29 Extracorporeal Devices and Related Technologies
On May 6, 1953, Gibbon closed an atrial septal defect with the use of a heart-lung machine, the culmination of more than 20 years of his own research.1,2 By the early 1950s, Gibbon had completed an extensive series of animal experiments with the heart-lung machine with survival rates of greater than 90%. However, his first attempt in human patients was not successful. On his second attempt, the patient’s circulation was supported for less than 20 minutes while the atrial septal defect was repaired. According to Dr. Bernard J. Miller, “Near the termination of the operation, the machine suddenly shut down—reason being, clotting of the blood on the oxygenator took place, and the automatic arterial control sensed the sudden fall in the pool at the bottom and shut the entire machine down.”3,4 However, the patient survived and was discharged from the hospital in 9 days. Gibbon’s five subsequent procedures at Jefferson Hospital were not successful and he abandoned the use of ECC. However, his one successful case served to inspire others, including John Kirklin at The Mayo Clinic, C. Walton Lillihei at the University of Minnesota, and Denis Melrose at Hammersmith Hospital in London, to continue the further development of ECC and cardiopulmonary bypass (CPB) in the laboratory and ultimately in the clinical arena. The accomplishments of these early pioneers in cardiac surgery have been described as being “the boldest and most successful feats of man’s mind.”4
Since the 1950s, CPB has undergone a dramatic metamorphosis from a lifesaving, yet life-threatening, technique to an event practiced nearly 1,000,000 times a year throughout the world. It is uncommon in today’s medical environment to encounter such an invasive procedure, with such significant risk and inherent morbidity, being practiced as routine. The goal of all techniques of CPB always has been to design an integrated system that could provide nutritive solutions with appropriate hemodynamic driving force to maintain whole-body homeostasis, without causing inherent injury. A recent randomized clinical trial, the Randomized Off-pump or On BYpass (ROOBY) trial, involving 2203 elective or urgent coronary artery bypass grafting (CABG) patients randomized to either off- or on-pump surgery is a testament to the efficacy and safety of CPB as currently practiced. At 1 year, the on-pump group had significantly better composite outcomes (death, myocardial infarction, or repeat revascularization) than the off-pump group (9.9% vs. 7.4%; P = 0.04). The overall rate of graft patency was lower in the off-pump group than in the on-pump group as well (82.6% vs. 87.8%; P < 0.01).5
Mechanical devices

Blood Pumps
All extracorporeal flow occurs through processes that incorporate a transfer of energy from mechanical forces to a perfusate, and, ultimately, to the tissue. Methods of achieving this transfer of energy include gravitational and mechanical forces, or a combination of both. It is through the transfer of energy from an electrical power source to the motor of a pumping mechanism and on to the fluid (blood) that causes tissue perfusion.4,6 Most extracorporeal pumps fall into one of the following categories: positive displacement (PD), centrifugal or constrained vortex (CP), passive filling, pneumatic and electrical pulsation, and axial flow (the latter pumps are used primarily as cardiac assist or replacement devices),7–9 and are described in Chapter 27.

Positive Displacement Pumps
The PD pump operates by occluding tubing between a stationary raceway and rotating roller(s) or occluder(s) (Figure 29-1). The pumping mechanism is also referred to as the pump head, and the tubing that traverses the raceway is referred to as the pump header. PD pumps were first proposed for use in cardiovascular medicine in the 1930s by Gibbon.2 In 1935, an adaptation to the PD pump was described that included tube bushings at the head of the raceway on both inlet and outlet locations to prevent tubing creepage around the roller head.9 Melrose10 later modified the pump to include a grooved raceway, which further reduced tubing shimmy. Both of these adaptations were important in reducing the mobility of tubing during the operation of the pump, which decreased the potential for tubing rupture in the pump head. In a PD pump, fluid is displaced in a progressive fashion from suction to discharge, with the capacity of the displacement dependent both on the volume of the tubing occluded by the rollers and on the number of revolutions per minute (rpm) of the roller. All PD roller pumps (RPs) use the volume in the pump header, which is referred to as a flow constant, and is specific to each size of tubing referred to by the internal diameter of tubing, for calculating the flow of the pump. This is displayed on a digital readout and is referred to as the output (flow) of the pump. It is measured in liters per minute. Although many types of RPs have been used for CPB, the most common PD pump in use today is the twin-RP.
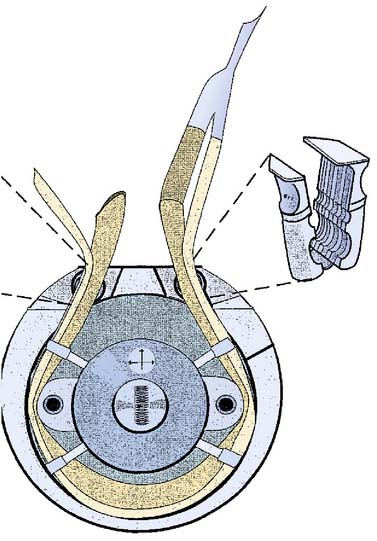
Figure 29-1 Stockert S-3 Twin Roller Pump diagram.
A positive displacement pump with a stationary raceway and rotating twin roller pumps.
(Courtesy of The Sorin Group, Arvada CO.)
There are currently at least five manufacturers of PD pumps used in ECC, with each device consisting of minor variations of the twin-RP design (Box 29-1). A modern heart-lung machine consists of between four and five of these RPs positioned on a base console (Figures 29-2 and 29-3). Most machines are modular in design, permitting the rapid change-out of a defective unit in the case of single-pump failure. It is standard practice of perfusionists to rotate the pumps along the base console in different positions so that mechanical wear is distributed evenly while maintaining equitable time utilization. Each pump is independently controlled by a rheostat that functions to regulate the rpm of the rollers. Each pump is calibrated according to specific flow constants that are calculated from the internal diameter of tubing, as well as the tubing length, placed in the pump raceway. Periodically, PD pumps are calibrated by performing a timed collection of pumped fluid to verify that after proper calibration the pump delivers the volume indicated on the pump flow display. The internal diameters for ECC tubing ranges between 1/8 and 5/8 inch/min. For this reason, a single console can be used to perfuse a wide range of patients whose size may vary from a few kilograms to several hundred. This is accomplished simply by changing the raceway tubing and the shims that hold the tubing in place. It is important to note that the larger the internal diameter of the tubing, the lower the rpm necessary to achieve a desired pump flow. This is especially important because there is a positive correlation between red blood cell (RBC) hemolysis and the rpm of the pump rotation. The magnitude of hemolysis is related to both the time and exposure of the blood to shear forces generated by the pump. A region of high pressure and shear force is created at the leading edge of the roller where the tubing is compressed, which is followed by a period of negative pressure as the tubing expands behind the roller. This momentary negative pressure under certain conditions may induce the cavitation of air dissolved in the solution. A further related concern is particulate emboli that may be generated by microfragmentation, so-called spallation, of the inner surface of the tubing where the roller contacts the tubing and where the fold at the edges of the tubing occurs.11 Studies of tubing wear over time have shown that polyvinylchloride fragments generated from RPs are numerous, frequently less than 20 μm in diameter, and begin to occur during the first hour of use.12 However, the majority of the hemolysis generated during a routine CPB procedure is not related to the occlusiveness of the arterial pump head but rather by the air-surface interface interaction occurring with the use of suction and “vent” lines components of the circuit.13 An underocclusive arterial pump head will result in retrograde flow. This, in turn, will require increased rpm to ensure adequate forward flow, which increases hemolysis.14 Overocclusive adjustment of the RP results in both hemolysis of RBCs and spallation (particulate fragmentation from the inner walls of tubing) that continues with PD pumps.15 Kurusz11 identified the erosive and fatiguing action of the RP as a major source for generating tubing particles in CPB circuits.
