Chapter 20 Function of the Posterior Cruciate Ligament and Posterolateral Ligament Structures
INTRODUCTION
The purpose of this chapter is to provide a summary of the important biomechanical principles gained from studies from the authors’ laboratory and other investigations regarding the posterior cruciate ligament (PCL) and posterolateral structures (PLS). The primary posterolateral structures of the knee joint are the fibular collateral ligament (FCL) and popliteus muscle-tendon-ligament unit (PMTL), including the popliteofibular ligament (PFL) and posterolateral capsule (PLC). Additional PLS that may be injured and require repair are the iliotibial band femoral and tibial attachments, meniscal tibial and femoral attachments (including fascicles), the fabellofibular ligament, and the lateral and anterior capsule. All of these structures are illustrated in Chapter 2, Lateral, Posterior, and Cruciate Knee Anatomy. Data from these investigations provide the basis for the diagnosis of abnormal knee motion limits in single and combined ligament injuries and allow the surgeon to plan the appropriate ligament reconstructive procedure.
EFFECT OF SECTIONING THE PCL AND PLS ON THE LIMITS OF KNEE MOTION
A series of studies were conducted in cadaveric knees to measure the limits of anteroposterior (AP) translation, internal-external tibial rotation, and varus-valgus rotation (using a six-degrees-of-freedom electrogoniometer) under specific forces and moments with a verified testing apparatus previously described.8,24,25,31,64,97
The PCL and PLS were sectioned in 15 knees first separately and then in combination to measure the resultant abnormal knee motion limits to simulate isolated and combined ligament ruptures.25 The PLS in this investigation included the FCL, PMTL, and PLC. A 100-N force was applied to determine the AP limits, 5 Nm was used for internal-external rotation limits, and 20 Nm was used for adduction-abduction (varus-valgus) limits from 0° to 100° of knee flexion.
In these ligament-cutting experiments, the popliteus tendon was sectioned from the femoral attachment, which effectively removed the entire popliteus muscle tendon and PFL static function. In other experiments to be described,67 the PFL was sectioned independently of the popliteus tendon attachment to investigate the individual function of this ligament. The PLC was removed, including all soft tissue structures posterior to the FCL, to ensure that the individual soft tissue components were removed. This included, in addition to the PLC, additional tissue components comprising the fabellofibular ligament and capsular arm of the biceps femoris short head.
Effect on AP Translation Limits
The normal anterior and posterior translation knee limits are shown in Figure 20-1A. The increase in these limits when the PCL is cut are shown in Figure 20-1B, and the further increase in these limits when the PLS are also sectioned are shown in Figure 20-1C. The values for the increase in motion limits are given in Table 20-1.
TABLE 20-1 Increased Motion Compared With Normal Motion that Occurred when the Indicated Structures Were Sectioned*
The data show that the PCL is a primary restraint to posterior tibial translation throughout knee flexion, with the exception of a small increase in posterior translation at full extension when the PLS are cut. The clinical finding of a knee with increased posterior translation at 30° to 45° of knee flexion, similar to the posterior translation limit at 90° (Fig. 20-2), indicates associated injury to the PLS and the medial structures.
It should be noted that a complete description of the translation limits requires an anatomic measurable point on the tibia, which is selected at the midcoronal point of the tibia. The limits of posterior translation to the medial and lateral tibiofemoral joints are described in a subsequent study.64
Effect on Internal-External Tibial Rotation Limits
The normal knee motion limits to internal and external tibial rotation are shown in Figure 20-3A. There is no increase in external tibial rotation when the PCL is cut alone (see Fig. 20-3B). This finding demonstrates that the PLS are the primary restraint for external tibial rotation throughout knee flexion. When the PLS only are sectioned, an increase in external tibial rotation occurs (see Fig. 20-3C), which is greatest at 30° of knee flexion. The amount of external tibial rotation decreases as the knee is flexed, showing the influence of the PCL in limiting external tibial rotation after the PLS are sectioned.
These data indicate that the PLS provide the primary restraint to external tibial rotation at low knee flexion positions and, therefore, should be tested in this range (20°–40° flexion). Increases in external tibial rotation at 90° indicate injury to both the PLS and the PCL, consistent with the dial test (see Chapter 22, Posterolateral Ligament Injuries: Diagnosis, Operative Techniques, and Clinical Outcomes). The data are in disagreement with the classic interpretation of the posterolateral drawer test at 90° flexion, indicating injury to only the PLS. The resisting function of the FCL, popliteus tendon, and PFL is described in separate studies later in this chapter.
Gollehon and coworkers22 reported an increase in external tibial rotation of 20° flexion after sectioning the PLS at 30° of flexion. Sectioning of the PCL along with the PLS did not produce further significant increases in external tibial rotation (Fig. 20-4).
Effect on Adduction-Abduction Rotation Limits
The normal limits of adduction and abduction rotation are shown in Figure 20-5A. Sectioning the PCL produces very small changes in these limits (see Fig. 20-5B). When the PLS are sectioned alone, large increases in adduction occur (see Fig. 20-5C), indicating that these structures are the primary restraint to this knee motion. Once the PLS are removed, the PCL becomes a primary restraint and large increases in the adduction limit occur with knee flexion.
The limits to adduction (varus angulation) are graphed in Figure 20-625 after sectioning the FCL, the PLS (PMTL and PLC), and all structures (PCL, FCL, PMTL, and PLC). The data demonstrate that the FCL is the primary restraint for lateral joint opening, with further increases after the remaining PLS are sectioned.
Markolf and associates52 developed an in vitro system in cadaveric knees in which load transducers were used to measure ACL and PCL forces under different loading situations. After sectioning the FCL and the other PLS, large increases were noted in resisting tensile forces in both cruciate ligaments under varus loading (Fig. 20-7) and in the PCL under posterior tibial loads and external tibial torques (Fig. 20-8). These findings are in agreement with prior studies, because the loss of the PLS converts both cruciate ligaments into primary restraints for varus loading, in which the ligaments are poorly positioned with small lever arms to resist this motion.
One problem with cadaveric studies that measure knee motion limits after ligament sectioning is that the true resulting millimeters of anterior or posterior translation (subluxation) of the medial and lateral tibiofemoral compartments are not measured or described. For example, when 15° of increased external tibial rotation is measured after cutting the PLS, the resultant abnormal millimeters of posterior translation of the lateral tibial condyle are unknown. This is because it is also necessary to measure and compute the center of tibial rotation to determine the effect of the increase in degrees of external tibial rotation on the lateral and medial tibiofemoral compartments. There are few published data on the true millimeters of tibial subluxation of the medial and lateral compartments (rotatory subluxations) that occur after knee ligament injuries, which are discussed in further detail later in this chapter.64
ROLE OF THE POSTERIOR OBLIQUE LIGAMENT
Petersen and colleagues,69 in a cadaveric study using a robotic testing system, examined the restraint of the superficial medial collateral ligament (SMCL), the deep medial collateral ligament (DMCL), the posterior oblique ligament (POL), and the posteromedial capsule (PMC) in resisting posterior tibial translation after PCL sectioning. The data shown in Figure 20-9 demonstrate the increase in posterior tibial translation after sequential ligament sectioning of the medial and posteromedial structures. The authors concluded that the POL has a much larger role than the SMCL and DMCL in resisting posterior tibial translation and internal tibial rotation. It should be noted that these two structures were first sectioned and no studies were done when the POL was sectioned first. This indicates that there could be a sectioning artifact introduced in the study. Even so, there are posteromedial oblique capsular fibers from the medial femoral condyle to the tibia, just posterior to the SMCL, that resist internal tibial rotation and posterior tibial translation (after PCL sectioning). The study concluded discrete oblique fibers form the middle arm of the POL described by Hughston and Eilers.36 Of interest in this cadaveric study was the finding that a valgus loading of the knee joint close to knee extension (with PCL deficiency) produced an increased posterior tibial translation of the medial compartment with absence of the POL and PMC.
Robinson and coworkers74 conducted a cadaveric study of the medial and posteromedial structures and could not identify a distinct separate POL structure, but did identify an oblique portion of the PMC at which fibers could be tensioned under internal tibial rotation loading. Robinson and associates73 and Haimes and colleagues31 studied the contribution of the PMC, which included the POL. Robinson and associates73 reported that the PMC resisted 28% of the posterior tibial load when the tibia rotated freely in the extended knee, which rose to 42% when the tibial was subjected to internal rotation. These authors concluded the PMC resisted posterior tibial translation close to full extension and less so with knee flexion, which relaxes the PMC. With knee flexion, the SMCL resisted posterior tibial translation.
FUNCTION AND ROLE OF THE PMTL
The PMTL includes (1) the muscular tibial origin, (2) the fibular origin (PFL), (3) the merging of both of these components to the popliteal tendon inserting on the lateral femoral condyle, (4) the inferior and superior popliteomeniscal fascicles forming the popliteal hiatus, and (5) the soft tissue attachments to the lateral meniscus and posterior tibia. The static stabilizing function of the PMTL that requires surgical reconstruction has been debated.40,61,67 The authors advanced the hypothesis that the PFL has a secondary role in that it provides a functional attachment of the popliteus tendon to the fibula. However, removal of this fibular attachment has little effect on the limits of motion as long as the popliteal tendon femoral and tibial attachments remain intact.67 This hypothesis questions operations in which only the PFL is reconstructed, without attention to the FCL or popliteus tendon femoral and tibial attachments.54,78,86
As described previously, Grood and coworkers25 reported that increases in external tibial rotation (posterior subluxation lateral tibial plateau) is highly dependent on the order of sectioning of the PLS. The last remaining PLS sectioned will, in general, provide the largest effect in terms of increased knee motion limits after the other primary and secondary restraints have been sectioned. Therefore, the experimental design of the order of ligament sectioning may induce an artifact and an incorrect assignment of a primary restraining role to a soft tissue structure. Shahane and associates78 in a cadaveric study concluded that the PMTL was the primary restraint to external tibial rotation and the FCL was a secondary restraint. However, this study did not include sectioning the FCL first to determine whether increases in external tibial rotation occurred prior to sectioning the PMTL. In contrast, Veltri and colleagues92,93 reported that sectioning the PFL (after first sectioning the FCL, popliteus tendon intact) resulted in only small and minor increases in external tibial rotation.
Critical Points FUNCTION AND ROLE OF THE POPLITEUS MUSCLE-TENDON-LIGAMENT UNIT
Popliteus muscle-tendon-ligament (PMTL) includes
The cadaveric study by Pasque and coworkers67 in the authors’ laboratory implemented an experimental system similar to that already described,24,25 which used an instrumented spatial linkage and loading of the knee joint with a 100-N posterior force, a 10-Nm varus force, and a 5-Nm external moment from 0° to 120° of knee flexion. The ligament sectioning of the PLS was varied in order to more precisely define the restraining function of the individual structures. The results are shown in Figures 20-10 and 20-11. Sectioning the PFL in the intact knee produced no statistically significant changes in motion limits, whereas sectioning both the popliteus tendon and the PFL together (removing the function of the PMTL) produced a small increase in external rotation from 3° to 5°.
