fewer calories. New York: HarperCollins, 2000.
93. Rolls BJ, Castellanos VH, Halford JC, et al. Volume of food consumed affects satiety in men. Am J Clin Nutr. 1998;67:1170-7.
94. Delgado-Aros S, Camilleri M, Castillo EJ, et al. Effect of gastric volume or emptying on meal-related symptoms after liquid nutrients in obesity: A pharmacologic study. Clin Gastroenterol Hepatol. 2005;3:997-1006.
95. Cecil JE, Francis J, Read NW. Relative contributions of intestinal, gastric, oro-sensory influences and information to changes in appetite induced by the same liquid meal. Appetite. 1998;31:377-90.
96. Distrutti F, Azpiroz F, Soldevilla A, Malagelada JR. Gastric wall tension determines perception of gastric distension. Gastroenterology. 1999;116:1035-42.
97. Tack J, Caenepeel P, Piessevaux H, et al. Assessment of meal induced gastric accommodation by a satiety drinking test in health and in severe functional dyspepsia. Gut. 2003;52:1271-7.
98. Lee KJ, Vos R, Janssens J, Tack J. Influence of duodenal acidification on the sensorimotor function of the proximal stomach in humans. Am J Physiol. 2004;286:G278-84.
99. Ladabaum U, Brown MB, Pan W, Tack J. Effects of nutrients and serotonin 5-HT3 antagonism on symptoms evoked by distal gastric distension in humans. Am J Physiol. 2001;280:G201-8.
100. Kojima M, Kangawa K. Grehlin: Structure and function. Physiol Rev. 2005;85:495-522.
101. Date Y, Murakami N, Toshinai K, et al. The role of the gastric afferent vagal nerve in ghrelin-induced feeding and growth hormone secretion in rats. Gastroenterology. 2002;123:1120-8.
102. Krowicki ZK, Burmeister MA, Berthoud HR, et al. Orexins in rat dorsal motor nucleus of the vagus potently stimulate gastric motor function. Am J Physiol. 2002;283:G465-72.
103. Tschop M, Wawarta R, Riepl RI, et al. Post-prandial decrease of circulating human ghrelin levels. J Endocrinol Invest. 2001;24:RC19-21.
104. Cummings DE, Weigle DS, Frayo RS, et al. Plasma ghrelin levels after diet-induced weight loss or gastric bypass surgery. N Engl J Med. 2002;346:1623-30.
105. Murray CD, Martin NM, Patterson M, et al. Ghrelin enhances gastric emptying in diabetic gastroparesis: A double-blind, placebo-controlled, crossover study. Gut. 2005;54:1693-8.
106. Tack J, Depoortere I, Bisschops R, et al. Influence of ghrelin on gastric emptying and meal-related symptoms in idiopathic gastroparesis. Aliment Pharmacol Ther. 2005;22:847-53.
107. Beglinger C, Degen L. Fat in the intestine as a regulator of appetite—role of CCK. Physiol Behav. 2004;83:617-21.
108. Moran TH, Ladenheim EE, Schwartz GJ. Within-meal gut feedback signaling. Int J Obes Relat Metab Disord. 2001;25:S39-41.
109. Pico C, Oliver P, Sanchez J, Palou A. Gastric leptin: A putative role in the short-term regulation of food intake. Br J Nutr. 2003;90:735-41.
110. Delgado-Aros S, Vella A, Camilleri M, et al. Effects of glucagon-like peptide-1 and feeding on gastric volumes in diabetes mellitus with cardio-vagal dysfunction. Neurogastroenterol Motil. 2003;15:435-43.
111. Glatzie J, Darcel N, Rechs AJ, et al. Apolipoprotein A-IV stimulates duodenal vagal afferent activity to inhibit gastric motility via a CCK1 pathway. Am J Physiol. 2004;287:R354-9.
112. Cuche G, Cuber JC, Malbert CH. Ileal short-chain fatty acids inhibit gastric motility by a humoral pathway. Am J Physiol. 2000;279:G925-30.
113. Degan L, Oesch S, Casanova M, et al. Effect of peptide YY3-36 on food intake in humans. Gastroenterology. 2005;129:1430-6.
114. Woods SC. Gastrointestinal satiety signals. I. An overview of gastrointestinal signals that influence food intake. Am J Physiol Gastrointest Liver Physiol. 2004;286:G7-13.
115. Stern RM, Crawford HE, Stewart W, et al. Sham feeding. Cephalic-vagal influences on gastric myoelectric activity. Dig Dis Sci. 1989;34:521-7.
116. Feldman M, Richardson CT. Role of thought, sight, smell, and taste of food in the cephalic phase of gastric acid secretion in humans. Gastroenterology. 1986;90:428-33.
117. Stern RM, Jokerst MD, Levine ME, Koch KL. The stomach’s response to unappetizing food: Cephalic-vagal effects on gastric myoelectrical activity. Neurogastroenterol Motil. 2001;13:151-4.
118. Sase M, Tamura H, Ueda K, Kato H. Sonographic evaluation of antepartum development of fetal gastric motility. Ultrasound Obstet Gynecol. 1999;13:323-6.
119. Hassan BB, Butler R, Davidson GP, et al. Patterns of antropyloric motility in fed healthy preterm infants. Arch Dis Child Fetal Neonatal Ed. 2002;87:F95-9.