BOX 29-1 Roller Pumps

Centrifugal Pumps
The second type of extracorporeal pump is a resistance-dependent pump termed a centrifugal (CP), or constrained vortex pump.16–18 The CP conducts fluid movement by the addition of kinetic energy to a fluid through the forced centrifugal rotation of an impeller or cone in a constrained housing (Box 29-2). The greatest force, highest energy, is found at a point most distal to the center axis of rotation (Figures. 29-4 to 29-6). CPs operate as pressure-sensitive pumps, with blood flow directly related to downstream resistance. Blood flow is, therefore, related to both the rpm of the cones or impellers and the total resistance. This represents an important safety feature in coupling blood flow with resistance. During unexpected increases in resistance, the total energy transfer from the CP to blood will not generate forces sufficient to cause arterial line separation. However, when downstream occlusion occurs, either through increases in afterload or through the placement of line clamps, the fluid in the pump head will be heated because of hydrodynamic processes in the magnetic coupling. This increase in temperature could result in increased blood trauma and coagulation defects.18
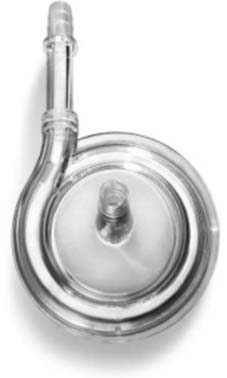
Figure 29-4 Rotaflow Centrifugal Pump disposable with low-friction one-point bearing (sapphire ball and PE calotte).
(Courtesy of Maquet Cardiovascular, Wayne, NJ.)
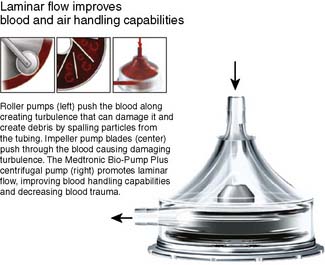
Figure 29-6 Medtronic Bio-Pump Plus Centrifugal Pump.
(Courtesy of Medtronic Cardiovascular, Eden Prarie, MN.)
The acceptance of these devices in routine CPB has increased tremendously since first being introduced into clinical practice in 1969,19 and it is the pump of choice during emergency bypass procedures. The CP also has been used as a ventricular assist device (VAD) because of its inherent safety features and pressure sensitivity, as well as relatively low cost. Although these pumps have been used extensively off-label for VADs, none of the CPs has received U.S. Food and Drug Administration clearance for use for systemic circulatory support for more than 6 hours. CP pumps have been used extensively off-label as VADs or in extracorporeal membrane oxygenation (ECMO) circuits. The afterload and preload sensitivity of these pumps make them particularly amenable for use for ECMO in the treatment of reversible respiratory dysfunction and postcardiotomy dysfunction.20 The Levitronix CentriMag Blood Pumping System (Levitronix, Waltham, MA) recently developed a pump with a novel magnetically levitated bearingless motor technology designed to minimize friction and heat generation in the blood path (Figure 29-7), which reduces stasis and minimizes blood trauma. The Centrimag is approved for use for up to 6 hours of support and is undergoing further investigation for prolonged use for patients with heart failure (HF). The Centrimag also recently received approval by the FDA for use as a right ventricular support device, for use up to 14 days to treat patients with right-heart failure—the first approval of this class of pump for use beyond 6 hours. In a recent in vitro study, Guan et al21 compared mechanical performance characteristics of the Centrimag pump with a conventional CP, the Rotaflow Centrifugal Pump (Maquet, Wayne, NJ) and reported better mechanical performance characteristics with the rotaflow pump in terms of higher shutoff flow rate, maximal flow, and propensity for retrograde flow.21 These findings deserve further study given the magnitude of cost for the Centrimag pump system disposable components (Centrimag costs 20 to 30 times more than other CP disposable components).
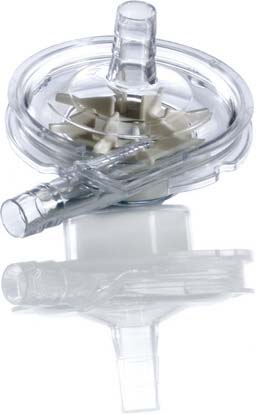
Figure 29-7 CentriMag Blood Pump.
Thoratec CentriMag magnetically levitated bearingless blood pump.
(Reprinted with permission from Thoratec Corporation.)
When gross air is introduced into the CP, as in emptying of the venous reservoir, the pump head will deprime, stopping forward flow, which reduces the risk for gas embolization. However, when small quantities of air are aspirated into the pump head, these bubbles will coalesce and be passed into the outlet stream of fluid movement, and potentially into the patient. Although the CP has been described as exerting less trauma to the cellular elements of blood,22 variability in individual pump hemolytic potential has been reported.23,24 Tamari et al25 have reported that the degree of hemolysis in CP is related to the hemodynamic conditions under which the pump is operated, with lower flows and higher pressure resulting in more hemolysis than similarly operated RPs. There have been reports of thrombus formation when these pumps are used with low anticoagulation or for prolonged periods.26 Later designs possess fins and channels that prevent these areas of stasis. Improved designs have addressed issues of stasis, heat generation, and bearing wear. One contemporary design has minimal contact area for the cone and the outer housing and incorporates a series of magnets to suspend the moving rotor within the pump housing.27 Additional advantages of CP over PD RPs include reduced mechanical trauma to extracorporeal tubing and the generation of high-volume output with moderate pressure development. A potential complication associated with nonocclusive-type pumps involves retrograde flow through the aortic cannula when the pressure in the central aorta exceeds that generated by the pump.28 This may occur during times of power disruption or pump failure when there is an increased risk for drawing air into the arterial line via purse-string sutures placed to secure the arterial cannula (see Safety Mechanisms for Extracorporeal Flow section later in this chapter). Other uses of CPs include supported CPB in high-risk angioplasty patients, left-heart bypass (LHB) during repair of descending thoracic aortic aneurysms or dissections, and veno-venous bypass during hepatic transplantation. Use of CPs to assist venous return for minimally invasive cardiac surgery is described as kinetic-assisted venous return.29,30
Currently, six manufacturers produce CPs for extracorporeal use: Biomedicus (Biomedicus-Medtronics, Minneapolis, MN), Delphin (3M Health Care, Ann Arbor, MI), Revolution Pump (The Sorin Group, Arvanda, CO), Capiox-SP (Terumo Medical Corporation, Somerset, NJ), Rotoflow Maquet (Wayne, NJ), and the Centrimag Pump. The operational characteristics are similar among the various systems in which the internal smooth cones or vaned impellers are connected to a central magnet (isolated from contact with blood by encasement in a polycarbonate housing), which couples with the console, where electromagnetic forces are produced. The centrifugal console usually is placed in the arterial pump head position on the heart-lung machine, replacing the main drive. All of the consoles currently available include their own battery backup systems in the event of power failure and a manually operated motor in case of drive motor or console failure. The Revolution pump is equipped with an electronic tubing clamp that may be programmed to deploy automatically if low, zero, or retrograde flow is sensed; if the level sensor in the venous reservoir senses a low level; if a high arterial line pressure is sensed; or if the air detector on the arterial line senses air in the circuit (Figure 29-8). This is an especially important feature when using these machines to transfer patients on ventricular assistance or during the conduct of emergency bypass. Each manufacturer markets disposable software that must be purchased in conjunction with the pump.