In a separate study in the authors’ laboratory, Wroble and associates97 reported the effect on external tibial rotation when only the popliteus tendon was sectioned in ACL-deficient knees (Fig. 20-12), and when only the FCL was sectioned along with the iliotibial band and lateral capsule (Fig. 20-13). Consistent with the studies previously discussed, only small increases in external tibial rotation were found when individual PLS were sectioned.
All of the PLS provide important restraints to external tibial rotation, whereas the PFL serves as a secondary restraint in providing a fibular origin to the PMTL. In acute knee injuries, this fibular attachment should be repaired if possible; however, graft reconstruction for the FCL and popliteus tendon tibial-femoral attachments is advocated (see Chapter 22, Posterolateral Ligament Injuries: Diagnosis, Operative Techniques, and Clinical Outcomes). The FCL functions to limit external tibial rotation, particularly at low knee flexion angles, and is an important structure to reconstruct in posterolateral injuries. Sugita and Amis87 measured the orientation of the FCL and PFL in cadaveric knees and reported that the FCL slackened with increasing knee flexion, achieving a vertical position at 70° flexion, which decreased its ability to resist external tibial rotation. The PTML maintained an oblique orientation, which allowed effective resistance of external tibial rotation with increasing knee flexion.
LaPrade and colleagues41 conducted an in vitro cadaveric knee study in which a specially designed buckle transducer was attached to the FCL, popliteus tendon, and PFL. The knee joints were subjected to AP forces (67 N), varus-valgus torques (12 Nm) and internal-external rotation torques (6 Nm). A reciprocal relationship of load-sharing with knee flexion was found, because the FCL had higher loads resisting the external rotation torque at low flexion positions and the popliteus complex had higher loads at 60° and 90° of knee flexion (Fig. 20-14). Even though buckle transducers have inherent problems in truly measuring ligament tensile-resisting loads, the results show that all three structures are important in resisting external tibial rotation loads and the presumed importance of anatomic reconstruction of these structures.
Application to Surgical Reconstruction
The authors have encountered knees requiring revision of a failed posterolateral procedure in which only the PMTL was reconstructed, leaving a partially or completely insufficient FCL. The abnormal lateral joint opening places high tensile forces on the PMTL that is not designed to resist varus moments (in the absence of a functional FCL). Similarly, performing only an FCL reconstruction in knees with a complete PMTL disruption subjects the FCL graft to external tibial rotation moments without the added load-sharing of the PMTL. At surgery, it is true that reconstructing only the FCL or the PMTL will initially eliminate a majority of the abnormal motions (lateral joint opening, external tibial rotation). However, the problem remains that the single reconstruction will be subjected to much higher in vivo loading conditions with the added load-sharing of the remaining competent PLS. Under these conditions, there is an increased risk of a return of knee instability. An anatomic reconstruction of the PLS with the goal of restoring the PLC and meniscus attachments, PMTL, and FCL is described in Chapter 22, Posterolateral Ligament Injuries: Diagnosis, Operative Techniques, and Clinical Outcomes.
POSTERIOR SUBLUXATIONS OF THE MEDIAL AND LATERAL TIBIOFEMORAL COMPARTMENTS
The millimeters of posterior subluxation of the medial and lateral tibiofemoral compartments after sectioning the PCL and PLS were measured in whole cadaver lower limbs in a testing apparatus previously described.64 The position of the lateral and medial tibial plateaus was determined by three reference points: the AP translation of a point located at the center of each plateau, and the medial and lateral location of points located midway between the tibial edges and the joint centers.
The purposes of this investigation were to, first, determine the AP position of the medial and lateral tibial plateaus when a known external rotation torque was applied at 30° and 90° of knee flexion. Second, to measure how the positions of the lateral and medial tibial plateaus change and the magnitude of this change (subluxation) after sectioning the PLS alone and in combination with the PCL. The anterior and posterior meniscofemoral ligaments were also sectioned when present. Studies have shown that these structures are not the primary restraints for posterior tibial translation or external rotation and provide a restraint only after the PCL has been removed.29,34,42 The study also determined the effect of physiologic joint laxity, or preexisting ligament laxity, on the magnitude of rotatory subluxation of the tibia after sectioning the PLS alone and in combination with the PCL.
Critical Points POSTERIOR SUBLUXATIONS OF THE MEDIAL AND LATERAL TIBIOFEMORAL COMPARTMENTS
Posterior Tibial Translation
External Tibial Rotation
Effect of Physiologic Laxity
Posterior Tibial Translation
The mean values of AP translation and external tibial rotation are shown in Figure 20-15 for intact knees and after sectioning the PLS and PLS/PCL. Sectioning only the PLS resulted in a mean increase in posterior translation of the lateral tibial plateau of 8.0 mm at 30° of flexion over the intact state (P < .01), and a smaller increase of 2.7 mm at 90° of flexion. There was no significant increase in posterior translation of the medial tibial plateau at either flexion angle. There was a large range of posterior tibial subluxations at both 30° (Fig. 20-16) and 90° of knee flexion (Fig. 20-17).
External Tibial Rotation
The mean values of the external tibial rotation limits for the intact knees and after sectioning the PLS and PLS/PCL are shown in Table 20-2. Cutting the PLS caused a significant increase (P < .01) in external tibial rotation at both 30° (mean increase, 13.0°) and 90° (mean increase, 5.4°) of flexion. Sectioning the PCL along with the PLS caused a further increase in external tibial rotation at 30° (mean increase, 5.0°; not significant) and at 90° of flexion (mean increase 15.1°, P < .01). Considerable variability was present between specimens in the amount of measured external tibial rotation at 30° (see Fig. 20-13) and 90° (see Fig. 20-14) of flexion.
TABLE 20-2 External Rotation Limits (100 N Posterior Force, 5 Nm External Moment)
Flexion | External Rotation (°; Mean ± SD) |
---|---|
30° | |
Intact | 18.2 ± 3.9 |
PLS* cut | 31.2 ± 5.0 |
PLS, PCL cut† | 36.2 ± 4.7 |
90° | |
Intact | 17.4 ± 3.5 |
PLS cut | 22.8 ± 5.0 |
PLS, PCL cut† | 37.9 ± 3.0 |
SD, standard deviation.
* PLS, posterolateral structures including fibular collateral ligament (FCL).
† PCL, posterior cruciate ligament and ligaments of Humphry and Wrisberg cut in addition to the PLS and FCL.
From these data, a classification system of rotatory subluxations was devised based on two concepts: the final position of the medial and lateral tibial plateaus under defined loading conditions (such as with either internal or external tibial rotation at a defined knee flexion angle) and the position of each plateau. There are three possible positions for each plateau: anterior subluxation, normal position, or posterior subluxation. For each of these positions of the lateral tibial plateau, there exist three corresponding positions for the medial tibial plateau (Fig. 20-18).
Under the same loading conditions at 90° of flexion, sectioning only the PLS resulted in a small (2.7-mm) increase in posterior translation of the lateral tibial plateau. These data agree with the external tibial knee motion limits previously reported,25 in which small and insignificant increases in external tibial rotation occurred at 90° after PLS sectioning. When the PCL was also sectioned, the lateral tibial plateau demonstrated a gross posterior subluxation, with a mean posterior translation of 34.2 ± 3.5 mm (23.5 mm from normal).
Effect of Physiologic Laxity
In a prior study, the authors62 reported that the clinician may frequently diagnose a posterolateral injury when, in fact, the increase in external tibial rotation is due to a medial collateral ligament (MCL) injury. For this reason, during clinical testing for tibial rotatory subluxation, the examiner must palpate the position of the medial and lateral tibial plateaus to qualitatively determine whether an anterior position of the medial plateau and a posterior position of lateral tibial plateau occur with external tibial rotation. This is why the rotation tests should be done in the supine position (and not the prone position) to enable the examiner to observe and palpate the relative step-off of both the medial and the lateral tibial plateaus at 30° and 90° of flexion. Still, it may be difficult to quantify the amount of posterior subluxation of the lateral tibial plateau with PLS injuries, and additional tests such as lateral joint opening under varus loading and varus recurvatum help to confirm the diagnosis.
In Figure 20-19, the external rotation dial test is shown for a cadaveric knee measured with a three-dimensional goniometer with 5 Nm load under conditions already described.62 The increase in the external rotation limit and anterior translation of the medial tibial plateau is shown in the normal knee and then after sectioning the ACL and SMCL. The tibial rotation increased from 7° to 16° and was associated with a lateral shift in the axis of tibial rotation. This resulted in an increase in anterior translation of the medial tibial plateau from 3.2 mm to 8.5 mm (anteromedial subluxation). In this study, an examination of cadaveric knees was performed by 11 experienced knee surgeons who were blinded as to the sectioned ligaments and asked to determine a diagnosis. More than half of the surgeons misinterpreted the increase in external tibial rotation as an injury to the PLS. This indicates that tests for rotatory subluxations of the knee joint are limited by the ability to quantitatively determine the millimeters of AP translation of the medial or lateral tibiofemoral joint under external rotation loads and variability between examiners should be expected.
EFFECT OF PCL AND POSTEROLATERAL RECONSTRUCTION ON RESTORING NORMAL KNEE MOTION LIMITS
Sekiya and coworkers77 conducted an in vitro cadaveric study using a robotic testing system in which the PCL was reconstructed with a two-strand construct (Achilles and semitendinosus tendons), the PFL was reconstructed with a gracilis tendon, and the popliteus tendon was reattached to its femoral origin. The specimens were subjected to a 134-N posterior tibial load and a 5-Nm external tibial torque at multiple flexion angles. The authors reported that the forces in the posterolateral grafts were significantly higher than those in the native PLS. A possible explanation for the increased loads on the posterolateral reconstruction is the fact that the two-strand PCL reconstruction did not restore normal posterior tibial translation, because significant increases in posterior tibial translation were detected from 0° to 90° of flexion compared with intact knees. The PCL grafts were tensioned to a defined load, but not to the load required to reduce posterior tibial translation to normal; therefore, the PCL reconstruction forces were low after the reconstruction. For this reason, additional loads were placed upon the posterolateral grafts.