120. Koch KL, Tran T, Bingaman S, Sperry N. Gastric myoelectrical activity in fasted and fed premature and term infants. J Gastrointestinal Motility. 1993;5:41-7.
121. Patterson M, Rintala R, Lloyd DA. A longitudinal study of electrogastrography in normal neonates. J Pediatr Surg. 2000;35:59-61.
122. Cheng W, Tam PK. Gastric electrical activity normalises in the first decade of life. Eur J Pediatr Surg. 2000;10:295-9.
123. Sanders KM, Ordog T, Ward SM. Physiology and pathophysiology of the interstitial cells of Cajal: From bench to bedside. IV. Genetic animal models of GI motility disorders caused by loss of interstitial cells of Cajal. Am J Physiol. 2002;282:G747-56.
124. Huizinga JD, Berezin I, Sircar K, et al. Development of interstitial cells of Cajal in a full-term infant without an enteric nervous system. Gastroenterology. 2001;120:561-7.
125. Vanderwinden JM, Liu H, De Latet MH, Vanderhaeghen JJ. Study of the interstitial cells of Cajal in infantile hyperthrophic pyloric stenosis. Gastroenterology. 1996;111:279-88.
126. Guo JP, Maurer AH, Fisher RS, Parkman HP. Extending gastric emptying scintigraphy from two to four hours detects more patients with gastroparesis. Dig Dis Sci. 2001;46:24-9.
127. Ziessman HA, Okolo PI, Mullin G, Chander A. Liquid gastric emptying is often abnormal when solid emptying is normal. J Clin Gastroenterol. 2009;43:639-43.
128. Abell TL, Camilleri M, Donohoe K, et al. Consensus recommendations for gastric emptying scintigraphy: A joint report of the American Neurogastroenterology and Motility Society and the Society of Nuclear Medicine. Am J Gastroenterol. 2008;103:753-63.
129. Viramontes BE, Kim DY, Camilleri M, et al. Validation of a stable isotope gastric emptying test for normal, accelerated or delayed gastric emptying. Neurogastroenterol Motil. 2001;13:567-74.
130. Bromer MQ, Kantor SB, Wagner VA, et al. Simultaneous measurement of gastric emptying with simple muffin meal using (13C) octanoate breath test and scintigraphy in normal subjects and patients with dyspeptic symptoms. Dig Dis Sci. 2002;47:1657-63.
131. Szarka LA, Camilleri M, Vella A, et al. A stable isotope breath test with a standard meal for abnormal gastric emptying of solids in the clinic and in research. Clin Gastroenterol Hepatol. 2008;6:635-43.
132. Darwiche G, Bjorgell O, Thorsson O, Almer LO. Correlation between simultaneous scintigraphic and ultrasonography: Measurement of gastric emptying in patients with type 1 diabetes mellitus. J Ultrasound Med. 2003;22:459-66.
133. Hausken T, Odegaard S, Berstad A. Antroduodenal motility studied by real-time ultrasonography. Effect of enprostil. Gastroenterology. 1991;100:59-63.
134. Marciani L, Gowland PA, Spiller RC, et al. Effect of meal viscosity and nutrients on satiety, intragastric dilution and emptying assessed by MRI. Am J Physiol. 2001;280:G1227-33.
135. Hausken T, Mundt M, Samsom M. Low antroduodenal pressure gradients are responsible for gastric emptying of a low-caloric liquid meal in humans. Neurogastroenterol Motil. 2002;14:97-105.
136. Cassilly D, Kantor S, Knight LC, et al. Gastric emptying of a non-digestible solid: Assessment with simultaneous SmartPill pH and pressure capsule, antroduodenal manometry, gastric emptying scintigraphy. Neurogastroenterol Motil. 2008;20:311-19.
137. Kuo B, McCallum RW, Koch KL, et al. Comparison of gastric emptying of a nondigestible capsule to a radio-labelled meal in healthy and gastroparetic subjects. Aliment Pharmacol Ther. 2008;27:186-96.
138. Smout AJ, van der Schee EJ, Grashuis JL. What is measured in electrogastrography? Dig Dis Sci. 1980;25:179-87.
139. Koch KL. Electrogastrography. In: Schuster M, Crowell M, Koch KL, editors. Atlas of Gastrointestinal Motility. Ontario, Canada: BC Decker; 2002:185-201.
140. Koch KL. Physiological basis of electrogastrography. In: Koch KL, Stern RM, editors. Handbook of electrogastrography. New York: Oxford Press; 2004:37-67.
141. Lin C, Chen JD, Schirmer BD, McCallum RW. Postprandial response of gastric slow waves: Correlation of serosal recordings with the electrogastrogram. Dig Dis Sci. 2000;45:645-51.
142. Verhagen MA, Van Schelven LJ, Samsom M, Smout AJ. Pitfalls in the analysis of electrogastrographic recordings. Gastroenterology. 1999;117:453-60.
143. Zhu H, Chen JDZ. Gastric distention alters frequency and regularity but not amplitude of the gastric slow wave. Neurogastroenterol Motil. 2004;16:745-52.
144. Levanon D, Zhang M, Orr WC, Chen JD. Effects of meal volume and composition on gastric myoelectrical activity. Am J Physiol. 1998;274:G430-4.