A number of investigators have conducted in vitro studies comparing CPs and RPs in terms of blood handling during short-term and long-term use. Oku et al,31 Jakob et al,32 Englehardt et al,33 and Hoerr et al34 reported less hemolysis with the CP when tested in vitro. Kress et al35 showed no difference between the two pump types in a rabbit ECMO model. Tamari et al36 examined hemolysis under various flow and pressure conditions in an in vitro model using porcine blood and concluded that the hemolysis index was related to the duration of blood exposure to shear, the ratio of pump pressure difference between the inflow and outflow, and the flow rate of the pump. From this work they provided guidelines related to pump selection based on the pressure/flow ratio likely to occur in a given application. Rawn et al37 compared an underocclusive RP with a CP and found a significantly higher index of hemolysis in the CP (3.38 to 14.65 vs. 29.58 g/100 L pumped). In a randomized trial, Salo et al38 examined inflammatory response mediators in 16 CABG patients with CPB times of less than 2 hours. These mediators included interleukin-1 beta (IL-1β), IL-2, IL-6, phospholipase A2, endotoxin, fibronectin, and serum C Group II phospholipase A2. These researchers found no differences in the levels of these inflammatory markers immediately post-CPB and at 24 hours after surgery. Other randomized clinical trials have been conducted to compare emboli generation, neurocognitive outcome, blood trauma, and patient charges. Wheeldon et al39 conducted a randomized, controlled trial in 16 patients, in which the only difference in equipment and technique was the type of pump used, and found significantly fewer microemboli, less complement activation, and better preservation of platelet count. Parault and Conrad40 reported a similar significant improvement in platelet preservation in a retrospective review of 785 cases and further reported that the differences were more profound in patients older than 70 years with CPB times of longer than 2 hours. Klein et al41 conducted a randomized, prospective clinical study in 1000 adult cardiac patients comparing RPs with the Biomedicus CP (Medtronic, Eden Prairie, MN), using risk stratification methodology, and reported clinical benefits to the CP including blood loss, renal function, and neurologic outcomes, but no significant difference in mortality. Ashraf et al42 examined S100 beta levels relative to pump type in a randomized, controlled trial that included 32 patients who had CABG and found no significant difference in S100 beta levels between the groups at 2 and 24 hours after bypass. Dickinson et al43 did a retrospective review of 102 patients examining length of stay, total patient charges, reimbursement, mortality, and major complications but could not identify a single difference. A more recent randomized control trial by Scott et al44 subjected 103 patients to a battery of 6 standardized tests and found a trend toward fewer abnormal tests in the CP group; however, it failed to reach statistical significance. DeBois et al45 conducted a trial in 200 elective CABG surgery patients who were randomized to either an RP or CP and found similar patient characteristics including platelet counts, hematocrit, transfusion rate, and mortality; however, they observed differences favoring the CP with regard to weight gain, length of stay, and net hospital financial balance. Alamanni et al46 evaluated the prevalence of major neurologic complications in 3,438 consecutive patients and found the occurrence of injury to be associated with age and a history of a previous neurologic event. The authors further reported that use of the CP provided a risk reduction for the considered events ranging from 23% to 84%. Babin-Ebell47 et al conducted a randomized trial of CABG patients and found a significant reduction in tissue factor in the group supported with a CP; however, this did not translate into a measurable reduction in thrombin formation or other apparent clinical benefit. Baufreton et al48 examined cytokine production (tumor necrosis factor-α, IL-6, IL-8) and circulating adhesion molecules (soluble endothelial-leukocyte adhesion molecule-1 and intercellular adhesion molecule-1) in a randomized, controlled trial of 29 CABG patients. They reported greater SC5b-9 and elastase levels in the CP group, suggesting more favorable performance from the RP with regard to complement and neutrophil activation.
Although nearly all of the randomized trials show significant benefit to systems designed with CPs, it is difficult to separate the improved performance conferred from other characteristics, such as lower prime volume, surface coating, more limited surface area, and reduced air-to-blood contact. Current research would suggest that CPs produce less blood damage; however, this improvement may be masked by blood trauma and inflammation related to contact activation of the blood related to cardiotomy suction, the introduction of gaseous and particulate emboli, and related factors. According to the recently published Guidelines on Perioperative Blood Transfusion and Blood Conservation in Cardiac Surgery, jointly endorsed by the Society of Thoracic Surgeons and the Society of Cardiovascular Anesthesiologists, “It is not unreasonable to select a CP rather than a RP but more so for safety reasons rather than blood conservation” (American Heart Association/American College of Cardiology Class IIb level of evidence B).49 In 2000, approximately 50% of the cardiac centers in the United States routinely used CPs.50

Safety Mechanisms for Extracorporeal Flow
Some of the most recent advances in pump design have been a result of a heightened awareness of increasing safety associated with complex operating systems. The PD pumps are pressure independent, which means they will continue to pump regardless of downstream resistance. In a CPB circuit, the summation of resistances against which a pump must function includes the total tubing length, the oxygenator, the heat exchanger, the arterial line filter, the cannula, and the patient’s systemic vascular resistance (SVR). Additional factors that influence SVR include the viscosity of the perfusate, related to the total formed element concentration, which primarily is dependent on the formed elements of blood and the temperature of the solution. According to Poiseuille’s law, the greatest resistance to flow is created at the arterial cannula, where the change in the caliber of the tubing lumen declines the most. Perfusionists routinely monitor the summation of all resistances and record this value as the arterial line, or system, pressure. This always will be greater than the pressure measured at the distal end of the circuit terminating at the cannula tip because the pressure drop across each component in the series circuit will be subtracted from the summation of resistance (resistors) in the entire circuit. Bypass circuitry and components have been designed to incorporate minimal pressure drops; therefore, in routine adult perfusion, the resistance becomes a function of the patient’s SVR and the pump flow rate. Establishing a normal value for arterial line resistance is difficult, although normal limits range between 100 and 350 mm Hg. Any acute change in resistance, such as unexpected clamping or kinking of the arterial line, results in an abrupt increase in arterial line pressure, which can lead to catastrophic line separation or circuit fracture anywhere on the high-pressure side of the circuit. A life-threatening event could occur on the initiation of CPB if the tip of the arterial cannula lodges against the wall of the aorta, undermining the intima of the vessel. Under these conditions, aortic dissection can occur as the vessel intima separates from the media, directing blood flow into a newly created false lumen. This dissection can extend throughout the entire length of the aorta. For this reason, perfusionists routinely check the line pressure after cannulation before the onset of CPB to ensure the presence of a pulsatile waveform, indicating proper cannula placement in the central lumen of the aorta. Either the absence of pulsatility or an extremely high line pressure (> 400 mm Hg when CPB is initiated) should immediately be investigated (see Chapter 28).
All heart-lung machines include a microprocessor-controlled safety interface with their pump consoles. These systems monitor and control pump function and serve as the primary mechanical safety control system for regulating extracorporeal flow. Pressure limits are set by the perfusionist and are determined by patient characteristics and the type of intervention performed. These units consist of early-warning alarms that alert the user to abrupt changes in pressure and will automatically turn off a pump when preset limits are exceeded. These safety devices have been used in both the main arterial pump and the cardioplegia pump; the latter become more important with the utilization of retrograde cardioplegia administration into the coronary sinus.51,52 Currently, the incorporation of a safety monitor for negative pressure sensing, located on the inflow side of the arterial pump head and during pulsatile perfusion when intermittent occlusion is created, still is lacking.
Electrical failure in the operating room can be especially catastrophic in the conduct of ECC when the native heart and lungs are unable to function. When such an event occurs during CPB, it is imperative that instantaneous actions be instituted to minimize the risk for whole-body hypoperfusion. The perfusionist should be mindful of the power limitations of the electrical outlet used in the cardiac operating room and also be aware of the location of the circuit breaker panel for the room and the specific number of the breaker in the panel for the outlet used for the heart-lung machine and other support equipment. Methods to ensure the safe conduct of CPB involve the incorporation of an emergency power source in the extracorporeal circuit that provides a secondary power source in the event of electrical interruption. Electrical failure during CPB was reported by 42.3% of respondents in a survey on perfusion accidents.53 Although hospitals are equipped with emergency generators for such events, their availability may be limited to certain electrical circuits within the operating suite. Furthermore, these emergency power systems require a brief interruption in power before a generator or backup source of power is initiated. Most heart-lung machines are equipped with uninterrupted backup power, sometimes referred to as the “Uninterrupted Power Source” (UPS), whereby there is a seamless transfer from the wall power source to an internal battery within the pump should the wall power fail. Thus, with this system, there is no loss of flow from the pumps that could result in retrograde flow and entrainment of air or disruption of settings and timers. Cases of primary power failure with concurrent emergency backup failure also have been reported.54 As a tertiary fallback measure, emergency hand cranks for CPB pumps are standard features in extracorporeal circuitry, which enable pump operation in the event of total power failure and when emergency systems fail to operate. However, care should be taken to ensure that the direction of blood flow is ascertained because hand cranking in the reverse direction of fluid flow could result in serious patient injury related to exsanguination and the entrainment of air around purse-string sutures at cannulation sites. The Retroguard valve (Quest Medical Incorporated, Allen, TX), a mechanical circuit component to prevent retrograde flow in the arterial outflow of the circuit, is available to prevent retrograde flow in the arterial line and possible entrainment of air into the circuit and into the patient’s arterial circulation. It is composed of a simple duck-bill valve that adds minimal resistance to forward flow in the circuit and will close when downstream pressure is greater (Figure 29-9).
Although the chance of infusing massive air boluses to patients has been reduced dramatically since the early days of CPB,53 this remains a serious potential event during surgery (Box 29-3). Cannulation of the heart with aortic and venting catheters has been identified as the primary cause for air embolization during ECC.55 Methods of air-bubble detection have improved tremendously, and the sensitivity for detecting small amounts of air has increased in modern heart-lung machines.56 Ultrasonic and capacitance air-detection systems, used for both level sensing and air detection in arterial and cardioplegia circuit lines, represent dramatic improvements over less sensitive (photoelectric) methods.57 However, lacking in clinical practice are effective, reliable level-sensing devices that alert the perfusionist to rapid changes in venous reservoir levels, especially during utilization of collapsible venous reservoir systems. Both air-bubble detection and level-sensing devices should be safety techniques used as standards of care in all extracorporeal circuits.