The data from the investigation by Sekiya and coworkers77 and a subsequent study32 show the dependence of posterolateral reconstructions on a functional PCL reconstruction. Harner and associates32 studied the effect of deficiency of the posterolateral structures on PCL Achilles tendon graft reconstructions in 10 cadaver knees. The authors reported that a functional PCL reconstruction (posterior tibial translation restored to within 2.4 ± 1.4 mm of the intact knee, 90° flexion) subjected to sectioning of the PLS resulted in a significant increase in posterior tibial translation (6.0 ± 2.7 mm at 30°, 4.6 ± 1.5 mm at 90° of flexion; P < .05). In addition, the forces in the PCL graft significantly increased for posterior tibial load, external tibial torque, combined posterior and external torque, and varus torque. These data support other studies that conclude that a PCL reconstruction may stretch out or fail if a concurrent injury to the PLS is not diagnosed and concurrently surgically addressed.52,59
LaPrade and colleagues40 conducted a biomechanical analysis of an anatomic posterolateral reconstruction in which a two-graft technique was used to replace the FCL, popliteus tendon, and PFL. The reconstructions were performed using Achilles tendon–bone constructs in 10 cadaver knees aged 62 to 74 years (Fig. 20-20). It should be noted that in the LaPrade and colleagues40 reconstruction, the PFL does not attach to the popliteus tendon at its normal anatomic attachment, and therefore, this represents an anatomic reconstruction of the popliteus tendon and FCL. The knees were tested under a 5-Nm varus moment and 5 Nm external rotation torque first with the posterolateral structures intact; second, with the FCL, popliteus tendon, and PFL sectioned; and third, after the posterolateral reconstruction. There was no significant difference between the intact and the reconstructed knees for varus translation at 0°, 60°, and 90° or for external tibial rotation at 0°, 30°, 60°, and 90° of flexion. The authors concluded that this technique restored static stability and provides the scientific basis for patient outcome studies. The authors of this chapter agree with this conclusion, because both the popliteus tendon (static attachments) and the FCL were reconstructed.
Apsingi and coworkers2 reported on the kinematics of cadaveric knees with a combined PCL and posterolateral ligament deficiency. The authors used both a single-bundle and a double-bundle PCL reconstruction that was combined with a femoral-fibular graft reconstruction for the posterolateral structures. The combined PCL and posterolateral reconstruction restored all the laxity limits to normal, and the double-bundle PCL reconstruction was not reported to have an advantage over the single-bundle reconstruction. Of importance, the nonanatomic femoral-fibular reconstruction involved two femoral tunnels with the graft passed in an anterior-to-posterior direction through the fibula. The location of the two femoral tunnels was determined by selecting isometric points on the lateral femoral condyle and not by attaching the two graft arms to any specific anatomic location. The two graft ends passed through the two femoral tunnels were tensioned independently to restore the adduction and external rotation limits to normal. The anterior limb was tensioned at 20° of flexion to match the laxity of the intact knee to varus loading. The posterior limb was tensioned at 90° of flexion to match the intact knee motion limit to external rotation torque. Each graft arm was only 5 mm in diameter. The data from this study and others on femoral-fibular reconstructions, if interpreted incorrectly, may suggest that the more simplified femoral-fibular reconstruction is all that is required for posterolateral insufficiency instead of the more complex anatomic reconstruction recommended by the senior author and LaPrade.40 It is true that in a time-zero cadaveric study, a single graft appropriately tensioned will restore varus and external rotation motion limits to normal.
Two points are worthy of emphasis. Apsingi and coworkers2 did not measure the effects of the femoral-fibular reconstruction on decreasing or unloading the PCL graft. Markolf and associates50 showed that an FCL reconstruction alone was insufficient to decrease or unload PCL graft forces. The Markolf and associates data50 provide added support to the concept of restoring all of the functional components of the PLS at the time of surgery. Second, a small-diameter graft passed through the fibula and secured to the femoral condyle may be insufficient to resist the large adduction and external rotation loads that occur postoperatively. The anatomic reconstruction advocated by Noyes60 and LaPrade40 places two larger-diameter grafts at both the femoral, the fibular, and the tibial attachment sites of respective PLS. The graft function, therefore, does not depend upon only a fibular attachment site, and the popliteus tendon graft is passed through a distal tibial tunnel. A third point on the application of biomechanical studies to clinical cases involves whether to select an isometric point on the femur for the FCL and popliteus tendon50,82 or, as described by some authors, to select the anatomic attachment site to restore native anatomy.40,60 Sigward and associates82 reported, in a cadaveric knee study, the femoral fixation sites for isometry of posterolateral reconstructions. A coordinate grid system was located across the lateral femoral condyle, permitting the placement of 21 holes in the femur, 5 mm apart, for the measurement of suture length changes with knee flexion. The authors did compute a mean location for the FCL and popliteus tendon attachment; however, the femoral isometric points showed major differences between knees. This wide variability between specimens indicates that one mean value for the ideal isometric point for a graft placement would most likely be incorrect for knees undergoing surgery. Future clinical studies are necessary to determine the ideal success rates from knees in which either an isometric point is selected at surgery or an anatomic attachment site is selected. Until these studies are available, the senior author recommends anatomic attachment points and an anatomic reconstruction for posterolateral instability as described in Chapter 22, Posterolateral Ligament Injuries: Diagnosis, Operative Techniques, and Clinical Outcomes.
CLINICAL EFFECTS OF POSTERIOR TIBIAL SUBLUXATION OF THE PCL-DEFICIENT KNEE
Morrison58 and Denham and Bishop16 estimated that PCL forces up to 50% body weight occur during level walking. Even higher PCL forces have been calculated during stair-climbing, ascending stairs, and descending stairs4 (see Chapter 23, Rehabilitation of Posterior Cruciate Ligament and Posterolateral Reconstructive Procedures, for other studies on estimated PCL forces during activity). In a study at the authors’ laboratory10 involving 10 patients with complete PCL ruptures, the amount of posterior tibial translation that occurred during a double-stance static squat test was measured. A lateral radiograph was obtained of both lower limbs, which was used to compute the amount of posterior tibial translation and sagittal plane forces on the PCL-deficient knee and the normal knee. A stress radiograph was taken with an 11-kg posterior force (108 N) to provide an objective measurement of the amount of posterior translation in the PCL-deficient knee compared with the opposite normal knee.
As noted by Daniel and colleagues,14 the quadriceps neutral angle is the angle of knee flexion at which the course of the patellar tendon is perpendicular to the articular surface of the tibia. The neutral knee flexion angle may vary from 60° to 90°, with a mean of 71°. Below this angle, the patellar tendon lies anterior to the tibial tubercle and produces a posterior tibial shear force. Conversely, at a greater flexion angle, the patellar tendon has a line of action coursing posterior to the attachment to the tibial tubercle, producing an anterior tibial shear force. The quadriceps neutral angle varies sufficiently between knees and is not an objective measurement in terms of the diagnosis of posterior tibial subluxation. However, patients with PCL-deficient knees frequently note an anterior shifting of the tibia from its posterior subluxated position when they attempt to stand from a seated position, which can be easily demonstrated in the clinic.
Experimental studies have documented significantly increased contact pressures in the medial tibiofemoral and patellofemoral compartments after PCL sectioning.21,43,84 Skyhar and coworkers84 reported that PCL sectioning caused significant increases in contact pressure on the medial tibiofemoral compartment (29.1 ± 11.6 Pa) at all knee flexion angles compared with intact specimens (19 ± 3.4 Pa; P < .05). A significant elevation in contact pressure in the patellofemoral compartment was found only at 60° of flexion. Gill and associates21 analyzed patellofemoral contact pressures under simulated muscle loads in both PCL-sectioned and PCL-reconstructed cadaver knees (Achilles tendon single-bundle allograft). Significantly greater peak contract pressures were measured in the PCL-deficient knees than in the intact knees under both an isolated quadriceps (400 N) and a combined quadriceps and hamstrings (400 N/200 N) load. The PCL-reconstructed knees also had elevated pressures, which were not significantly different from those measured in the PCL-deficient specimens. There was no effect of knee flexion angle on these findings. The residual posterior tibial translation after the PCL reconstruction was not determined.
MENISCOFEMORAL LIGAMENTS
Gupte and colleagues27 conducted an analysis of 16 anatomic studies involving 1022 cadaveric knees and reported that 91% had at least one meniscofemoral ligament (MFL). The anterior meniscofemoral ligament (aMFL) was identified in 390 knees (48%), the posterior meniscofemoral ligament (pMFL) was identified in 569 knees (70%), and both the aMFL and the pMFL were found in 257 knees (32%). It is important to identify the aMFL and pMFL (when present) to determine the true PCL anatomic footprint for graft placement, particularly when a two-strand graft construct is used.30 The anatomic attachment of the aMFL is distal to the PCL attachment (Fig. 20-21), which gives the appearance that the PCL footprint is adjacent to the articular cartilage when, in fact, the true PCL attachment is a few more millimeters proximal.
The cross-sectional area of the aMFL ranges from 6.8 to 7.8 mm2, and the cross-sectional area of the pMFL ranges from 6.7 to 12.7 mm2.27 For the surgeon, this indicates that these structures will be encountered at the time of PCL surgery. The mean ultimate loads of the aMFL and pMFL have been reported to be 265 ± 152 N and 443 ± 287 N, respectively.38 A comparison of the ultimate strength of the MFLs and the PCL is not possible because published cadaveric data are from older specimens tested at reduced failure loads than would be expected.
Critical Points MENISCOFEMORAL LIGAMENTS
Gupte and colleagues27 reviewed the numerous theories and evolutionary perspectives on the function of the MFL and concluded that there are insufficient data to support a functional role of these structures in humans. In animals such as sheep, horses, and dogs, the lateral meniscus posterior horn is attached by virtue of the pMFL without a separate posterior tibial attachment. In humans, the posterior horn of the lateral meniscus has two separate attachments into the tibia and, when the pMFL is present, an additional attachment into the femur. In rare cases, the posterolateral meniscus tibial attachment is absent and attaches only by the femoral pMFL, which must be preserved with any surgical procedure such as PCL reconstruction. The Wrisberg-type discoid lateral meniscus may have the pMFL as the only attachment, with an absent posterior tibial attachment. Gupte and colleagues27 noted that the pMFL is tight in both knee extension and deep knee flexion. It has been theorized that the pMFL may pull the lateral meniscus into the joint during flexion, causing clicking and snapping symptoms leading to meniscus deteroriation.17 It is possible that the pMFL provides additional resistance to posterior displacement of the lateral meniscus posterior horn during maximum tibial external rotation; however, this has not been experimentally proved.
The effect of the MFLs acting as a secondary restraint to posterior tibial translation was investigated by Gupte and coworkers28 in cadaveric knees. These authors postulated that the MFLs provide to the PCL a “synergistic reinforcement” in resisting posterior tibial translation. In this study, the PCL was first divided and the increase in posterior tibial translation measured, then the MFLs were divided (Figs. 20-22 and 20-23). The data showed that the MFLs contributed 28% of the restraining force at 90° of knee flexion without any restraint for rotatory subluxations. The data show that partial or isolated tears of the PCL may be supported in part by intact MFL structures, resulting in less overall posterior tibial translation. This suggests the potential benefit of preserving when possible at surgery the function of the MFLs, which is often difficult in double-bundle PCL reconstructions. This also provides the rationale for protecting knees with isolated PCL ruptures, using a postoperative brace at full extension with a calf pad for 4 weeks to allow initial healing. The secondary ligament restraints maintain a normal tibiofemoral joint position at full extension, maintaining a normal PCL tibiofemoral attachment distance.