145. Koch KL, Stern RM, Vasey M, et al. Gastric dysrhythmias and nausea of pregnancy. Dig Dis Sci. 1990;35:961-8.
146. Koch KL, Frissora C. Nausea and vomiting in pregnancy. Gastroenterol Clin North Am. 2003;32:201-34.
147. Parkman HP, Miller MA, Trate D, et al. Electrogastrography and gastric emptying scintigraphy are complementary for assessment of dyspepsia. J Clin Gastroenterol. 1997;24:214-19.
148. Koch KL, Stern RM, Stewart WR, Vasey MW. Gastric emptying and gastric myoelectrical activity in patients with symptomatic diabetic gastroparesis: Effects of long-term domperidone treatment. Am J Gastroenterol. 1989;84:1069-75.
149. Brzana RJ, Koch KL, Bingaman S. Gastric myoelectrical activity in patients with gastric outlet obstruction and idiopathic gastroparesis. Am J Gastroenterol. 1998;93:1803-9.
150. Chen JD, Lin Z, Pan J, McCallum RW. Abnormal gastric myoelectrical activity and delayed gastric emptying in patients with symptoms suggestive of gastroparesis. Dig Dis Sci. 1996;41:1538-45.
151. Parkman HP, Hasler WL, Barnett JL, et al. Electrogastrography: A document prepared by the gastric section of the American Motility Society Clinical GI Motility Testing Task Force. Neurogastroenterol Motil. 2003;15:89-102.
152. Abell TL, Malagelada JR. Glucagon-evoked gastric dysrhythmias in humans shown by an improved electrogastrographic technique. Gastroenterology. 1985;88:1932-40.
153. De Schepper HU, Cremonini F, Chitkara D, Camilleri M. Assessment of gastric accommodation: Overview and evaluation of current methods. Neurogastroenterol Motil. 2004;16:275-85.
154. Mundt MW, Hausken T, Samsom M. Effect of intragastric barostat bag on proximal and distal gastric accommodation in response to liquid meal. Am J Physiol. 2002;283:G681-6.
155. Tack J, Piessevaux H, Coulie B, et al. Role of impaired gastric accommodation to a meal in functional dyspepsia. Gastroenterology. 1998;115:1346-52.
156. Sarnelli G, Caenepeel P, Geypens B, et al. Symptoms associated with impaired gastric emptying of solids and liquids in functional dyspepsia. Am J Gastroenterol. 2003;98:783-8.
157. Caldarella MP, Azpiroz F, Malagelada JR. Antro-fundic dysfunctions in functional dyspepsia. Gastroenterology. 2003;124:1220-9.
158. Bennink RJ, van den Elzen BD, Kuiken SD, Boeckxstaens GE. Noninvasive measurement of gastric accommodation by means of pertechnetate SPECT: Limiting radiation dose without losing image quality. J Nucl Med. 2004;45:147-52.
159. Bouras EP, Delgado-Aros S, Camilleri M, et al. SPECT imaging of the stomach: Comparison with barostat, and effects of sex, age, body mass index, and fundoplication. Gut. 2002;51:781-6.
160. Jones MP, Hoffman S, Shah D, et al. The water load test: Observations from healthy controls and patients with functional dyspepsia. Am J Physiol. 2003;284:G896-904.
161. Talley NJ, Camileri M, Burton D, et al. Double-blind, randomized, placebo-controlled study to evaluate the effects of tegaserod on gastric motor, sensory and myoelectrical function in healthy volunteers. Aliment Pharmacol Ther. 2006;24:859-67.
162. Rajan E, Gostout CJ, Lurken MS, et al. Endoscopic “no hole” full-thickness biopsy of the stomach to detect myenteric ganglia. Gastrointest Endosc. 2008;68:301-7.
163. Iwasaki H, Kajimura M, Osawa S, et al. A deficiency of gastric interstitial cells of Cajal accompanied by decreased expression of neuronal nitric oxide synthase and substance P in patients with type 2 diabetes mellitus. J Gastroenterol. 2006;41:1076-87.
164. Ordog T. Interstitial cells of Cajal in diabetic gastroenteropathy. Neurogastroenterol Motil. 2008;20:8-18.
165. Zarate N, Mearin F, Wang XY, et al. Severe idiopathic gastroparesis due to neuronal and interstitial cells of Cajal degeneration: Pathological findings and management. Gut. 2003;52:966-70.
166. De Giorgio R, Guerrini S, Barbara G, et al. Inflammatory neuropathies of the enteric nervous system. Gastroenterology. 2004;126:1872-83.
167. Pardi DS, Miller SM, Miller DL, et al. Paraneoplastic dysmotility: Loss of interstitial cells of Cajal. Am J Gastroenterol. 2002;97:1828-33.
168. Moskovitz DN, Robb KV. Small cell lung cancer with positive antiHu antibodies presenting as gastroparesis. Can J Gastroenterol. 2002;16:171-4.
169. Pande H, Lacy BE, Crowell MD. Inflammatory causes of gastroparesis: Report of five cases. Dig Dis Sci. 2002;47:2664-8.
170. Pasricha PJ, Pehlivanov ND, Gomez G, et al. Changes in the gastric enteric nervous system and muscle: A case report on two patients with diabetic gastroparesis. [abstract]. BMC Gastroenterology, 8, 2008:21
171. Vittal H, Farrugia G, Gomez G, Pasricha PJ. Mechanisms of disease: The pathological basis of gastroparesis—A review of experimental clinical studies. Gastroenterol Hepatol. 2007;4:336-46.