Extracorporeal circuitry

Blood Gas Exchange Devices
The ECC of blood incorporating total heart-lung bypass could not be accomplished were it not for the development of devices that could replace the function of the lungs in pulmonary gas exchange. The technology of pumps to replace the mechanical action of the heart was developed well before their incorporation in ECC. Therefore, the limiting factor hindering the progression of CPB was the development of an artificial lung, or blood gas exchange device (BGED), commonly referred to as a membrane oxygenator (Box 29-4). The term membrane denotes the separation of blood and gas phases by a semipermeable barrier, whereas oxygenator refers to the change in oxygen partial pressure that occurs by the arterialization of venous blood. However, “oxygenator” is a misrepresentation of the functional ability of these systems to perform ventilatory control of carbon dioxide. Numerous engineering challenges hindered the development of BGEDs, but two of the most pressing were the design of high-capacity units for gas exchange with low rates of bioreactivity. The latter requirement, also termed biocompatibility, was imperative to reduce both RBC trauma and activation of the formed elements of blood.
BOX 29-4 Membrane Oxygenators
In the 1940s, the first dialyzer membranes were made of cellulose acetate, and although intended for use in dialysis, they also had gas exchange characteristics.1 In the 1950s, several membrane materials (including polyethylene and ethyl cellulose) were used in a flat sheet or plate configuration. At the same time, rotating disk oxygenators were introduced whereby gas exchange was accomplished by spreading venous blood in a thin film over a rotating disk, which was exposed to an oxygen-rich environment. In the 1960s, the first disposable membrane oxygenators were introduced and were made primarily of silicone rubber in either a plate or spiral wound design. Silicone offered the distinct advantage of separating both the blood and gas phases, facilitating gas exchange through a semipermeable barrier by diffusion. Teflon was introduced in the 1970s as a membrane material, together with microporous polypropylene, which first appeared in Travenol membrane devices. Today, the majority of commercially available oxygenators are made of polypropylene in either a pleated or folded configuration, or as capillary hollow fibers (Figure 29-10). In the United States, manufacturers develop oxygenators that meet federal regulatory guidelines for performance and biocompatibility. Those devices meeting these requirements are “cleared,” approved for use for up to 6 hours of CPB, and represent the majority of oxygenators. Currently, there is only one oxygenator that utilizes silicone membranes that is approved for long-term support such as that occurring for ECMO. However, the “off-label” use of more durable, lower prime, hollow-fiber technology membrane oxygenators and newer polymethylpentane fiber oxygenators is widely reported in the literature.
Historically, oxygenators were divided into two broad classes based on the method of gas exchange: bubble and membrane systems. Bubble-type devices have been shown to denature plasma proteins, increase RBC fragility,58 activate platelets,59 and generate substantial gaseous microemboli (GME).60–62 For these reasons, they are no longer used in most countries and are infrequently encountered in all but a few remaining places throughout the world. Bubbler systems use a direct gas-blood interface, with gas exchange occurring by the dispersion of gas, either 100% oxygen or a mixture of oxygen and carbon dioxide (carbogen), through a column of desaturated blood. Bubble devices are made of two separate compartments: an oxygenating column and a defoaming chamber. The dispersion of gas in a bubbler occurs through a sparger plate, where a thin film of blood comes in direct contact with gas. This direct blood-gas interface results in the production of foam, where gas exchange occurs. Coalescence of the foam is achieved in the defoaming chamber both through the presence of surface tension–reducing substances and by filtration. Gas exchange is affected by several factors, including the quantity of gas and the size of bubbles produced in the gas sparger.63 Small bubbles are extremely efficient at oxygen exchange but poor at carbon dioxide exchange, whereas large bubbles are poor in oxygen but good in carbon dioxide exchange.
Membrane oxygenators are made of three distinct compartments: gas, blood, and water (see Figure 29-10). The latter phase is also termed the heat exchange compartment and is used for temperature control. Gas and blood are partitioned into separate compartments with either a limited or absent gas-blood interface. Microporous membrane oxygenators initially have a blood-gas interface that becomes diminished only after the inner blood contact surface has been exposed to plasma; and a protein layer is deposited, acting as a diffusible barrier to gas exchange. The most common material in use today in membrane oxygenators is microporous polypropylene, which has excellent capacity for gas exchange and good biocompatibility. Membrane devices made of silicone materials transfer gas directly by diffusion across the semipermeable membrane and effectively never have a blood–gas interface.64 Despite the improvements made to extracorporeal devices over the past several decades, once blood is exposed to synthetic surfaces, hematologic changes result. Initially, complement is activated mainly through alternative pathways, resulting in the liberation of toxic mediators such as C3a and C5a.65,66 Both platelets and leukocytes that elicit a complex series of inflammatory and hemostatic reactions that ultimately increase the risk for postoperative complications are activated.63
A multitude of factors must be considered in the design of a membrane BGED, including total surface area, blood film thickness, diffusion residence time, gas diffusion rate, blood flow rate, blood flow geometrics, and gas flow characteristics. The most influential factors that affect blood trauma in an oxygenator are related to how blood traverses the device and are termed shear stress and stasis.67 Design characteristics that minimize these effects by optimizing flow pattern geometry through extracorporeal devices have been generated through mathematical models termed computational fluid dynamics.68 Two of the most important considerations in designing a membrane device are determining the type of membrane material and the handling of water vapor produced in the gas phase of the device. This water vapor would be synonymous with pulmonary exudate and, when excessive, mimics pulmonary edema associated with permeability changes of the alveolar capillary membrane. Another important membrane feature is how blood flows through the membrane. As fluid moves through a conduit, laminae are established, with the highest velocity of flow achieved in the center of the tube. At the same time, the outermost layers, nearest the walls of the conduit, effectively have no velocity because of the drag coefficient of the inside surface. This occurs in both the gas phase and the blood phase of membrane oxygenators. The laminar effect can be disrupted by several techniques that produce a “secondary flow,” facilitating increased gas exchange.69 In hollow-fiber membrane oxygenators, incorporating blood flow outside of the fibers, mixing is achieved by winding of the fibers, creating a crossing pattern, increasing blood exposure to the membrane surface. Laminar flow is reduced in hollow-fiber oxygenators with blood flow through the fibers by the expansion and contraction of the capillaries via the movement of blood through them, gently disrupting the boundary layers.
The oxygenator represents the largest source of nonendothelialized surface area in the extracorporeal circuit, ranging in size between 0.5 and 2.5 M2. As a consequence, it is imperative that the device is meticulously primed to remove all residual air before establishing CPB. Oxygenators have been shown to possess different abilities to remove gaseous emboli that vary according to the physical CPB conditions including temperature and pressure decline.70–73 In an effort to reduce surface exposure and prime volume, several membrane oxygenators are now manufactured that either possess integrated arterial line filters (FX Oxygenator Line; Terumo Cardiovascular, Ann Arbor, MI; Figure 29-11) or systems in which the arterial line filter is sequenced in the oxygenator (Synthesis; Sorin Biomedical, Arvada, CO). Some studies suggest that these devices may result in a reduction in gaseous micromboli.74,75
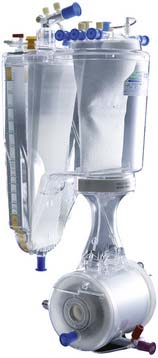
Figure 29-11 Hard-shell venous reservoir with integral cardiotomy and membrane oxygenator (Terumo RX15).
(Courtesy of Terumo Cardiovascular, Ann Arbor, MI)
Numerous studies have identified the occurrence of GME during cardiac surgery with CPB.76 Weitkemper et al77 have shown that currently used microporous membrane oxygenators have widely variable characteristics related to how they handle gas. Furthermore, the design characteristics in some cases cause partial removal of GME, as well as a change in size and numbers of microbubbles. Dickinson et al78 conducted an in vitro analysis that showed significant air-handling differences between the oxygenators from four different manufacturers. They demonstrated how a sonar-based system, the embolus Detection and Classification System (EDAC; Lunar Technology, Blacksburg, VA), could be used to evaluate perfusion systems with regard to their ability to handle gas entrained in the circuit.