Bergfeld and associates5 conducted a cadaveric study on 20 knees in which total AP translation was measured in four different flexion angles after cutting the PCL and MFLs. Testing was performed with the tibia in neutral, internal, and external rotation (5 Nm torque). Statistically significant decreases in posterior tibial translation occurred with internal and external tibial rotation (Fig. 20-24 and Table 20-3). The authors concluded that 90° knee flexion and neutral tibial rotation was the best position to test for maximum posterior translation and that the medial and lateral secondary restraints were tensioned to reduce the posterior limit with internal-external tibial rotation. Because the MFLs were cut, other structures limited posterior translation in internal tibial rotation and not the MFLs, as had been reported previously.11 Note in Chapter 3, The Scientific Basis for Examination and Classification of Knee Ligament Injuries, after sectioning the PCL, the posterior tibial translation increased 12.1 ± 0.6 mm and 15 of 22 specimens had increases greater than 10 mm. This indicates that the amount of posterior translation determined by secondary restraints will vary depending on their physiologic laxity. Therefore, the frequently reported value of greater than 10 mm posterior translation indicating injury to the secondary restraints cannot be used. The secondary restraints may be lax owing to either physiologic laxity or injury.
Gupte and colleagues26 introduced the concept of a “meniscal tug test” at arthroscopy, which involves placing tension on the aMFL with a nerve hook and observing movement in the posterior horn of the lateral meniscus. The aMFL was confirmed in 88% of 68 knees, whereas the pMFL was identified in only 9%. The authors concluded that this test could be used to distinguish between fibers of the PCL and the MFLs, which is helpful in avoiding a misdiagnosis of a partial versus a complete PCL tear.
POPLITEOMENISCAL FASCICLE ATTACHMENTS
Staubli and Birrer85 conducted anatomic dissections of the popliteus tendon and its fascicles and described their relationship to the lateral meniscus attachments in cadaveric knees. These authors also compared arthroscopic examinations of the popliteus-lateral meniscus attachments in a normal control group of 107 patients with those of 68 patients with acute and chronic ACL disruptions. Structural lesions of the popliteus meniscus attachments were observed in approximately 19% of control knees compared with 86% to 95% of ACL-deficient knees. The authors concluded that the surgeon should determine whether an anterior subluxation and disruption of lateral meniscus attachments is present, requiring operative repair at the time of ACL reconstruction.
Critical Points POPLITEOMENISCAL FASCICLE ATTACHMENTS
Simonian and coworkers,83 in a cadaveric study, transected the anteroinferior fascicle and then the posterosuperior fasciculi to determine the contribution of these structures to lateral meniscus stability. The mean lateral meniscal motion under a 10-N load was 3.6 mm. After the anteroinferior fascicle was cut, an increase in anterior displacement of the posterior horn of the lateral meniscus of 1.8 mm (50%) occurred, and when both fasciculi were cut, an increase of 2.8 mm (78%) was reported. The authors concluded that the popliteomeniscal fasciculi contributed to lateral meniscus stability and acknowledged the difficulty in objectively determining abnormal meniscus displacements at the time of arthroscopic surgery. Tests for abnormal lateral meniscus displacement at the time of arthroscopic surgery and the repair of the popliteomeniscal fascicles are discussed in Chapter 28, Meniscus Tears: Diagnosis, Repair Techniques, and Clinical Outcomes.
PCL FIBER FUNCTION: SCIENTIFIC BASIS FOR PLACEMENT OF PCL GRAFTS AT TIBIOFEMORAL LOCATIONS
The anatomy of the cruciate ligaments has been described for over a century in qualitative terms as having two major bundles or bands. The Weber brothers in 183694 and Fick in 191120 published some of the first anatomic descriptions of the ACL and PCL; however, these authors did not conduct biomechanical studies to support their descriptions. The PCL has been classically described as a structure composed of an anterolateral bundle that is tense in flexion and a posteromedial bundle that is tense in extension. This nomenclature is still used today, even though it is incorrect because it does not precisely describe the function of the PCL fibers and gives the surgeon inadequate data for determining placement of PCL graft strands (see Chapter 21, Posterior Cruciate Ligament: Diagnosis, Operative Techniques, and Clinical Outcomes).
Inderster and associates37 divided the PCL into three bundles: anterolateral, posteromedial, and posterior oblique. These authors reported that the femoral-to-tibial distances of the anterolateral and posteromedial bundles increased with knee flexion from 0° to 90°, whereas the length of the posterior oblique bundle remained the same. Accordingly, they recommended a more posterior position of a PCL reconstruction at the femoral attachment of the posterior oblique bundle. The authors did not induce posterior loads on the tibia to determine femoral-tibial length changes under loading conditions, nor did they measure tibial displacements after PCL reconstruction.
Covey and colleagues12,13 described a fiber continuum of the PCL consisting of four regions: anterior, central, posterior longitudinal, and posterior oblique. This four-fiber region classification was confirmed by Makris and coworkers44 according to fiber orientation and osseous sites of attachment visualized in 24 cadaver knees. The anterior fiber region attachment was located at the most anterior portion of the femoral attachment and inserted into the anterior portion of the tibial attachment. The central fiber region was the widest portion, representing the middle part of the femoral attachment, and inserted into the middle and slightly lateral aspect of the tibial attachment. The anterior and central fiber regions represented nearly 80% of the PCL, with the posterior fiber regions accounting for the remaining 15% to 20%. The posterior fiber region consisted of a posterior longitudinal attachment to the tibia medially and a posterior oblique region attachment to the tibia laterally.
These investigations reported that under operative conditions, most of the PCL’s attachment sites exhibit nonisometric behavior. Only the small posterior fiber attachment sites (which account for 15% of the PCL44) demonstrate near isometry. In addition, Covey and colleagues12 reported marked loosening of most PCL fibers by an applied quadriceps force at knee flexion angles less than 75° when compared with an unloaded state. Internal tibial rotation slackened certain fibers (anterior, central) near extension, but significantly tightened others (central, posterior) with flexion. The authors concluded that the joint angle and type of load applied to the knee joint produce different functional roles to the four fiber regions of the PCL.
Quantitative data from many investigators have been reported using a variety of techniques to determine the length-tension behavior of the PCL fibers under loaded conditions. These measurement conditions include the use of force measurement sensors,3,46,52 wire cables,18 mercury strain gauges,19,39 and photogrammetric techniques.15,88,90,91 Ahmad and associates1 performed an analysis of PCL bundle length change and angle of orientation with knee flexion and extension. The anterolateral bundle was observed to become more vertical with increased knee flexion and the posteromedial bundle became more horizontal. Therefore, the posteromedial bundle has a better orientation to resist posterior tibial translation owing to its horizontal position. The study data are in agreement with other studies showing the marked changes in the length of PCL fibers with knee flexion and extension based on their femoral attachment.
The use of an instrumented spatial linkage during application of joint loads (followed by digitization of the tibia and femoral surfaces and ligament attachment locations) allows the length of discrete fiber strands or regions within a ligament to be measured based on tibiofemoral attachment sites and the change in distance (fiber length) with knee motion.23,33,76 This information is required to determine locations of graft attachments whose tibiofemoral length changes the least with knee flexion or, alternatively, graft attachment locations at which the fiber length changes the most. The surgeon should select graft attachment locations that have the least amount of change in tibiofemoral length, and tension the graft at the knee flexion position where the graft length is the longest (and therefore functional). For example, if a graft is tensioned at a knee flexion angle where the tibiofemoral fiber length is at its shortest, the graft will initially constrain knee flexion or extension and fail as the graft construct (tibiofemoral distance) lengthens with further knee flexion or extension.
The PCL ligament length pattern is highly dependent on a proximal-to-distal direction of the femoral attachment, and not an anterior-to-posterior direction, as proposed by the anterolateral-posteromedial bundle concept previously discussed. In Figure 20-25, the tibiofemoral separation distances are shown for a number of proximal-to-distal locations that pass through the PCL femoral attachment. A transitional zone (<2 mm length change) adjacent to the center of rotation is shown. All attachment locations distal to line A-A lengthen with knee flexion, and all fibers proximal to this line shorten with knee flexion. In Figure 20-26, the average tibiofemoral separation length is shown along three femoral attachment site locations. Two sites are within the PCL femoral attachment and one is outside the PCL attachment. These data demonstrate that the fiber length change is minimal in an anterior-to-posterior direction within the PCL attachment. These experimental findings were corroborated by Sidles and colleagues,81 Ogata and McCarthy,65 and Trus and coworkers.89
The changes in tibial attachment location show only a small effect on the tibiofemoral separation distance, as demonstrated in Figure 20-27. These experimental conditions are valid assuming a PCL fiber follows a straight path between attachment sites. Native PCL fibers do not follow straight paths, but may have curvatures induced from other fibers. As yet, there is no description of fiber anatomy suggesting major PCL fiber curvature from one attachment site to another.
A simplified planar model may be used to explain the larger effect of the femoral attachment on PCL fiber length change in comparison with the tibial attachment. The model is shown in Figure 20-28, where the tibia is stationary and the femur rotates about a fixed center. The femur is positioned at 45°, and the change in tibiofemoral distance is shown relative to the center of rotation with knee extension and flexion to 90°. The planar model does not describe the true three-dimensional behavior of the PCL fibers, which is more complex. However, the model does show how the fiber length change is dependent on its femoral attachment relative to the center of femoral rotation, which is simplified to a fixed point. The reader is referred to Chapter 3, The Scientific Basis for Examination and Classification of Knee Ligament Injuries, for further information on ACL and PCL fiber function.
In Figure 20-29, the center transitional zone (<2 mm change in tibiofemoral length) is shown. The zone is located in the proximal one third of the PCL femoral attachment toward the roof of the intercondylar region, 11 mm from the articular cartilage distally, extending approximately 10 mm from the roof in a posterior direction. The data show that the majority of the PCL lengthens with knee flexion. The contour map showing the length change from 0° to 90° is shown in a planar manner in Figure 20-30. A previous study showed that a graft placed into a nonisometric location, just distal to the transitional zone, demonstrated a few millimeters increase in tibiofemoral distance with knee flexion, providing greater control of posterior tibial displacement than a graft placed in a so-called isometric zone.23 These data show that any graft placed within the PCL attachment will have portions that undergo different elongations along every 2 to 3 mm in graft cross-sectional area, progressing in a proximal-to-distal direction.
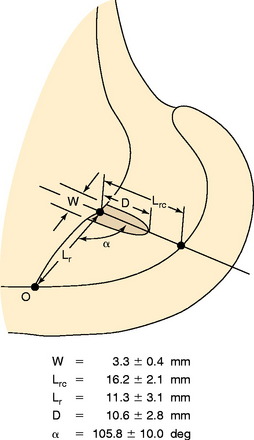
FIGURE 20-29 The geometric variables that describe the location and orientation of the most isometric line.