172. Koch KL. Unexplained nausea and vomiting. Curr Treatment Options Gastroenterol. 2000;3:303-13.
173. Camilleri M, Talley NJ. Pathophysiology as a basis for understanding symptom complexes and therapeutic targets. Neurogastroenterol Motil. 2004;16:135-42.
174. Parkman HP, Haster WL, Fisher RS. American Gastroenterological Association medical position statement: Diagnosis and treatment of gastroparesis. Gastroenterology. 2004;127:1589-91.
175. Horowitz M, O’Donovan D, Jones KL, et al. Gastric emptying in diabetes: Clinical significance and treatment. Diabet Med. 2002;19:177-94.
176. Koch KL. Diabetic gastropathy: Gastric neuromuscular dysfunction in diabetes mellitus. A review of symptoms, pathophysiology, and treatment. Dig Dis Sci. 1999;44:1061-75.
177. Jebbink RJA, Samsom M, Bruijs PP, et al. Hyperglycemia induces abnormalities of gastric myoelectrical activity in patients with type 1 diabetes mellitus. Gastroenterology. 1994;107:1390-7.
178. Hasler WL, Soudah HC, Dulai G, Owyang C. Mediation of hyperglycemia-evoked gastric slow-wave dysrhythmias by endogenous prostaglandins. Gastroenterology. 1995;108:727-36.
179. Schvarcz E, Plamar M, Aman J, et al. Physiological hyperglycemia slows gastric emptying in normal subjects and patients with insulin-dependent diabetes mellitus. Gastroenterology. 1997;113:60-6.
180. Malagelada JR, Rees WD, Mazzotta LJ, Go VL. Gastric motor abnormalities in diabetic and postvagotomy gastroparesis: Effect on metoclopramide and bethanechol. Gastroenterology. 1980;78:286-93.
181. Fraser R, Horowitz M, Dent J. Hyperglycemia stimulates pyloric motility in normal subjects. Gut. 1991;32:475-8.
182. Rayner CK, Su YC, Doran SM, et al. The stimulation of antral motility by erythromycin is attenuated by hyperglycemia. Am J Gastroenterol. 2000;95:2233-41.
183. Andrews JM, Rayner CK, Doran S, et al. Physiological changes in blood glucose affect appetite and pyloric motility during intraduodenal lipid infusion. Am J Physiol. 1998;275:G797-804.
184. Lingenfelser T, Sun W, Hebbard GS, et al. Effects of duodenal distension on antropyloroduodenal pressures and perception are modified by hyperglycemia. Am J Physiol. 1999;276:G711-18.
185. Troncon LE, Rosa-Silva L, Oliveira RB, et al. Abnormal intragastric distribution of a liquid nutrient meal in patients with diabetes mellitus. Dig Dis Sci. 1998;43:1421-9.
186. Samson M, Roelofs JM, Akkermans LM, et al. Proximal gastric motor activity in response to a liquid meal in type 1 diabetes with autonomic neuropathy. Dig Dis Sci. 1998;43:491-6.
187. Soykan I, Lin Z, Sarosiek I, McCallum RW. Gastric myoelectrical activity, gastric emptying, and correlations with symptoms and fasting blood glucose levels in diabetic patients. Am J Med Sci. 1999;317:226-31.
188. Takahashi T, Kojima Y, Tsunoda Y, et al. Impaired intracellular signal transduction in gastric smooth muscle of diabetic BB/W rats. Am J Physiol. 1996;270:G411-17.
189. Horvath VJ, Vittal H, Lorincz A, et al. Reduced stem cell factor links smooth myopathy and loss of interstitial cells of cajal in murine diabetic gastroparesis. Gastroenterology. 2006;130:759-70.
190. Mearin F, Camilleri M, Malagelada JR. Pyloric dysfunction in diabetics with recurrent nausea and vomiting. Gastroenterology. 1986;90:1919-25.
191. Intagliata N, Koch KL. Gastroparesis in type 2 diabetes mellitus: Prevalence, etiology, diagnosis and treatment. Curr Gastroenterol Reps. 2007;9:270-9.
192. CDC. National Diabetes Fact Sheet. www.cdc.gov/diabetes/pubs/estimates05.htm#top. Accessed February 6, 2007
193. Schreibman I, Xu L, Carusone S, et al. Water load test unmasks nausea, bloating and tachygastria in patients with type 2 diabetes mellitus. [abstract]. Neurogastroenterol Motil, 13, 2001:274
194. Bertin E, Schneider N, Abdelli N, et al. Gastric emptying is accelerated in obese type 2 diabetic patients without autonomic neuropathy. Diabetes Metab. 2001;27:357-64.
195. Watkins CC, Sawa A, Jeffrey S, et al. Insulin restores neuronal nitric oxide synthase expression and function that is lost in diabetic gastropathy. J Clin Invest. 2000;106:373-84.
196. James AN, Ryan JP, Crowell MD, Parkman HP. Regional gastric contractility alterations in a diabetic gastroparesis mouse model: Effects of cholinergic and serotoninergic stimulation. Am J Physiol. 2004;287:G612-19.