A new nonporous membrane surface composed of poly-(4-methyl-1-pentene) (PMP) fibers has shown improved diffusion compared with the conventional polypropylene (PPL) hollow fibers. PPL affords improved durability and biocompatibility when used for long-term support79,80 and for routine CPB.81 Although oxygen and carbon dioxide gas exchange are comparable between polypropylene hollow fibers and the PMP nonporous fibers, it is important to note that the transfer of volatile anesthetic agents is not the same. Wiesenack et al82 demonstrated that the PMP fibers allow only minimal transfer of isoflurane compared with the currently used PPL microporous hollow-fiber oxygenators. During long-term support, it is not uncommon for PMP oxygenators to develop breaches in the surface that lead to plasma leaks after 40 to 90 hours of use, whereas the PPL fibers tend to be more robust and are not prone to plasma leaks, and continue to transfer oxygen and carbon dioxide for many days.

Venous and Cardiotomy Reservoirs
There are two general categories for venous reservoirs: open and closed systems (Box 29-5). Open systems have a hard polycarbonate venous reservoir and usually incorporate a cardiotomy reservoir and defoaming compartment (see Figure 29-11). Closed systems are collapsible polyvinylchloride bags that have a minimal surface area and often a thin single-layer screen filter, and they require a separate external cardiotomy reservoir for cardiotomy suction (Figure 29-12). Filters and defoaming compartments in the venous reservoir and air-trapping ports located at the highest level of the blood flow path within the oxygenator are areas designed to allow passive removal of air. Studies that have examined the air-handling capabilities of oxygenators have shown that all of the currently available oxygenators do not sufficiently remove GME when challenged with air in the inflow.77,78 The use of an open system offers several distinct advantages. Unlike collapsible reservoirs, it is not necessary to actively aspirate air, which may be entrained in the venous line during CPB. The large buoyant air migrates to the top of the reservoir and escapes through strategically placed vents on the reservoir cover. An additional benefit of the use of “open” hard-shell reservoir systems incorporates the capability of vacuum-assisted venous drainage, although alternative methods have been implemented augmenting venous drainage to closed “bag” systems using CPs or creative vacuum applications applied to the venous line to enhance natural gravity drainage. Furthermore, a number of studies have reported a greater incidence of GME caused by air entrained in the venous line and furthermore that vacuum-assisted venous drainage further increases GME counts.81,83–87 Wilcox has raised concern that vacuum-assisted venous drainage has been used clinically without any significant redesign of the components of the CPB circuit to improve the gas handling performance in negative pressure conditions.86 The prime volume may be reduced slightly because the integration of the venous reservoir with the cardiotomy eliminates connecting circuitry and may permit a smaller bore venous line with use of vacuum-assisted venous drainage. With open systems, the circulating blood is exposed to a larger and more complex surface that contains defoaming sponges and antifoam agents.
BOX 29-5 Venous Reservoirs
Open Systems
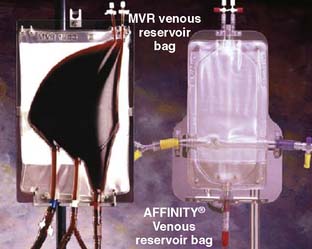
Figure 29-12 Example of a closed system.
Oxygenator with a collapsable polyvinyl chloride venous reservoir.
(Courtesy of Medtronic Cardiovascular, Minneapolis, MN.)
Air can traverse the oxygenator into the arterial outflow of the CPB circuit and into the patient’s arterial circulation, including the cerebral circulation, producing contact activation of the vascular endothelium or obstruction at the microcapillary level. Thousands of GMEs can be introduced into the patient’s arterial circulation with these circuits if air becomes continuously entrained into the venous inflow, a condition that could not be tolerated with a collapsible reservoir. Recently, several randomized clinical trials have found superior clinical outcomes with a system equipped with a closed reservoir and a centrifugal arterial pump.88,89 Schonberger et al90 prospectively studied differences in inflammatory and coagulation activation of blood in CABG patients treated with open and closed reservoir systems. Levels of complement 3a, thromboxane B2, fibrin degradation products, and elastase were significantly greater in open reservoir patients. Furthermore, the largest (P < 0.001) amount of shed blood loss, greatest (P < 0.05) need for colloid-crystalloid infusion, and largest (not significant) need for donor blood (0.8 ± 0.4 vs. 0.2 ± 0.2 units of packed cells) were observed in the patient supported with open reservoir systems.
Aldea et al91 conducted a randomized, controlled trial to evaluate the effects of cardiotomy suction in CABG patients. Use of cardiotomy suction resulted in significant increases in thrombin, neutrophil, and platelet activation, as well as the release of neuron-specific enolase, after CPB. The authors suggested that limiting increases in these markers would be best accomplished by eliminating cardiotomy suction and routinely using heparin-bonded circuits whenever possible.

Miniaturized Cardiopulmonary Bypass
The principal drawbacks to the conventional CPB circuit include activation of the systemic inflammatory response, aberration in coagulation function, CPB-related gaseous embolism, and excessive hemodilution requiring blood transfusions. A principal design approach to overcome some of these problems has been the introduction of “miniaturized CPB circuits” that reduce the blood–foreign surface contact, blood-air interface, and hemodilution (Figures 29-13 to 29-15). The currently available “mini” systems consist of either an adaptation of standard CPB components or the introduction of new devices by manufacturers that have a striking resemblance to existing devices.92–126 The manufacturers’ “mini” systems all use a single CP to provide kinetic venous drainage and arterial blood propulsion. All have eliminated or isolated the venous reservoir to reduce blood foreign surface contact, and all eliminated the introduction of activated blood from a cardiotomy suction system. Field shed blood is recaptured and washed by an autotransfuser before reintroduction to the “mini” systems. Many of the systems have incorporated multisite bubble detection and innovative air removal systems to automatically remove and isolate micro and macro air entrained in the CPB circuit.
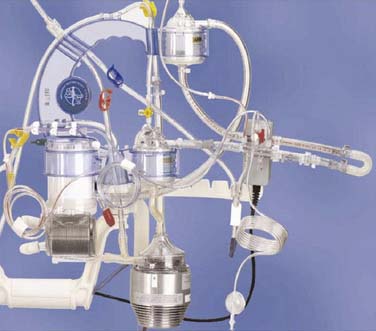
Figure 29-13 Medtronic resting heart closed “mini-bypass” system.
(Courtesy of Medtronic Cardiopulmonary, Minneapolis, MN.)
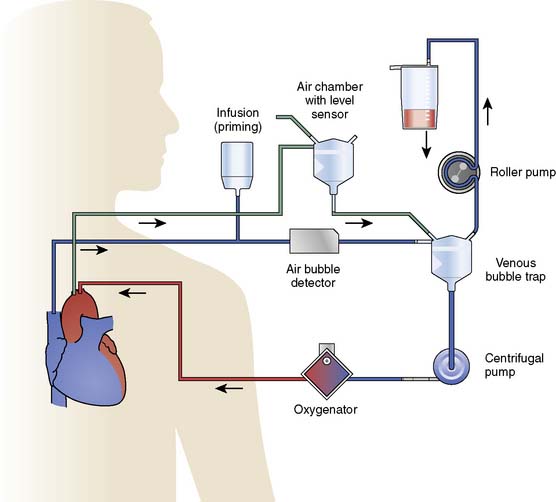
Figure 29-15 Maquet Minimal ExtraCorporeal Circulation (MECC).
(Courtesy of Maquet Cardiovascular, Wayne, NJ.)
A recent meta-analysis of randomized, controlled trials conducted by Zangrillo127 et al sought to determine whether the use of miniaturized CPB translates into decreased morbidity including blood transfusion, neurologic events, and blood loss in patients having cardiac surgery. Sixteen trials met inclusion criteria, 1619 patients (803 to miniaturized CPB and 816 undergoing standard cardiac surgery). Miniaturized CPB proved to be beneficial in terms of decreased transfusion rate and decreased cardiac and neurologic injury. These finding are summarized in Figure 29-16. Current use of such systems is limited. Further studies are necessary to substantiate the benefit of such systems and will likely increase the adoption of this new technology.

Heat Exchangers
Patients who are exposed to ECC will become hypothermic in the absence of an external source of heat to regulate body temperature. Most CPB systems use some form of heat exchanger in the circuit to warm and/or cool the patient’s blood. The majority of oxygenators contain integral heat exchangers that blood passes through before undergoing gas exchange (see Figure 29-10). Heat exchangers may be absent from circuits used for ventricular assist or certain types of LHB. However, in either of these scenarios, external warming blankets and ambient room temperature are controlled to restrict declines in patient temperature. Heat exchangers can be made from a variety of materials, although the most often used are aluminum (anodized or silicone-coated anodized), stainless steel, and polypropylene. Stainless steel is the most durable and chemically inert of all commercially used heat exchangers.