(From Grood, E. S.; Hefzy, M. S.; Lindenfeld, T. N.: Factors affecting the region of most isometric femoral attachments. Part I: the posterior cruciate ligament. Am J Sports Med 17:197–207, 1989.)
In a second investigation at the authors’ laboratory,76 several attachment sites at the proximal and distal origins located around the circumference of the PCL femoral attachment were studied. The peripheral attachment sites originated on the proximal border of the PCL (anterior, middle, posterior, and posterior oblique) and on the distal border (anterior, middle, and posterior). The limbs were loaded to 100 N and the knees flexed from 0° to 120°. This was one of the first studies to examine PCL fiber length changes at knee flexion angles greater than 90°. The changes in tibiofemoral length for the seven selected peripheral attachment points are shown in Figure 20-31. These data confirm that proximal PCL fibers lengthen with knee extension, whereas distal fibers lengthen with knee flexion. In Figure 20-32, the flexion angles in which the fiber length elongations were the least (within 5% of the maximum length and, therefore, the functional zone) are graphed for each attachment point. The data as a whole show a progressive loading of fibers from distal to proximal within the PCL attachment with progressive knee flexion. There is a smaller effect proceeding from anterior to posterior. These data contradict the description of PCL fiber function that divides the PCL into an anterolateral bundle (which is tight in flexion) and a posteromedial bundle (which is tight in flexion).
The surgeon has the option of placing a PCL graft strand into different regions of the PCL femoral attachment site that determines the functional range of the graft with knee flexion and the knee flexion position to tension the graft. This was investigated in a third cadaveric study in the authors’ laboratory in 12 cadaver knees (Fig. 20-33).45 The one-strand and two-strand reconstructions were attached in three different locations within the PCL femoral footprint (Figs. 20-34 to 20-36). A bone–patellar tendon–bone (B-PT-B) graft was used to provide secure fixation and prevent slippage in the fixture apparatus. The shallow edge of the graft was placed 2 mm from the articular cartilage margin. The center of the deep proximal strand was within 1 to 2 mm of the proximal edge of the PCL attachment. Within the femoral tunnels, the graft orientation was chosen to place the fibers on either the shallow or the distal side of the tunnel, with the bone placed along the deep side. A central tibial tunnel was chosen. A 50-N posterior force was applied to the tibia for the single-strand construct, and a 100-N force was applied to the two-strand PCL reconstructions. The tension in the graft strand was adjusted to restore posterior translation to within ± 1 mm of the intact knee. The graft tensioning was performed at 90° flexion for all grafts except the proximal (deep) graft, which was tensioned at 30° knee flexion. This was done to remain consistent with prior data on the increased tibiofemoral separation distance of a graft placed at this location.
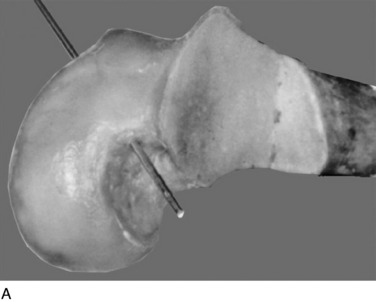
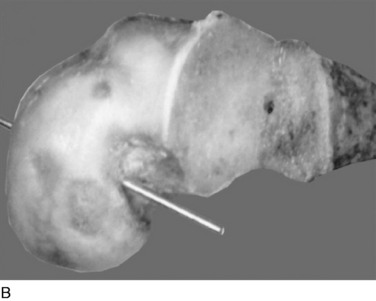
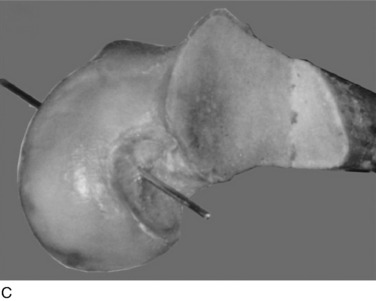
FIGURE 20-35 A–C, The oblique view of the three femoral tunnel positions.
(From Mannor, D. A.; Shearn, J. T.; Grood, E. S.; et al.: Two-bundle posterior cruciate ligament reconstruction. An in vitro analysis of graft placement and tension. Am J Sports Med 28:833–845, 2000.)
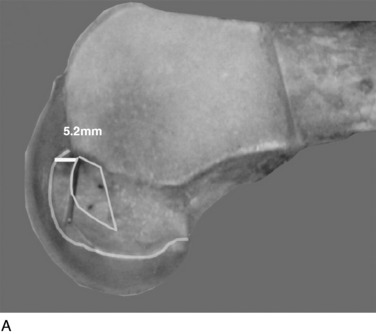
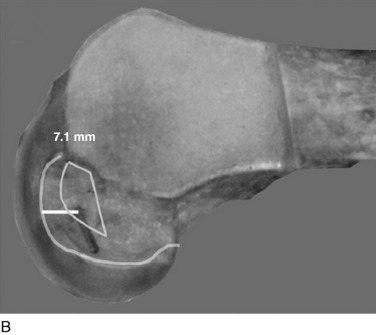
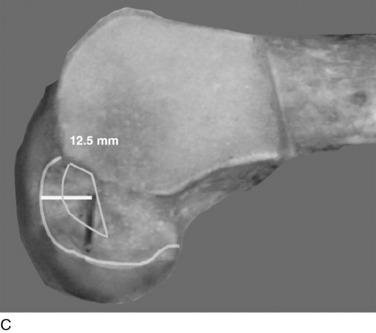
FIGURE 20-36 A–C, The sagittal view of the three femoral tunnel positions.
(From Mannor, D. A.; Shearn, J. T.; Grood, E. S.; et al.: Two-bundle posterior cruciate ligament reconstruction. An in vitro analysis of graft placement and tension. Am J Sports Med 28:833–845, 2000.)
In Figure 20-37, the increase in graft tension with knee flexion is shown along with the posterior translation limit. The one-strand graft restored the knee joint to a normal posterior translation limit, with a more than doubling of graft tension with knee flexion (maximum, 118 ± 15 N; P < .001). In Figure 20-38, the one-strand reconstruction placed at the distal and middle (S2) position shows a similar tension relationship with knee flexion and also restored knee posterior translation limits to normal. In contrast, the single-graft strand placed into a deep and more proximal position (Fig. 20-39) showed less overall graft tensile loading and an abnormal increase in posterior translation with knee flexion. The difference in the behavior from the two shallow grafts was significant (P < .001). The more proximal graft position and graft tensioning did not restore normal posterior translation limits and, therefore, is not a position recommended for PCL reconstruction.
The more complex behavior of a two-strand PCL reconstruction is shown in Figure 20-40 where the two strands were both placed into the more distal locations and tensioned at 90°. Both strands shared the applied load, as shown. In contrast, a two-strand construct in which one strand was placed anterior and a second was placed more proximal resulted in a marked difference in load-sharing, with a reciprocal loading relationship found between strands (Fig. 20-41). In both situations, posterior translation limits were restored to normal. Similar results were reported by Carson and associates9 who conducted an in vitro study of cadaveric knees using a two-bundle (two femoral tunnels) and tibial inlay graft and measured forces in the anterolateral and posteromedial bundles. A reciprocal loading pattern was reported when the anterolateral bundle was placed anterior and the posteromedial bundle was placed posterior to the center of femoral rotation. These authors recommended that tensioning and fixation of the anterolateral and posteromedial bundles be performed at 90° and 0°, respectively.
To further investigate these findings, a fourth cadaveric study was conducted on 19 lower limbs to determine the tension that develops in various two-strand PCL graft constructs.80 The test conditions are shown in Figure 20-42 for the graft strand configurations. A one-strand PCL reconstruction was performed and the measured graft tension compared with three different two-strand reconstructions. The location of the single- and two-strand graft configurations are shown in Figure 20-43. The one-strand graft was placed in the anterior one third and middle of the PCL femoral attachment. The two-strand PCL graft reconstructions placed one strand in the anterior femoral region (AD2) and the second graft strand in either the distal, the middle, or the proximal third of the PCL attachment. The reconstructions were tensioned (with a custom-designed instrumented spatial linkage) to restore posterior translation to ± 1 mm of the intact knee (90° flexion, 100 N posterior load). The two-strand graft configurations were all tensioned to share load, with the exception that the proximal strand (MP) was tensioned to carry 20% of the load and the anterior strand was tensioned to carry 80%. The tension in each graft strand was directly measured during knee motion using strain-gauge load-cells following a methodology previously described.80
The relationship between graft strand placement within the PCL femoral attachment and the tension and function of the graft strand are shown in Table 20-4. In Figure 20-44, the tension in the single-strand PCL graft is shown to nearly double with knee flexion. Under a 100-N posterior load, the graft tension reached over 200 N. This doubling of the tensile load as a multiple of the applied posterior load has been shown in numerous experiments conducted in laboratory studies. With the knee at 90° of flexion, the PCL is elevated approximately 60° from the sagittal AP plane. At this flexion position, the PCL resists nearly all (95%) of the applied posterior force. In this study, the graft tensions required to restore normal posterior translation limits ranged from 230 to 300 N.
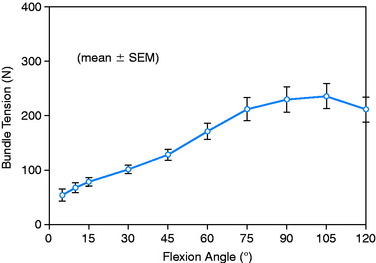
FIGURE 20-44 Strand tension for the one-strand reconstruction. SEM, standard error of the mean.
(From Shearn, J. T.; Grood, E. S.; Noyes, F. R.; Levy, M. S.: Two-bundle posterior cruciate ligament reconstruction: how bundle tension depends on femoral placement. J Bone Joint Surg Am 86:1262–1270, 2004.)
The graft strand tensions for the two-strand PCL reconstructions are shown in Figures 20-45 to 20-47. The average tension and the peak tension decreased for the two-strand reconstructions in which the second graft strand was placed in either a distal or a middle position and able to share the posterior resisting load (Table 20-5). This represented the most ideal arrangement for the two graft strands, particularly when the second graft strand was placed in the middle third of the PCL attachment. In contrast, placement of the second graft strand in a more proximal location resulted in a reciprocal loading behavior between both graft strands, with higher loads placed on the anterior graft strand in comparison with those in the other two-strand configurations.
The data in this experiment support the conclusions of prior studies previously described that small changes in graft position in a proximal-to-distal direction within the PCL attachment have a pronounced effect on graft tension behavior. The hypothesis of this study is that load-sharing between two-strand PCL reconstructions is more ideal in terms of graft function in the long term in preventing posterior tibial subluxation with increasing knee flexion. In addition, the lower graft strand loads in a load-sharing configuration would be more protective against deleterious graft elongation that would be expected to occur under higher loads. It should be noted that under the conditions of the experiment, all of the two-strand reconstructions restored the posterior translation limit to within ± 1 mm of the intact knee, whereas the single-strand reconstruction resulted in a slight overconstraint of 2 mm (which occurred at the 30° flexion position; Fig. 20-48).