197. Ordog T, Takayama I, Cheung WK, et al. Remodeling of networks of interstitial cells of Cajal in a murine model of diabetic gastroparesis. Diabetes. 2000;49:1731-9.
198. Cowley DJ, Vernon P, Jones T, et al. Gastric emptying of solid meals after truncal vagotomy and pyloroplasty in human subjects. Gut. 1972;13:176-81.
199. Akkermans LM, Hendrikse CA. Post-gastrectomy problems. Dig Liver Dis. 2000;32:S263-4.
200. Sun WM, Doran SM, Jones KL, et al. Long-term effects of pyloromyotomy on pyloric motility and gastric emptying in humans. Am J Gastroenterol. 2000;95:92-100.
201. Fich A, Neri M, Camilleri M, et al. Stasis syndromes following gastric surgery: Clinical and motility features of 60 symptomatic patients. J Clin Gastroenterol. 1990;12:505-12.
202. Eagon JC, Miedema BW, Kelly KA. Postgastrectomy syndromes. Surg Clin North Am. 1992;72:445-65.
203. Le Blanc-Louvry I, Savoye G, Maillot C, et al. An impaired accommodation of the proximal stomach to a meal is associated with symptoms after distal gastrectomy. Am J Gastroenterol. 2003;98:2642-7.
204. Mathias JR, Fernandez A, Sninsky CA, et al. Nausea, vomiting and abdominal pain after Roux-en-Y anastomosis: Motility of the jejunal limb. Gastroenterology. 1985;88:101-7.
205. Vantrappen G, Coremans G, Janssens J, et al. Inversion of the slow wave frequency gradient in symptomatic patients with Roux-en-Y anastomosis. Gastroenterology. 1991;101:1282-8.
206. Lundell LR, Myers JC, Jamieson GG. Delayed gastric emptying and its relationship to symptoms of “gas bloat” after antireflux surgery. Eur J Surg. 1994;160:161-6.
207. Vu MK, Ringers J, Arndt JW, et al. Prospective study of the effect of laparoscopic hemifundoplication on motor and sensory function of the proximal stomach. Br J Surg. 2000;87:338-43.
208. Scheffer RC, Tatum RP, Shi G, et al. Reduced tLESR elicitation in response to gastric distention in fundoplication patients. Am J Physiol. 2003;284:G815-20.
209. Lindeboom MY, Vu MK, Ringers J, et al. Function of the proximal stomach after partial versus complete laparoscopic fundoplication. Am J Gastroenterol. 2003;98:284-90.
210. Farrell TM, Richardson WS, Halkar R, et al. Nissen fundoplication improves gastric motility in patients with delayed gastric emptying. Surg Endosc. 2001;15:271-4.
211. Liberski SM, Koch KL, Atnip RG, Stern RM. Ischemic gastroparesis: Resolution after revascularization. Gastroenterology. 1990;99:252-7.
212. Balaban DH, Chen J, Lin Z, et al. Median arcuate ligament syndrome: A possible cause of idiopathic gastroparesis. Am J Gastroenterol. 1997;92:519-23.
213. Noar MD, Lihua XU, Koch KL. Effect of radiofrequency ablation on gastric dysrhythmias and gastric emptying in patients with gastroesophageal reflux disease (GERD) and functional dyspepsia. [abstract]. Gastroenterology. 2003;124:A98
214. Soykan I, Sivri B, Saroseik I, et al. Demography, clinical characteristics, psychological and abuse profiles, treatment, and long-term follow-up of patients with gastroparesis. Dig Dis Sci. 1998;43:2398-404.
215. Oh JJ, Kim CH. Gastroparesis after a presumed viral illness: Clinical and laboratory features and natural history. Mayo Clin Proc. 1990;65:636-42.
216. Bityutskiy LP, Soykan I, McCallum RW. Viral gastroparesis: A subgroup of idiopathic gastroparesis—clinical observations and long-term outcomes. Am J Gastroenterol. 1997;92:1501-4.
217. Vassalo M, Camilleri M, Caron BL, Low PA. Gastrointestinal motor dysfunction in acquired selective cholinergic dysautonomia associated with infectious mononucleosis. Gastroenterology. 1991;100:252-8.
218. Jain D, Moussa K, Tandon M, et al. The role of interstitial cells of Cajal in motility disorders of the bowel. Am J Gastroenterol. 2003;98:618-24.
219. Ordog T, Baldo M, Danko R, Sanders KM. Plasticity of electrical pacemaking by interstitial cells of Cajal and gastric dysrhythmias in W/W/mutant mice. Gastroenterology. 2002;123:2028-40.
220. Tack J, Talley NJ, Camilleri M, et al. Functional gastroduodenal disorders. Gastroenterology. 2006;130:1466-79.
221. Bersherdas K, Leahy A, Mason I, et al. The effect of cisapride on dyspepsia symptoms and the electrogastrogram in patients with non-ulcer dyspepsia. Aliment Pharmacol Ther. 1998;12:755-9.
222. Chang CS, Lien HC, Yeh HZ, et al. Effect of cisapride on gastric dysrhythmia and emptying of indigestible solids in type-II diabetic patients. Scand J Gastroenterol. 1998;33:600-4.
223. Cucchiara S, Minella R, Riezzo G, et al. Reversal of gastric electrical dysrhythmias by cisapride in children with functional dyspepsia: Report of three cases. Dig Dis Sci. 1992;37:1136-40.