Other potential risks of heat exchangers are associated with the type of material used for construction. Because stainless steel is relatively expensive, aluminum has been used most often as the material for heat exchangers. Aluminum, however, has a high toxicity in humans; when blood levels exceed 100 mg/L, careful patient monitoring is imperative and levels greater than 200 mg/L are toxic.128 Aluminum oxide concretions were found recently in organs of neonatal patients having undergone ECMO; these concretions most likely formed from aluminum leached from the anodized aluminum heat exchangers used in the circuit.129
Heat exchanger performance standards were established in the American Association of Medical Instruments draft report in 1982.130 Performance testing is conducted by the simultaneous measurement of three temperatures: blood inlet temperature, blood outlet temperature, and water inlet temperature. Heat exchanger performance is reflected through a coefficient for heat transfer calculated by the following equation (where a coefficient of 1 is equal to 100% efficiency):

Arterial Line Filters
Arterial line filters significantly reduce the load of gaseous and particulate emboli and should be used in CPB circuits131,132 (Figure 29-17). Some studies suggest that 20-μm screen filtration is superior to 40-μm filtration in the reduction of cerebral embolic counts.132 A dose-response relation between GME and subtle neurologic injury has been reported, and some studies have demonstrated a protective effect of arterial line filtration on neurologic outcomes133–135 Whitaker et al’s136 clinical trial showed that the use of a leukocyte-depleting arterial line filter reduced cerebral embolic count and demonstrated a trend (not statistically significant) toward improved postoperative psychometric test scores. The GME separation performance of 10 different arterial line filters in clinical use was recently evaluated.137 All were found to be moderately effective, and rated pore size did not predict performance. A systematic review of the data related to arterial line filtration reported that the level of evidence supporting this practice was high (Class I level of evidence A).138 Filter design has been of two principal types: microporous screen filters and depth filters composed of dense fiber material packed in a polycarbonate housing patented by Swank. Screen filters are the predominant type in current use. Screen filters trap particulate and gaseous emboli that are of larger diameter than their effective pore size. The filter material is accordion pleated to provide a larger surface area within a lower prime housing. Two contemporary filter designs consist of a larger flat screen surface that is located concentrically around the oxygenator fiber bundle. The Terumo F series filter incorporates the screen material concentrically surrounding the fiber bundle. This design reduces CPB circuit prime volume because the filter media is incorporated into the oxygenator housing, eliminating the prime volume of the separate arterial filter housing.139 Preston et al140 found that the F05 series oxygenator released more emboli than a similar model oxygenator used in combination with a separate 32-μm arterial filter, although the difference was not statistically significant. Sorin Group has incorporated a concentric filter design that surrounds the fiber bundle. The screen forms an envelope around the fiber bundle. This design does not effectively reduce prime; however, the larger housing provides an effective bubble trap (Box 29-6).

Cannulae and Tubing
The major devices of CPB are those that replace the systems from which the heart-lung machine has derived its name. However, as with most technologic advances, it is the combination of all component parts that function in toto to ensure success. Besides the pump and oxygenator, a seamless array of tubing is required to connect the patient to the heart-lung machine. Monitoring lines are necessary not only to ensure patient hemodynamic management but also to assess the proper function of the pump. Manufacturers of tubing and circuit packs can attest to the large number of variations in combinations and configurations of circuit assemblies requested by different institutions, as well as by individual clinicians within the same institution. The following discussion of an “ideal” tubing circuit has been generated from the experiences of the authors and may differ somewhat among cardiac centers (Figure 29-18).
The majority of cardiac procedures using CPB are performed with venous cannulation through the right atrium (RA) and arterial return into the ascending aorta. A multitude of cannulae are available for all types of cardiac surgery, which may reflect the developmental philosophy that if a vessel could conceivably be perfused or drained, then a cannula could be made to facilitate entry. The key principles of cannulae design include minimizing turbulence, reducing cannulae exit velocity, and avoiding areas of stagnant flow so that blood trauma and thrombus formation are minimal (Figure 29-19). In the past, cannulae were constructed of stainless steel or tapered polyvinylchloride. Subsequently, thin-walled stainless steel was used to increase effective orifice diameter and reduce cannulae pressure drop across the cannulae. Currently, most cannulae are fabricated from polyvinylchloride with composite polycarbonate thin wall tips. The ends of the cannulae are formed to permit easy vascular entry while maintaining maximum lumen (caliber) size. According to Poiseuille mechanics, the greatest resistance, measured as pressure drop in a circuit, is going to be found at the smallest opening for fluid flow and has an inverse exponential relation to the fourth power of the radius of the lumen. Therefore, to reduce pressure drops across the circuit, cannulae are selected to facilitate the greatest flow with the least injury to the vessel because of mechanical abrasion. Several arterial cannulae designs have incorporated multiple openings and dispersion tips to reduce velocity at the tip of the cannulae and reduce the likelihood of disruption of atheromatous debris from the intimal surface of the aorta.141,142 Most cannulae have a wire reinforcement body to prevent kinking when the cannula is curved to accommodate placement in the surgical field and to maintain cannula rigidity. Although cannulation of the ascending aorta is preferred for most procedures, femoral arterial cannulation often is selected for reoperations or minimally invasive surgical procedures (Figure 29-20). The axillary or subclavian artery often is selected for arterial return for patients with severe atherosclerosis of the ascending aorta. This site offers the advantage of providing antegrade flow to the arch vessels, protection of the arm and hand, and avoidance of inadvertent cannulation of the false lumen in cases in which type A aortic dissection has occurred. The axillary artery is accessed through a subclavicular incision.143 Although the vessel may be cannulated directly with a long thin-walled 7- or 8-mm cannula, the preferred technique is to sew a 10- or 12-mm Dacron graft to the right axillary artery and insert a 20- to 24-Fr cannula into the graft. This technique provides uninterrupted flow to the right arm and hand. Transapical aortic cannulation through a 1-cm incision on the anterior wall of the left ventricle also has been described for patients with type A dissection. A 7.0-mm aortic cannula may be placed into the left ventricle and advanced across the aortic valve (Box 29-7).
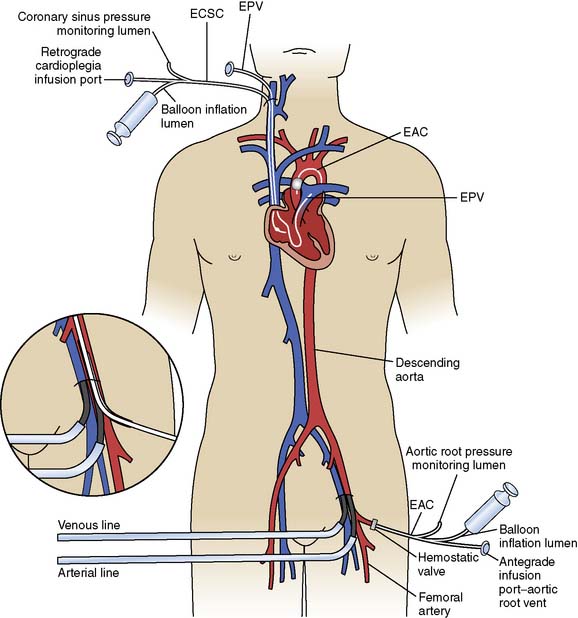
Figure 29-20 Diagram of cannulation used for minimally invasive surgery.
EAC, endoaortic occlusion balloon; ECSC, endocoronary sinus catheter; EPV, endopulmonary vent.
(From Toomasian JM, Williams DL, Colvin SB, et al: Perfusion during coronary and mitral valve surgery utilizing minimally invasive port-access technology. J Extra Corpor Technol 29:66–72, 1997.)
BOX 29-7 Arterial Cannulae
Although both silicone (Silastic) and polyurethane tubing have been used in extracorporeal circuits, plasticized polyvinylchloride is exclusively used in CPB circuits. Plasticizers impart flexibility into tubing and comprise as much as 40% of the polymer. The most commonly used plasticizer for medical-grade tubing is di(2-ethylhexyl) phthalate (DEHP). There is growing concern about the migration (leaching) of DEHP from tubing into the blood because DEHP has been shown to cause inflammation and is potentially a carcinogen and a toxic agent.144,145 Numerous studies since the 1970s have shown that DEHP and its metabolites are present in blood products146–149 and tissues,150,151 as well as intravenous solutions152 and pharmaceuticals.153 Recently, the release properties of various plasticized polyvinylchloride tubing exposed to electrolyte solutions for up to 28 days were evaluated. Tubing formulations with one dioctyl adipate (DOA) stored in 0.9% sodium chloride solution had significantly less leaching than either DEHP plasticizer or tri(2-ethylhexyl) trimellitate (TOTM).