Petersen and associates,68 in a cadaveric study, examined the effect of the femoral tunnel position of a two-bundle PCL reconstruction using a robotic testing system. These authors reported a significant effect of the femoral tunnel position in fiber tension behaviors. An anterior position of the two tunnels (anterolateral 7 mm, posteromedial 4 mm from femoral articular cartilage) was recommended. A more posterior (deep) placement of the tunnels was not recommended. One problem should be noted in that the PCL reconstructions (80 N tension at 90° knee flexion) resulted in a residual posterior tibial translation (7–10 mm at 90° knee flexion). It is preferred that biomechanical experiments tension grafts until the abnormal posterior translation is eliminated and not to an arbitrary graft tension, as in this study.
Markolf and colleagues47 conducted a cadaveric study in which one- and two-strand PCL reconstructions were performed to determine whether the second graft strand placed in a posterior position (two placements, center and proximal PCL footprint) provided an advantage in terms of tensile load-sharing between graft fibers. The posteriorly placed grafts provided a resistance to posterior tibial translation, developed tension in the low knee flexion range, and developed greater than the normal PCL forces measured prior to PCL reconstructions. One problem not explained in the study is that under 100 N posterior tibial load, the PCL would be expected (as the primary restraint in an oblique position) to develop close to or greater than the 100-N applied posterior forces. In the experimental results, the PCL only developed forces of approximately 30 to 50 N in the 20° to 40° flexion range, which is considerably lower than would be expected. Therefore, it is difficult to interpret the data because the PCL and graft forces were less than expected.
Race and Amis70 examined the separate functions of the anterolateral (aPCL) and posteromedial (pPCL) PCL bundles under loaded conditions in cadaveric knees. The results showed that approaching knee extension, ligamentous restraints other than the PCL provide a significant contribution to resisting posterior tibial translation (Fig. 20-49). With increasing knee flexion, the aPCL and the pPCL provide a posterior resisting force. These results question the traditional concept that the pPCL provides a posterior resistance close to knee extensions because the pPCL is aligned nearly perpendicular to a posterior loading direction. Further, with knee flexion, the pPCL is oriented in a more advantageous position because it is less vertical in terms of resisting posterior tibial displacement. These data support those from the senior author,45 as already discussed. In addition, surgical reconstruction of the knee with significant posterior instability at low flexion angles implies damaged medial and posterolateral structures described in the bumper model analogy in Chapter 3, The Scientific Basis for Examination and Classification of Knee Ligament Injuries.
Race and Amis72 demonstrated in a cadaveric study that a single anterolateral bundle reconstruction alone would not prevent abnormal posterior tibial translation at high flexion angles. Markolf and coworkers48 studied in cadavers the effect of a single PCL graft placed in three tunnels (anterior, central, and posterior) within the PCL femoral footprint. The authors reported that if a single graft is used, a central location provided the best match for the PCL. The anterolateral and posteromedial tunnel positions did not control posterior tibial translation and underwent potentially deleterious high forces. A problem in the experimental design was that the posteromedial graft was tensioned at 90° knee flexion (its shortest position) and, therefore, underwent very high tensile loads as the knee approached full extension. It is important at surgery to determine the length change of the graft construct (tibiofemoral separation distance during knee flexion-extension), as is discussed later.
Wiley and associates96 studied in cadavers the effect of single- and double-bundle PCL grafts and reported that the double-bundle reconstruction had statistically lower posterior tibial translation. Both graft constructs were placed into the distal two thirds, or more shallow, PCL femoral attachment (avoiding a posterior or deep graft placement) and both grafts were tensioned at 90° knee flexion. The single-bundle PCL reconstruction was placed more proximally in the PCL attachment.
The primary conclusion of these studies is that most of the graft constructs are capable of restoring the posterior translation limit to normal after PCL sectioning. However, the constructs do so by inducing high tensile forces in the graft that may be deleterious and result in graft elongation and failure. To the extent that the in vitro data apply to in vivo graft function, the surgeon may select a position of the second graft strand that induces either load-sharing or reciprocal loading. Placement of the two graft strands in the middle region avoids the large tibiofemoral separations and resultant graft tensions that affect grafts placed closer to the periphery of the PCL attachment. These findings contradict those of other studies that recommend that the second graft strand be placed distal or proximal in relation to the PCL attachment.70,71 Even though the surgeon may select a more ideal graft placement in regard to load-sharing between graft strands, or the more ideal placement of a single graft strand, the data show that due to the diameter of the graft, there will be asymmetrical graft loading with portions of the graft at the outside diameter undergoing different elongation and tension than other portions. This makes it difficult for any graft strand to reproduce the complex microgeometry of the PCL in which different length collagen fibers are brought into the loading sequence as knee flexion occurs.
CYCLICAL FATIGUE TESTING OF ONE- AND TWO-STRAND PCL GRAFT CONSTRUCTS
Several potential mechanisms exist for failure of a PCL graft after implantation, and it is important to design operative techniques and a postoperative rehabilitation program that diminish high tensile forces when possible. Because the PCL is the primary restraint to posterior tibial subluxation, any activities that involve high knee flexion or hamstring activation (descending stairs, walking down a ramp, squatting) may induce deleterious graft tensile forces.4,10,51,66 In the early postoperative period, before graft maturation occurs and an adequate quadriceps muscle force production is able to counteract these forces, graft elongation or failure might occur. All of the muscles that cross the knee joint are involved in increasing joint contact forces by allowing normal knee joint geometry to decrease high tibial shear forces (see Chapter 23, Rehabilitation of Posterior Cruciate Ligament and Posterolateral Reconstructive Procedures).10,49,57 Improper graft tensioning (at the knee position of the shortest length) will produce high tensile forces as the graft elongates with knee flexion or extension. Abrasion of collagen strands against bone tunnels is a known cause of graft thinning and rupture.6,53,79
The cyclical fatigue of a PCL graft after implantation has been reported in only a few studies.6,53,79 The failure mechanism of cyclical fatigue involves the nonuniform distribution of loads. For example, a single graft strand placed into the PCL femoral attachment with knee flexion-extension would subject the individual graft fibers to different tensions and lengths. The native PCL has different fiber lengths that are brought into the loading profile; however, a single-diameter graft of a fixed length does not function as a native PCL fiber. This means that individual fibers would, at certain periods, be carrying the majority of the applied load, potentially resulting in an overload situation and graft fiber elongation. The biomechanical studies already presented show that a proximal-to-distal change in graft position of 2 to 3 mm results in significant changes in graft tension-length relationships. As well, a PCL graft strand may be subjected to angulation (bending) or torsional forces at the bone insertion site. A native ligament has two fibrocartilagenous zones63 owing to diffuse insertional forces over a wide area and decreased stiffness that prevents stress concentration effects. It is theorized that collagen graft fibers placed into a bone tunnel do not undergo remodeling to restore a normal attachment site,7,95 and therefore, stress concentration effects are always present, particularly at higher in vivo loads when activity is resumed.
The transfer of a B-PT-B construct may be highly beneficial in this regard because there is evidence that the bone-tendon junction does remodel to a normal attachment site.75
Studies of cyclical fatigue testing of PCL grafts after implantation have reported a marked effect of this loading that produced premature graft failure. In three studies, the flexion angle of the graft construct was fixed and a load applied in a cyclical manner to test a one-strand B-PT-B graft.6,53,55 This type of loading is less ideal in terms of understanding graft cyclical fatigue properties because single tensile loading does not reproduce the loading profile that a graft would be subjected to with knee flexion-extension motion. Mehalik56 studied the cyclical behavior of one-strand Achilles tendon grafts in knees cycled to 90° of flexion and reported an increase in posterior translation of approximately 1.7 mm after 1000 cycles (posterior load, 50 N; flexion limit, 105°).
Markolf and colleagues53 performed a cyclical loading study of a B-PT-B allograft using a tibial tunnel and a tibial inlay technique. After 2000 cycles, the graft length of the inlay and tunnel methods increased 5.9 mm and 9.8 mm, respectively (load level, 50–300 N). The authors concluded that grafts undergo thinning and increase in length with both fixation techniques. There was greater thinning and degradative effects of the angulated grafts in tibial tunnels than with the tibial inlay grafts. For example, 10 of 31 grafts (32%) failed at the acute angle of tibial attachment prior to 2000 cycles, whereas all of the 31 tibial inlay graft constructs survived the testing procedure. The data suggest that a 10-mm wide B-PT-B graft replacement for PCL reconstruction, with either a tunnel or an inlay technique, might result in unacceptable graft elongation under the loading profile. However, the relationship of the cyclical loading behavior to the other mechanical properties of the graft was not studied, and it is possible that significant disuse and age effects resulted in increased elongation or premature failure.
Bergfeld and coworkers6 compared a tibial tunnel graft technique with a tibial inlay technique using a 10-mm central third B-PT-B graft in cadavers. Both reconstructions restored posterior translation limits to within 2 mm (150 N loading). A tensile load on the PCL graft at the femoral site of 89 N was used. After 72 cycles (from 0°–90°), the “laxity” of both reconstructions increased as a result of graft stretching, with thinning of the graft found at the tibial tunnel exit but not in the tibial inlay group. The authors acknowledged that the conditions of tensioning may have overconstrained knees in the inlay group.
In a study from the authors’ laboratory,79 the cyclical behavior of a PCL B-PT-B graft was reported using cadaveric knee joints cycled from 5° to 120° under 100 N posterior loading. The conditions of the experiment were already described and four graft constructs used are shown in Figure 20-42. The behavior of a normal PCL under cyclical loading is shown in Figure 20-50, where at 2048 cycles, the increase in posterior translation under loading was less than 1 mm. The return of 2.5 mm of posterior translation for the four PCL reconstruction techniques is shown in Table 20-6. All of the graft configurations showed a marked sensitivity to cyclical loading. The return of 7.5 mm of posterior translation was also low in terms of the numbers of cycles. Only the two-strand construct with one anterior graft strand and a second strand placed in the middle configuration showed a two- to threefold increase in the number of cycles to graft failure (Fig. 20-51). Even so, this number of cycles to failure would be reached in the first 2 weeks of rehabilitation unless appropriate measures were taken to limit flexion-extension cycles or the maximum of 120°. The results further showed that 76% of the grafts failed at or adjacent to the femoral attachment region (19 of 25 grafts), with 20% (5 of 25 grafts) in the mid-substance, and 4% (1 of 25 grafts) at the tibial attachment (P < .001).
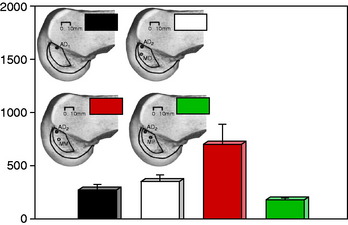
FIGURE 20-51 Cycle number corresponding to a 7.5-mm posterior translation increase.