224. Rothstein RD, Alavai A, Reynolds JC. Electrogastrography in patients with gastroparesis and effect of long-term cisapride. Dig Dis Sci. 1993;38:1518-24.
225. Tack J, Caenepeel P, Fischler B, et al. Symptoms associated with hypersensitivity to gastric distension in functional dyspepsia. Gastroenterology. 2001;121:526-35.
226. Tack J, Caenepeel P, Piessevaux H, et al. Assessment of meal induced gastric accommodation by a satiety drinking test in health and in severe functional dyspepsia. Gut. 2003;52:1271-7.
227. Kim DY, Delgado-Aros S, Camilleri M, et al. Noninvasive measurement of gastric accommodation in patients with idiopathic nonulcer dyspepsia. Am J Gastroenterol. 2001;96:3099-105.
228. Boeckxstaens GE, Hirsch DP, van den Elzen BD, et al. Impaired drinking capacity in patients with functional dyspepsia: Relationship with proximal stomach function. Gastroenterology. 2001;121:1054-63.
229. Fischler B, Tack J, De Gucht V, et al. Heterogeneity of symptom pattern, psychological factors, and pathophysiological mechanisms in severe functional dyspepsia. Gastroenterology. 2003;124:903-10.
230. Muth ER, Koch KL, Stern RM. Significance of autonomic nervous system activity in functional dyspepsia. Dig Dis Sci. 2000;45:854-63.
231. Koch KL, Stern RM. Functional disorders of the stomach. Semin Gastrointest Dis. 1996;7:185-95.
232. Samsom M, Verhagen MA, vanBerge Henegouwen GP, Smout AJ. Abnormal clearance of exogenous acid and increased acid sensitivity of the proximal duodenum in dyspeptic patients. Gastroenterology. 1996;116:515-20.
233. Tack J, Talley NJ, Camilleri M, et al. Functional gastrointestinal disorders. In: Drossman DA, editor. The Rome III functional gastrointestinal disorders. Lawrence, Kansas: Allen Press; 2006:419-86.
234. McCallum RW, Berkowitz DM, Lerner E. Gastric emptying in patients with gastroesophageal reflux. Gastroenterology. 1981;80:285-91.
235. Tack J, Caenepeel P, Arts J, et al. Prevalence of acid reflux in functional dyspepsia and its association with symptom profile. Gut. 2005;54:1370-6.
236. Koch KL, Xu L, Noar M. Gastric myoelectrical and emptying activity in patients with gastroesophageal reflux disease (GERD) and dysmotility-like functional dyspepsia (GERD+): Effect of water load test. [abstract]. Am J Gastroenterol. 2001;96:526.
237. Quigley EM. Gastroesophageal reflux disease: The roles of motility in pathophysiology and therapy. Am J Gastroenterol. 1993;88:1649-51.
238. Zerbib F, des Varannes SB, Ropert A, et al. Proximal gastric tone in gastro-esophageal reflux disease. Eur J Gastroenterol Hepatol. 1999;11:511-15.
239. Penagini R, Hebbard G, Horowitz M, et al. Motor function of the proximal stomach and visceral perception in gastro-esophageal reflux disease. Gut. 1998;42:251-7.
240. Noar MK, Koch KL. Effect of radiofrequency ablation on gastric dysrhythmias and gastric emptying in patients with gastroesophageal reflux disease (GERD) and functional dyspepsia. [abstract]. Gastroenterology. 2003;124:A98.
241. Noar MD, Noar E. Gastroparesis associated with gastroesophageal reflux disease and corresponding reflux symptoms may be corrected by radiofrequency ablation of the cardia and esophagogastric junction. Surg Endosc. 2008;22:2440-4.
242. Koch KL, Bingaman S, Muth ER, Ouyang A. Effects of physiological gastric distention on nausea, stomach fullness, satiety and gastric myoelectrical activity in patients with irritable bowel syndrome. [abstract]. Gastroenterology. 1997;112:A763.
243. Penning C, Vu MK, Delmarre JB, Masclee AA. Proximal gastric motor and sensory function in slow transit constipation. Scand J Gastroenterol. 2001;36:1267-73.
244. Stranghellini V, Cogliandro RF, de Giorgio R, et al. Chronic intestinal pseudo-obstruction: Manifestations, natural history and management. Neurogastroenterol Motil. 2007;19:440-52.
245. De Giorgio R, Sarnelli G, Corinaldesi R, Stranghellini V. Advances in our understanding of the pathology of chronic intestinal pseudo-obstruction. Gut. 2004;53:1549-52.
246. Marie L, Levesque H, Ducrotte P, et al. Gastric involvement in systemic sclerosis: A prospective study. Am J Gastroenterol. 2001;96:77-83.
247. Verne GN, Soldevia-Pico C, Robinson ME, et al. Autonomic dysfunction and gastroparesis in cirrhosis. J Clin Gastroenterol. 2004;38:72-6.
248. Van Vlem B, Schoonjans R, Vanholder R, et al. Delayed gastric emptying in dyspeptic chronic hemodialysis patients. Am J Kidney Dis. 2000;36:962-8.
249. Chowdhury RS, Forsmark CE, Davis RH, et al. Prevalence of gastroparesis in patients with small duct chronic pancreatitis. Pancreas. 2003;26:235-8.