Blood flows out of the RA cannula and into the venous reservoir when CPB is initiated. The venous line connects the cannula to the venous reservoir. Mixed venous oxygen saturation is measured by optical or chemical fluorescence by flow through cells placed in the venous line. A stopcock is placed in the venous line to facilitate the delivery of medications and for venous sampling. Blood then enters the venous reservoir, which serves as a volume chamber for mixing and acts as a safety feature, providing additional response time to the perfusionist. Venous reservoirs come in two broad categories: hard shell (see Figure 29-11) and soft shell (see Figure 29-12), which refers to the rigidity of the device and its ability to collapse on itself. Hard-shell reservoirs are open to the atmosphere (open systems) through a ventilation port on top of the reservoir and are superior in handling gross quantities of air that may return through the venous or cardiotomy line. Soft-shell reservoirs are called closed systems and will collapse on themselves with the inadvertent emptying of the reservoir. The venous reservoir also has an inlet line that drains blood from a cardiotomy reservoir.
The oxygenator has two ports on the outflow side by which arterialized blood is accessed: a recirculation port and an arterial outlet port. The recirculation port is used both to provide a safety line for relieving overpressurization and to facilitate easy replacement in the event of device failure. It also is used as an exit port of arterialized blood for sanguineous cardioplegia or in the separate perfusion of a second arterial cannula. The arterial outlet port is where the arterial blood leaves the oxygenator and flows to the arterial line filter. The arterial line filter is a screen device constructed of synthetic material, with a specific pore size effectively blocking particles greater in size than the rating of the filter (20 and 40 μm). Microembolic particles originate from many sources in the extracorporeal circuit, including the BGED, tubing, and heat exchanger, and include various substances including polycarbonate, filter material fibers, silicone, and polyvinylchloride particles.154,155 Arterial line pressure also is measured between the arterial port and the arterial line filter. An arterial monitoring device can be placed “in line” to reflect arterial oxygen saturations and as a trending device for pH, Pao2, and Paco2. Just distal to the arterial line filter is a bubble detector that is an essential feature for the conduct of safe perfusion. This device is controlled by a microprocessor and is used to detect microgaseous and macrogaseous emboli. This is the last safety feature in the line before blood returns to the patient through the arterial cannula. When the ascending aorta is the site for arterial perfusion, an aortic cannula is placed through a purse-string suture with positioning of the cannula tip so that flow is directed cephalad toward the brachiocephalic vessels. The location of the aortic cannula and the direction of arterial blood flow emphasize the importance of assuring safe, continuous flow of filtered perfusate devoid of embolic particles.
Cardioplegia delivery systems
Melrose and colleagues156 were the first to describe chemical arrest of the heart with potassium citrate solution. The arresting solution was delivered with a syringe directly into the aortic root after the application of the aortic cross clamp.156 Similarly, others described delivery of various formulations of arresting solutions contained in collapsible intravenous bags using a sterile intravenous tubing set that was passed off the surgical field and attached to an inflatable infusion pump bag. This system was replaced by the use of recirculating circuits where the cardioplegia solution was recirculated in a system that consisted of a polycarbonate-filtered reservoir, and cooling of tubing that was placed in a bucket of ice (Figure 29-21). These recirculating systems provided filtration of the solution and improved control of delivery pressure and temperature. A single-pass system for delivery of blood cardioplegia in a predetermined ratio, which subsequently became the most widely used cardioplegia delivery system, was described in 1978 by Buckberg and colleagues157,158 (Figure 29-22). The ratio of blood to cardioplegia could be adjusted by changing the internal diameter of the tubing in the custom delivery set. The blood and crystalloid components are delivered to a miniaturized heat exchanger bubble trap before delivery at the surgical field. With this system, the temperature of the cardioplegia may be regulated from 4° C to 37° C with the use of a cooler/heater device. More recently, Menasché and colleagues159 described a microplegia system in which arresting additives are added directly to tepid blood from the CPB circuit with a standard infusion pump to arrest the heart. This greatly decreased the volume of crystalloid solutions delivered in a typical CPB procedure and avoided the detrimental consequences of volume overload and subsequent hemodilution. The authors also cited a few particular advantages of minicardioplegia, including improved oxygenation and improved control of blood volume, not to mention reduced cost.
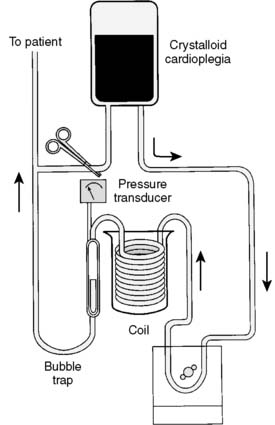
Figure 29-21 Coil-type cardioplegia delivery system.
This can be used for sanguineous or asanguineous cardioplegia solution.
Adjunct means of cooling and protecting the myocardium during aortic cross clamping include the use of topical application of cold solutions to prevent early transmural myocardial rewarming. A common method for cooling the myocardium is achieved by the surgeon creating a “pericardial cradle” in the chest by suspending the pericardium with stay sutures to the chest retractor. Cold (4° C) topical saline solution is then applied to the pericardium, bathing the heart in cold solution while a sucker line is placed in the well to evacuate the saline solution. Topical saline has been shown to cool the epicardium and diminish transmural gradients,160,161 but it also has resulted in phrenic nerve paresis and myocardial damage.162,163 An alternate technique involves a topical cooling device, which consists of a coolant flow pad in which cold (4° C) saline flows, separated from the body by a metal skeleton and polyurethane insulator, which protects the posterior mediastinum and phrenic nerve from hypothermic injury.164 The benefits of a topical cooling device over topical cold saline include a reduction in total hemodilution, procurement of a drier operative field, reduced blood loss in waste suction, and more uniform distribution of cooling.164–166 However, these devices are costly, require a separate RP for delivery, and may not be applicable for all procedures in which the heart will be lifted and elevated away from the posterior pericardium.

Disposable Cardioplegia Circuits
All cardioplegia delivery systems consist of two distinct components classified as either disposable or nondisposable devices.167 Effective myocardial protection is ensured only through the precise interface of both disposable and nondisposable components of the cardioplegia delivery system, which function to ensure safe, precise, and accurate administration of cardioplegic solutions. The disposable items that make up a standard cardioplegic circuit consist of three basic parts: a heat exchanger, a bubble trap with an incorporated filter, and various delivery cannulae. The disposable devices are utilized on a single-use basis and, because of their consumptive nature, represent the most significant cost associated with mechanical myocardial protection.
Early methods of cardioplegia delivery consisted of infusions of pharmacologic agents directly into the aortic root, or left ventricle, via handheld syringes. Unfortunately, such methods caused a heterogenous distribution of solution and led to the need for more precise delivery techniques. Many clinicians turned to a pressurized bag method in which a bag of crystalloid solution was placed in a pressure bag and cardioplegia infused at a semicontrolled rate dependent on the degree of pressure and the bore of the cardioplegic needle.168,169 Although the results were better than those previously obtained, there was a profound lack of safety features that included pressure monitoring and control systems, as well as air-handling capacity, and a lack of temperature control. Vertrees and colleagues170 described a simple circuit that utilized a coronary perfusion reservoir, a coil submerged in iced water, and an RP. This system was a significant improvement over previous techniques insomuch as it included a means to trap air and to measure pressure within the circuit.

Cardioplegic Delivery Catheters
Antegrade Aortic Root Cardioplegia
The distribution of cardioplegia to the myocardium with antegrade cardioplegia techniques is hindered in patients with atherosclerotic lesions, where distal perfusion is lost because of vascular obstruction. Furthermore, impaired delivery of cardioplegia may occur because of the retrograde escape of cardioplegia across the aortic valve. This commonly occurs if the patient has aortic insufficiency. However, it may occur in patients with a competent aortic valve that becomes distorted by the placement of the aortic cross clamp.171 Some antegrade cardioplegia cannulae have an integrated pressure monitoring lumen that allows measurement and display of the antegrade cardioplegia infusion pressure. It also is common to measure the cardioplegia delivery system pressure from a site distal to the cardioplegia delivery pump. A high system pressure alerts the team to an obstruction or malplacement of the cardioplegia delivery cannula. A low system pressure would occur from aortic insufficiency or from some breach of the delivery system. Conditions such as aneurysmal deformation of the ascending aorta and aortic valvular lesions both compromise the delivery of cardioplegia when administered via the antegrade direction. This concern has led to the search for alternative administration techniques for cardioplegic delivery.