(From Shearn, J. T.; Grood, E. S.; Noyes, F. R.; Levy, M. S.: One- and two-strand posterior cruciate ligament reconstructions: cyclic fatigue testing. J Orthop Res 23:958–963, 2005.)
Hiraga and colleagues35 reported the results of cyclical loading tests on four types of PCL reconstruction in cadaveric knees. The knee joints were cycled between 0 and 100 N for 1000 cycles. Increases in posterior tibial translation were attributed to slippage of the graft and/or permanent elongation of the graft construct. The knees were positioned at 90° of flexion, and the cyclical loading involved repetitive AP displacements. This is in contrast to the study by Shearn and coworkers79 in which the knees were cycled between 0° and 120° of knee flexion. It is important that differences in cyclical loading techniques be understood, because the cyclical loading under both posterior load and knee flexion-extension is more detrimental in terms of inducing graft elongation or failure than unidirectional loading. Hiraga and colleagues35 reported that no grafts failed under the reduced loading of 100 N; however, there were increases in posterior tibial translation after only 100 cycles in all reconstruction groups. The greatest increase was found in semitendinosus-gracilis (STG) grafts that were fixated with an EndoButton (Smith & Nephew, Naples, FL), which was then an STG graft fixed with an interference screw and an Endopearl device (Linvatec, Largo, FL). There was no difference between B-PT-B tibial tunnel and B-PT-B tibial inlay reconstructions, with increases in residual posterior translation of approximately 3 mm reported for both tunnel and inlay grafts.
1 Ahmad C.S., Cohen Z.A., Levine W.N., et al. Codominance of the individual posterior cruciate ligament bundles. An analysis of bundle lengths and orientation. Am J Sports Med. 2003;31:221-225.
2 Apsingi S., Nguyen T., Bull A.M., et al. Control of laxity in knees with combined posterior cruciate ligament and posterolateral corner deficiency: comparison of single-bundle versus double-bundle posterior cruciate ligament reconstruction combined with modified Larson posterolateral corner reconstruction. Am J Sports Med. 2008;36:487-494.
3 Arms S.W., Pope M.H., Johnson R.J., et al. The biomechanics of anterior cruciate ligament rehabilitation and reconstruction. Am J Sports Med. 1984;12:8-18.
4 Berchuck M., Andriacchi T.P., Bach B.R., Reider B. Gait adaptations by patients who have a deficient anterior cruciate ligament. J Bone Joint Surg Am. 1990;72:871-877.
5 Bergfeld J.A., McAllister D.R., Parker R.D., et al. The effects of tibial rotation on posterior translation in knees in which the posterior cruciate ligament has been cut. J Bone Joint Surg Am. 2001;83:1339-1343.
6 Bergfeld J.A., McAllister D.R., Parker R.D., et al. A biomechanical comparison of posterior cruciate ligament reconstruction techniques. Am J Sports Med. 2001;29:129-136.
7 Blickenstaff K.R., Grana W.A., Egle D. Analysis of a semitendinosus autograft in a rabbit model. Am J Sports Med. 1997;25:554-559.
8 Butler D.L., Noyes F.R., Grood E.S. Ligamentous restraints to anterior-posterior drawer in the human knee. A biomechanical study. J Bone Joint Surg Am. 1980;62:259-270.
9 Carson E.W., Deng X.H., Allen A., et al. Evaluation of in situ graft forces of a 2-bundle tibial inlay posterior cruciate ligament reconstruction at various flexion angles. Arthroscopy. 2007;23:488-495.
10 Castle T.H.Jr., Noyes F.R., Grood E.S. Posterior tibial subluxation of the posterior cruciate–deficient knee. Clin Orthop. 1992;284:193-202.
11 Clancy W.G.Jr., Shelbourne K.D., Zoellner G.B., et al. Treatment of knee joint instability secondary to rupture of the posterior cruciate ligament. Report of a new procedure. J Bone Joint Surg Am. 1983;65:310-322.
12 Covey D.C., Sapega A.A., Marshall R.C. The effects of varied joint motion and loading conditions on posterior cruciate ligament fiber length behavior. Am J Sports Med. 2004;32:1866-1872.
13 Covey D.C., Sapega A.A., Sherman G.M. Testing for isometry during reconstruction of the posterior cruciate ligament. Anatomic and biomechanical considerations. Am J Sports Med. 1996;24:740-746.
14 Daniel D.M., Stone M.L., Barnett P., Sachs R. Use of the quadriceps active test to diagnose posterior cruciate ligament disruption and measure posterior laxity of the knee. J Bone Joint Surg Am. 1988;70:386-391.
15 de Lange A., Van Dijk R., Huiskes R. Three-dimensional experimental assessment of knee ligament length patterns: in vitro. Trans Orthop Res Soc. 1983;8:10.
16 Denham R.A., Bishop R.E. Mechanics of the knee and problems in reconstructive surgery. J Bone Joint Surg Br. 1978;60:345-352.
17 Dickhaut S.C., DeLee J.C. The discoid lateral-meniscus syndrome. J Bone Joint Surg Am. 1982;64:1068-1073.
18 Dorlot J.M., Christel P., Meunier A. The displacement of the bony insertion sites of the anterior cruciate ligament during the flexion of the knee. Trans Orthop Res Soc. 1983;8:328.
19 Edwards R.G., Lafferty J.F., Lange K.O. Ligament strain in the human knee. J Basic Eng. 1970;92:131-136.
20 Anatomie und Mechanik der Gelenke. In: Fick R., von Bardeleben K., editors. Part I: Anatomie der Gelenke; Part II: Allgemeine Gelenk- und Muskelmechanik; Part III: Spezielle Gelenk- und Muskelmechanik. Jena, Germany: Gustav Fischer, 1911.
21 Gill T.J., DeFrate L.E., Wang C., et al. The effect of posterior cruciate ligament reconstruction on patellofemoral contact pressures in the knee joint under simulated muscle loads. Am J Sports Med. 2004;32:109-115.
22 Gollehon D.L., Torzilli P.A., Warren R.F. The role of the posterolateral and cruciate ligaments in the stability of the human knee. A biomechanical study. J Bone Joint Surg Am. 1987;69:233-242.
23 Grood E.S., Hefzy M.S., Lindenfeld T.N. Factors affecting the region of most isometric femoral attachments. Part I: the posterior cruciate ligament. Am J Sports Med. 1989;17:197-207.
24 Grood E.S., Noyes F.R., Butler D.L., Suntay W.J. Ligamentous and capsular restraints preventing straight medial and lateral laxity in intact human cadaver knees. J Bone Joint Surg Am. 1981;63:1257-1269.
25 Grood E.S., Stowers S.F., Noyes F.R. Limits of movement in the human knee. Effect of sectioning the posterior cruciate ligament and posterolateral structures. J Bone Joint Surg Am. 1988;70:88-97.
26 Gupte C.M., Bull A.M., Atkinson H.D., et al. Arthroscopic appearances of the meniscofemoral ligaments: introducing the “meniscal tug test.”. Knee Surg Sports Traumatol Arthrosc. 2006;14:1259-1265.
27 Gupte C.M., Bull A.M., Thomas R.D., Amis A.A. A review of the function and biomechanics of the meniscofemoral ligaments. Arthroscopy. 2003;19:161-171.
28 Gupte C.M., Bull A.M., Thomas R.D., Amis A.A. The meniscofemoral ligaments: secondary restraints to the posterior drawer. Analysis of anteroposterior and rotary laxity in the intact and posterior-cruciate-deficient knee. J Bone Joint Surg Br. 2003;85:765-773.
29 Gupte C.M., Smith A., Jamieson N., et al. Meniscofemoral ligaments—structural and material properties. J Biomech. 2002;35:1623-1629.
30 Gupte C.M., Smith A., McDermott I.D., et al. Meniscofemoral ligaments revisited. Anatomical study, age correlation and clinical implications. J Bone Joint Surg Br. 2002;84:846-851.
31 Haimes J.L., Wroble R.R., Grood E.S., Noyes F.R. Role of the medial structures in the intact and anterior cruciate ligament–deficient knee. Limits of motion in the human knee. Am J Sports Med. 1994;22:402-409.
32 Harner C.D., Vogrin T.M., Hoher J., et al. Biomechanical analysis of a posterior cruciate ligament reconstruction. Deficiency of the posterolateral structures as a cause of graft failure. Am J Sports Med. 2000;28:32-39.
33 Hefzy M.S., Grood E.S. Sensitivity of insertion locations on length patterns of anterior cruciate ligament fibers. J Biomech Eng. 1986;108:73-82.
34 Heller L., Langman J. The menisco-femoral ligaments of the human knee. J Bone Joint Surg Br. 1964;46:307-313.
35 Hiraga Y., Ishibashi Y., Tsuda E., Toh H.T. Biomechanical comparison of posterior cruciate ligament reconstruction techniques using cyclic loading tests. Knee Surg Sports Traumatol Arthrosc. 2006;14:13-19.
36 Hughston J.C., Eilers A.F. The role of the posterior oblique ligament in repairs of acute medial (collateral) ligament tears of the knee. J Bone Joint Surg Am. 1973;55:923-940.
37 Inderster A., Benedetto K.P., Klestil T., et al. Fiber orientation of posterior cruciate ligament: an experimental morphological and functional study, part 2. Clin Anat. 1995;8:315-322.
38 Jamieson N., Bull A.M., Amis A.A. Meniscofemoral ligaments—incidence, anatomy and strength. J Bone Joint Surg Br. 2000;82(suppl II):139.
39 Kennedy J.C., Hawkins R.J., Willis R.B. Strain gauge analysis of knee ligaments. Clin Orthop Relat Res. 1977;129:225-229.
40 LaPrade R.F., Johansen S., Wentorf F.A., et al. An analysis of an anatomical posterolateral knee reconstruction: an in vitro biomechanical study and development of a surgical technique. Am J Sports Med. 2004;32:1405-1414.
41 LaPrade R.F., Tso A., Wentorf F.A. Force measurements on the fibular collateral ligament, popliteofibular ligament, and popliteus tendon to applied loads. Am J Sports Med. 2004;32:1695-1701.
42 Last R.J. Some anatomical details of the knee joint. J Bone Joint Surg Br. 1948;30:683-688.
43 MacDonald P., Miniaci A., Fowler P., et al. A biomechanical analysis of joint contact forces in the posterior cruciate deficient knee. Knee Surg Sports Traumatol Arthrosc. 1996;3:252-255.
44 Makris C.A., Georgoulis A.D., Papageorgiou C.D., et al. Posterior cruciate ligament architecture: evaluation under microsurgical dissection. Arthroscopy. 2000;16:627-632.
45 Mannor D.A., Shearn J.T., Grood E.S., et al. Two-bundle posterior cruciate ligament reconstruction. An in vitro analysis of graft placement and tension. Am J Sports Med. 2000;28:833-845.