250. Neild PJ, Nijran KS, Yazaki E, et al. Delayed gastric emptying in human immunodeficiency virus infection: Correlation with symptoms, autonomic function and intestinal motility. Dig Dis Sci. 2000;45:1491-9.
251. Fortunato JE, Darbari A, Cuffari C, et al. Esophageal motility dysfunction in children with Rett syndrome, gastroesophageal reflux and dysphagia. J Appl Res. 2008;8:84-94.
252. DiBaise JK, Quigley EM. Tumor-related dysmotility: Gastrointestinal dysmotility syndromes associated with tumors. Dig Dis Sci. 1998;43:1369-401.
253. Lee HR, Lennon VA, Camilleri M, Prather CM. Perineoplastic gastrointestinal motor dysfunction: Clinical and laboratory characteristics. Am J Gastroenterol. 2001;96:373-9.
254. Lin Z, Chen JD, Parolisi S, et al. Prevalence of gastric myoelectrical abnormalities in patients with nonulcer dyspepsia and H. pylori infection: Resolution after H. pylori eradication. Dig Dis Sci. 2001;46:739-45.
255. Abell TL, Malagelada JR, Lucas AR, et al. Gastric electromechanical and neurohormonal function in anorexia nervosa. Gastroenterology. 1987;93:958-65.
256. Koch KL, Bingaman S, Tan L, Stern RM. Visceral perceptions and gastric myoelectrical activity in healthy women and in patients with bulimia nervosa. Neurogastroenterol Motil. 1998;10:3-10.
257. Prakash C, Staiano A, Rothbaum RJ, Clouse RE. Similarities in cyclic vomiting syndrome across age groups. Am J Gastroenterol. 2001;96:684-8.
258. Li BU, Misiewicz L. Cyclic vomiting syndrome: A brain-gut disorder. Gastroenterol Clin North Am. 2003;32:997-1019.
259. Hasler WL. Dumping syndrome. Curr Treat Options Gastroenterol. 2002;5:139-45.
260. Delgado-Aros S, Camilleri M, Cremonini F, et al. Contributions of gastric volumes and gastric emptying to meal size and postmeal symptoms in functional dyspepsia. Gastroenterology. 2004;127:1685-94.
261. Stanghellini V, Tosetti C, Horowitz M, et al. Predictors of gastroparesis in out-patients with secondary and idiopathic upper gastrointestinal symptoms. Dig Liver Dis. 2003;35:389-96.
262. Revicki DA, Rentz AM, Dubois D, et al. Development and validation of a patient-assessed gastroparesis symptom severity measure: The Gastroparesis Cardinal Symptom Index. Aliment Pharmacol Ther. 2003;18:141-50.
263. Quigley EM, Hasler WL, Parkman HP. AGA technical review on nausea and vomiting. Gastroenterology. 2001;120:263-86.
264. Brzana RJ, Koch KL. Gastroesophageal reflux disease presenting with intractable nausea. Ann Intern Med. 1997;126:704-7.
265. Chial HJ, Camilleri M, Williams DE, et al. Rumination syndrome in children and adolescents: Diagnosis, treatment, and prognosis. Pediatrics. 2003;111:158-62.
266. Rasquin A, Di Lorenzo C, Forbes D, et al. Childhood functional gastrointestinal disorders: Child/adolescent. Gastroenterology. 2006;130:1527-37.
267. Thumshirn M, Camilleri M, Hanson RB, et al. Gastric mechanosensory and lower esophageal sphincter function in the rumination syndrome. Am J Physiol. 1998;275:G314-21.
268. Hoogerwerf WA, Pasricha PJ, Kalloo AN, Schuster MM. Pain: The overlooked symptom in gastroparesis. Am J Gastroenterol. 1999;94:1029-33.
269. Carnett JB. Intercostal neuralgia as a cause of abdominal pain and tenderness. SGO. 1926;12:625-34.
270. Srinivasan R, Greenbaum DS. Chronic abdominal wall pain: A frequently overlooked problem. Practical approach to diagnosis and management. Am J Gastroenterol. 2002;97(4):824-30.
271. Koch KL. Nausea and vomiting. In: Wolfe MM, editor. Therapy of digestive disorders. 2nd ed. Philadelphia: Elsevier; 2006:1003-17.
272. Delaney BC, Wilson S, Roalfe A, et al. Cost effectiveness of initial endoscopy for dyspepsia in patients over age 50 years: A randomised controlled trial in primary care. Lancet. 2000;356:1965-9.
273. Maganti K, Onyemer K, Jones MP. Oral erythromycin and sympathetic relief of gastroparesis: A systemic review. Am J Gastroenterol. 2003;98:259-63.
274. Dhir R, Richter JE. Erythromycin in the short- and long-term control of dyspepsia symptoms in patients with gastroparesis. J Clin Gastroenterol. 2004;38:237-42.
275. Lata PF, Pigarelli DL. Chronic metoclopramide therapy for diabetic gastroparesis. Ann Pharmacother. 2003;37:122-6.
276. Ganzini L, Casey DE, Haffman WF, McCall AL. The prevalence of metoclopramide-induced tardive dyskinesia and acute extrapyramidal movements. Arch Intern Med. 1993;153:1469-75.