46 Markolf K.L., et al. Direct measurement of resultant forces in the anterior cruciate ligament. An in vitro study performed with a new experimental technique. J Bone Joint Surg Am. 1990;72:557-567.
47 Markolf K.L., Feeley B.T., Jackson S.R., McAllister D.R. Biomechanical studies of double-bundle posterior cruciate ligament reconstructions. J Bone Joint Surg Am. 2006;88:1788-1794.
48 Markolf K.L., Feeley B.T., Jackson S.R., McAllister D.R. Where should the femoral tunnel of a posterior cruciate ligament reconstruction be placed to best restore anteroposterior laxity and ligament forces? Am J Sports Med. 2006;34:604-611.
49 Markolf K.L., Graff-Radford A., Amstutz H.C. In vivo knee stability. A quantitative assessment using an instrumented clinical testing apparatus. J Bone Joint Surg Am. 1978;60:664-674.
50 Markolf K.L., Graves B.R., Sigward S.M., et al. Effects of posterolateral reconstructions on external tibial rotation and forces in a posterior cruciate ligament graft. J Bone Joint Surg Am. 2007;89:2351-2358.
51 Markolf K.L., O’Neill G., Jackson S.R., McAllister D.R. Effects of applied quadriceps and hamstrings muscle loads on forces in the anterior and posterior cruciate ligaments. Am J Sports Med. 2004;32:1144-1149.
52 Markolf K.L., Wascher D.C., Finerman G.A. Direct in vitro measurement of forces in the cruciate ligaments. Part II: the effect of section of the posterolateral structures. J Bone Joint Surg Am. 1993;75:387-394.
53 Markolf K.L., Zemanovic J.R., McAllister D.R. Cyclic loading of posterior cruciate ligament replacements fixed with tibial tunnel and tibial inlay methods. J Bone Joint Surg Am. 2002;84:518-524.
54 Maynard M.J., Deng X., Wickiewicz T.L., Warren R.F. The popliteofibular ligament. Rediscovery of a key element in posterolateral stability. Am J Sports Med. 1996;24:311-316.
55 McAllister D.R., Markolf K.L., Oakes D.A., et al. A biomechanical comparison of tibial inlay and tibial tunnel posterior cruciate ligament reconstruction techniques. Graft pretension and knee laxity. Am J Sports Med. 2002;30:312-317.
56 Posterior cruciate ligament reconstruction: an investigation of surgical variables: graft attachment location, knee flexion angle at graft fixation, and postoperative knee mobilization. In: Mehalik J.N., editor. Department of Aerospace Engineering and Engineering Mechanics. Cincinnati, OH: University of Cincinnati, 1992.
57 Morrison J.B. Function of the knee joint in various activities. Biomed Eng. 1969;4:573-580.
58 Morrison J.B. The mechanics of the knee joint in relation to normal walking. J Biomech. 1970;3:51-61.
59 Noyes F.R., Barber-Westin S.D. Posterior cruciate ligament revision reconstruction, part 1: causes of surgical failure in 52 consecutive operations. Am J Sports Med. 2005;33:646-654.
60 Noyes F.R., Barber-Westin S.D. Posterolateral knee reconstruction with an anatomical bone–patellar tendon–bone reconstruction of the fibular collateral ligament. Am J Sports Med. 2007;35:259-273.
61 Noyes F.R., Barber-Westin S.D. Surgical reconstruction of severe chronic posterolateral complex injuries of the knee using allograft tissues. Am J Sports Med. 1995;23:2-12.
62 Noyes F.R., Cummings J.F., Grood E.S., et al. The diagnosis of knee motion limits, subluxations, and ligament injury. Am J Sports Med. 1991;19:163-171.
63 Noyes F.R., DeLucas J.L., Torvik P.J. Biomechanics of anterior cruciate ligament failure: an analysis of strain-rate sensitivity and mechanisms of failure in primates. J Bone Joint Surg Am. 1974;56:236-253.
64 Noyes F.R., Stowers S.F., Grood E.S., et al. Posterior subluxations of the medial and lateral tibiofemoral compartments. An in vitro ligament sectioning study in cadaveric knees. Am J Sports Med. 1993;21:407-414.
65 Ogata K., McCarthy J.A. Measurements of length and tension patterns during reconstruction of the posterior cruciate ligament. Am J Sports Med. 1992;20:351-355.
66 Ohkoshi Y., Yasuda K., Kaneda K., et al. Biomechanical analysis of rehabilitation in the standing position. Am J Sports Med. 1991;19:605-611.
67 Pasque C., Noyes F.R., Gibbons M., et al. The role of the popliteofibular ligament and the tendon of popliteus in providing stability in the human knee. J Bone Joint Surg Br. 2003;85:292-298.
68 Petersen W., Lenschow S., Weimann A., et al. Importance of femoral tunnel placement in double-bundle posterior cruciate ligament reconstruction: biomechanical analysis using a robotic/universal force-moment sensor testing system. Am J Sports Med. 2006;34:456-463.
69 Petersen W.J., Loerch S., Schanz S., et al. The role of the posterior oblique ligament in controlling posterior tibial translation in the posterior cruciate ligament–deficient knee. Am J Sports Med. 2008;36:495-501.
70 Race A., Amis A.A. Loading of the two bundles of the posterior cruciate ligament: an analysis of bundle function in a-P drawer. J Biomech. 1996;29:873-879.
71 Race A., Amis A.A. PCL reconstruction. In vitro biomechanical comparison of “isometric” versus single- and double-bundled “anatomic” grafts. J Bone Joint Surg Br. 1998;80:173-179.
72 Race A., Amis A.A. The mechanical properties of the two bundles of the human posterior cruciate ligament. J Biomech. 1994;27:13-24.
73 Robinson J.R., Bull A.M., Thomas R.R., Amis A.A. The role of the medial collateral ligament and posteromedial capsule in controlling knee laxity. Am J Sports Med. 2006;34:1815-1823.
74 Robinson J.R., Sanchez-Ballester J., Bull A.M., et al. The posteromedial corner revisited. An anatomical description of the passive restraining structures of the medial aspect of the human knee. J Bone Joint Surg Br. 2004;86:674-681.
75 Rodeo S.A., Arnoczky S.P., Torzilli P.A., et al. Tendon-healing in a bone tunnel. A biomechanical and histological study in the dog. J Bone Joint Surg Am. 1993;75:1795-1803.
76 Saddler S.C., Noyes F.R., Grood E.S., et al. Posterior cruciate ligament anatomy and length-tension behavior of PCL surface fibers. Am J Knee Surg. 1996;9:194-199.
77 Sekiya J.K., Haemmerle M.J., Stabile K.J., et al. Biomechanical analysis of a combined double-bundle posterior cruciate ligament and posterolateral corner reconstruction. Am J Sports Med. 2005;33:360-369.
78 Shahane S.A., Ibbotson C., Strachan R., Bickerstaff D.R. The popliteofibular ligament. An anatomical study of the posterolateral corner of the knee. J Bone Joint Surg Br. 1999;81:636-642.
79 Shearn J.T., Grood E.S., Noyes F.R., Levy M.S. One- and two-strand posterior cruciate ligament reconstructions: cyclic fatigue testing. J Orthop Res. 2005;23:958-963.
80 Shearn J.T., Grood E.S., Noyes F.R., Levy M.S. Two-bundle posterior cruciate ligament reconstruction: how bundle tension depends on femoral placement. J Bone Joint Surg Am. 2004;86:1262-1270.
81 Sidles J.A., Larson R.V., Garbini J.L., et al. Ligament length relationship in the moving knee. J Orthop Res. 1988;6:593-610.
82 Sigward S.M., Markolf K.L., Graves B.R., et al. Femoral fixation sites for optimum isometry of posterolateral reconstruction. J Bone Joint Surg Am. 2007;89:2359-2368.
83 Simonian P.T., Sussmann P.S., van Trommel M., et al. Popliteomeniscal fasciculi and lateral meniscal stability. Am J Sports Med. 1997;25:849-853.
84 Skyhar M.J., Warren R.F., Ortiz G.J., et al. The effects of sectioning of the posterior cruciate ligament and the posterolateral complex on the articular contact pressures within the knee. J Bone Joint Surg Am. 1993;75:694-699.
85 Staubli H.-U., Birrer S. The popliteus tendon and its fascicles at the popliteal hiatus: gross anatomy and functional arthroscopic evaluation with and without anterior cruciate ligament deficiency. Arthroscopy. 1990;6:209-220.
86 Sudasna S., Harnsiriwattanagit K. The ligamentous structures of the posterolateral aspect of the knee. Bull Hosp Jt Dis Orthop Inst. 1990;50:35-40.
87 Sugita T., Amis A.A. Anatomic and biomechanical study of the lateral collateral and popliteofibular ligaments. Am J Sports Med. 2001;29:466-472.
88 Trent P.S., Walker P.S., Wolf B. Ligament length patterns, strength, and rotational axes of the knee joint. Clin Orthop Relat Res. 1976;117:263-270.
89 Trus P., Petermann J., Gotzen L. Posterior cruciate ligament (PCL) reconstruction—an in vitro study of isometry. Part I: tests using a string linkage model. Knee Surg Sports Traumatol Arthrosc. 1994;2:100-103.
90 Length measurements on the cruciate ligaments: measuring technique and results. In: Van Dijk R., editor. The Behavior of the Cruciate Ligaments in the Human Knee. Netherlands: Dissertation, University of Nijmegen; 1983:89-126.
91 van Dijk R., Huiskes R., Selvik G. Roentgen stereophotogrammetric methods for the evaluation of the three dimensional kinematic behaviour and cruciate ligament length patterns of the human knee joint. J Biomech. 1979;12:727-731.
92 Veltri D.M., Deng X.-H., Torzilli P.A., et al. The role of the popliteofibular ligament in stability of the human knee. A biomechanical study. Am J Sports Med. 1996;24:19-27.
93 Veltri D.M., Deng X.H., Torzilli P.A., et al. The role of the cruciate and posterolateral ligaments in stability of the knee. A biomechanical study. Am J Sports Med. 1995;23:436-443.
94 Weber W., Weber E. Mechanik der menschlichen. Part II. Gehwerkzeuge, editor. Ueber das kniegelenk. Gottingen, Germany; 1836:161-202.
95 Weiler A., Peine R., Pashmineh-Azar A., et al. Tendon healing in a bone tunnel. Part I: biomechanical results after biodegradable interference fit fixation in a model of anterior cruciate ligament reconstruction in sheep. Arthroscopy. 2002;18:113-123.
96 Wiley W.B., Askew M.J., Melby A.3rd, Noe D.A. Kinematics of the posterior cruciate ligament/posterolateral corner–injured knee after reconstruction by single- and double-bundle intra-articular grafts. Am J Sports Med. 2006;34:741-748.
97 Wroble R.R., Grood E.S., Cummings J.S., et al. The role of the lateral extra-articular restraints in the anterior cruciate ligament–deficient knee. Am J Sports Med. 1993;21:257-262. discussion 263