277. Barone JA. Domperidone: A peripherally acting dopamine 2-receptor antagonist. Ann Pharmacol. 1999;33:429-40.
278. Patterson D, Abell T, Rothstein R, Koch K, Barnett J. A double-blind multi-center comparison of domperidone and metoclopramide in the treatment of diabetic patients with symptoms of gastroparesis. Am J Gastroenterol. 1999;94:1230-4.
279. Braden B, Enghofer M, Schaub M, et al. Long-term cisapride treatment improves diabetic gastroparesis but not glycaemic control. Aliment Pharmacol Ther. 2002;16:1341-6.
280. Degen L, Matzinger D, Merz M, et al. Tegaserod, a 5-HT4 receptor partial agonist, accelerates gastric emptying and gastrointestinal transit in healthy male subjects. Aliment Pharmacol Ther. 2001;15:1745-51.
281. Lacy BE, Yu S. Tegaserod: A new 5-HT4 agonist. J Clin Gastroenterol. 2002;34:27-33.
282. Andresen V, Camilleri M. Challenges in drug development for functional gastrointestinal disorders. Part 1: functional dyspepsia. Neurogastroenterol Motil. 2006;18:346-53.
283. Parkman HP, Hasler WL, Fisher RS. American Gastroenterological Association technical review on the diagnosis and treatment of gastroparesis. Gastroenterology. 2004;127:1592-1622.
284. Prakash C, Lustman PJ, Freedland KE, Clouse RE. Tricyclic antidepressants for functional nausea and vomiting: Clinical outcome in 37 patients. Dig Dis Sci. 1998;43:1951-6.
285. Hu S, Stern RM, Koch KL. Electrical acustimulation relieves vection-induced motion sickness. Gastroenterology. 1992;102:1854-8.
286. Stern RM, Jokerst MD, Muth ER, Hollis C. Acupressure relieves the symptoms of motion sickness and reduces abnormal gastric activity. Alt Ther Health Med. 2001;7:91-4.
287. Abell T, McCallum R, Hocking M, et al. Gastric electrical stimulation for medically refractory gastroparesis. Gastroenterology. 2003;125:421-8.
288. McCallum R, Lin Z, Wetzel P, et al. Clinical response to gastric electrical stimulation in patients with post surgical gastroparesis. Clin Gastroenterol Hepatol. 2005;3:49-54.
289. Lin Z, Sarosiek I, Forster J, McCallum RW. Symptom responses, long-term outcomes and adverse events beyond 3 years of high-frequency gastric electrical stimulation for gastroparesis. Neurogastroenterol Motil. 2006;18:18-27.
290. Familoni BO, Abell TL, Voeller G, et al. Electrical stimulation at a frequency higher than basal rate in human stomach. Dig Dis Sci. 1997;42:885-91.
291. Lin ZY, McCallum RW, Schirmer BD, Chen JD. Effects of pacing parameters in the entrainment of gastric slow waves in patients with gastroparesis. Am J Physiol. 1998;274:G186-91.
292. McCallum RW, Chen JD, Lin Z, et al. Gastric pacing improves emptying and symptoms in patients with gastroparesis. Gastroenterology. 1998;114:456-61.
293. McCallum RW, Lin Z, Sarosiek I, et al. Preliminary results of multi-point gastric electrical pacing for the treatment of diabetic gastroparesis. [abstract]. Gastroenterology. 2007;132:A112.
294. Lacy BE, Zayat EN, Crowell MD, Schuster MM. Botulinum toxin for the treatment of gastroparesis: A preliminary report. Am J Gastroenterol. 2002;97:1548-52.
295. Bromer MQ, Friedenberg F, Miller LS, et al. Endoscopic pyloric injections of botulinum toxin A for the treatment of refractory gastroparesis. Gastrointest Endosc. 2005;61:833-9.
296. Miller LS, Szych GA, Kantor SB, et al. Treatment of idiopathic gastroparesis with injection of botulinum toxin into the pyloric sphincter muscle. Am J Gastroenterol. 2002;97:1653-60.
297. Parrish CR. Nutrition concerns for the patient with gastroparesis. Curr Gastroenterol Rep. 2007;9:295-302.
298. Jednak MA, Shadigian EM, Kim MS, et al. Protein meals reduce nausea and gastric slow wave dysrhythmic activity in first trimester pregnancy. Am J Physiol. 1999;277:G855-61.
299. Levine ME, Muth ER, Williamson MJ, Stern RM. Protein-predominant meals inhibit the development of gastric tachyarrhythmia, nausea and the symptoms of motion sickness. Aliment Pharmacol Ther. 2004;19:583-90.
300. Lien HC, Sun WM, Chen YH, et al. Effects of ginger on motion sickness in gastric slow-wave dysrhythmias induced by circular vection. Am J Physiol. 2003;284:G481-9.
301. Levine ME, Gillis MG, Koch SY, et al. Protein and ginger for the treatment of chemotherapy-induced delayed nausea. J Alt Comp Med. 2008;14:545-51.
302. Kim CH, Nelson DK. Venting percutaneous gastrostomy in the treatment of refractory idiopathic gastroparesis. Gastrointest Endosc. 1998;47:67-70.
303. Devendra D, Millward BA, Travis SP. Diabetic gastroparesis improved by percutaneous endoscopic jejunostomy. Diabetes Care. 2000;23:426-7.