Retrograde Coronary Sinus Cardioplegia
Retrograde delivery of blood to the heart via the coronary sinus and venous circulation was first proposed by C. Walton Lillehei. In 1982, Menasche and colleagues172 revisited the application of retrograde coronary sinus cardioplegia (RCSC), and with this technique they found superior maintenance of left ventricular function when compared with direct coronary artery perfusion in patients with coronary artery disease. Initially, this technique was proposed as a means of delivering cardioplegia as a replacement for direct coronary artery cannulation in procedures involving the aortic valve or root. However, the utility of RCSC quickly expanded as a means of delivering nutritive flow to the distal myocardium in patients with severe coronary artery disease.173,174 The delivery of RCSC, provided catheter position in the sinus and seal of the sinus by the balloon are optimal, results in a more uniform distribution of cardioplegia than antegrade and causes minimal disturbance of the operative field during administration.
Coronary sinus cardioplegia cannulae come in numerous configurations varying in catheter size, balloon configuration, stylet characteristics, and inflation mode (Figure 29-23). Various cannula designs are available incorporating geometric design to promote better fit into the sinus. The terminal end of the cannula is fitted with a balloon, which serves the function of seating the cannula in the coronary sinus so that cardioplegia is delivered into the coronary venous system, minimizing the amount of cardioplegia leakage into the right atrium. Some have textured balloon surfaces to minimize dislodgement of the catheter from the coronary sinus but result in leakage of the cardioplegia solution into the right atrium. Some designs have balloons that automatically inflate when flow is initiated through the catheter. Others require manual filling of the balloon with a syringe. In an animal model, Menasche and colleagues175 have shown that autoinflated retrograde catheters leak as much as 22% of cardioplegic flow, whereas manually inflated catheters had a leakage rate less than 1%. Several authors have described the efficacy of combining both antegrade and retrograde cardioplegic delivery methods in a single integrated system.176,177 These authors believe that the delivery of both antegrade and retrograde cardioplegia concomitantly supplies better perfusion to all regions of the myocardium despite the degree of coronary occlusive disease. Drainage is ensured through both thebesian and arteriosinusoidal vessels. Ihnken et al178 found in a large series of high-risk patients (New York Heart Association Class III and IV) that simultaneous delivery of warm cardioplegia directly into the bypass grafts and the coronary sinus was both safe and efficacious in assuring myocardial protection. A major disadvantage of this multisite simultaneous delivery method is that most of the flow will be directed down the path of least resistance and not necessarily uniformly to all segments of the heart muscle.
Despite the excellent results achieved with RCSC, complications resulting from cannulation and excessive pressurization of the coronary sinus occasionally are reported. These include rupture of the coronary sinus, poor perfusion of the right ventricle and posterior septum, and nonhomogenous flow patterns.179,180 In addition, controversy exists concerning the optimal delivery flow rate of RCSC. In one study of 62 patients, retrograde flow rates less than 100 mL/min resulted in reduced coronary venous effluent pH, with the authors recommending that maintenance of a minimum flow rate of 200 mL/min be considered whenever RCSC is used.181 The optimal delivery pressure also is controversial. Most centers use a guideline of 20 to 40 mm Hg pressure measured in the delivery catheter at a point distal to the balloon. It has been suggested by one researcher that pressures as high as 50 mm Hg are safe.182

Nondisposable Cardioplegia Pumping Mechanisms
The Quest MPS consists of a microprocessor-controlled electromechanical instrument and disposable delivery set that is integral to the device (Figure 29-24). There is a main pumping mechanism and a pumping subsystem that operates by a set of four pistons, each driven by a stepper motor, that align with several pouches in the disposable cartridge to mechanically displace the pouch contents. The subsystem pouches contain an arresting agent and one additional additive if required. The main pumping mechanism consists of two motor-driven piston pumps and valved pouches that alternately fill and pump extracorporealized blood and crystalloid solutions, providing constant cardioplegia flow. A number of sensors become activated when the cartridge is placed into the console, and the system software completes a series of self-checks before operation. The cardioplegic solution passes through a stainless-steel heat exchanger, which controls the caloric transfer of heat in congruence with the integral heater/cooler. There are four temperature sensors to provide temperature-controlled cardioplegia delivery, and the inlet water and cardioplegia temperatures are continuously displayed.
Priming solutions and controversies
Before ECC can be attempted, the patient must be connected to the CPB machine, necessitating the creation of a fluid-filled circuit to ensure continuity with the patient. Not only is it important that the circuit be “primed,” but it also must be completely devoid of any gaseous bubbles or particulate matter that potentially could embolize. For this reason, perfusionists often perform painstaking maneuvers to rid the circuit of bubbles before bypass. Historically, early priming solutions were formulated to closely match the patient’s own rheologic characteristics, which necessitated the use of fresh whole blood.183 Priming the early oxygenating circuits required vast quantities of blood and balanced electrolyte solutions, and cardiac operations often were scheduled around the availability of blood donors. Eight to 10 units of heparinized blood was required to prime the average CPB circuit,184 and the risk for contracting viral hepatitis was substantial. The addition of blood to priming solutions also induced the capillary leak syndrome,185 which may implicate histamine as a contributing factor in leading to postpump pulmonary dysfunction. As a result of the excellent work on hemodilution by Messmer and colleagues186,187 and others,188 it is now the rare adult patient who receives any blood in the circuit before the initiation of CPB. Instead, balanced electrolyte solutions are the first choice in priming bypass circuits. In pediatric circuits, however, where the circuit volume often exceeds the patient’s circulating blood volume, allogeneic blood products often are added to the prime to reduce the risk for anemia and hypoproteinemia (see Chapters 28, 30, and 31).
When nonhemic primes are used during CPB, there is a concomitant reduction in SVR at the onset of ECC as a result of the reduced viscosity of the blood.189 Although the oxygen-carrying capacity of the pump perfusate is reduced by hemodilution, overall oxygen delivery may not be significantly affected because the reduced viscosity enhances perfusion. Safe levels of hemodilution are dependent on multiple factors that include the patient’s metabolic rate, cardiovascular function and reserve, degree of atherosclerotic disease and resultant tissue perfusion, and core temperature. Although an absolute value for the degree of hemodilution tolerated will vary among individual patients, the studies of Kessler and Messmer support a minimal hematocrit value of 20% to ensure oxygen delivery and tissue extraction.190,191 Much progress has been made in understanding absolute tolerances of hemodilution through treating patients of certain religious groups who refuse the transfusion of allogeneic blood products.192
For years controversy has raged over the inclusion of colloids in pump primes, with specific emphasis placed on the value of albumin as a routine prime constituent.193,194 The nonphysiologic effects of CPB, with both nonpulsatile and pulsatile perfusion, are known to alter various hemodynamic and physiologic forces affecting the extravascularization of plasma water, especially in the lungs, which leads to respiratory dysfunction.195 Total body water is increased after CPB, leading to tissue edema and altered organ function.196 Total body fluid shifts may take several days after CPB to correct because of the degree of hypotonicity created during bypass.197 Although the pathophysiology related to tissue edema is appreciated, the influence of factors such as total bypass time and pressure gradients during CPB remains to be elucidated. Priming of the CPB circuit with crystalloid solutions alone reduces colloid oncotic pressure, and this reduction is directly related to the total volume of prime solution and the overall level of hemodilution. Hypo-oncotic primes promote tissue edema through interstitial expansion with plasma water.193 A significant decline in plasma albumin occurs after CPB in patients who have been exposed to crystalloid-only primes.198 Albumin199 and various high-molecular-weight colloid solutions are added by some groups to the prime to offset these changes, although the benefits associated with each practice remain controversial.
Both high- and low-molecular-weight hydroxyethyl starch (HES), synthetic colloids that are derived from amylopectin, have been used as volume-expanding adjuncts to crystalloid primes.200,201 HES is an effective colloid oncotic increasing agent that has colloidal properties similar to that of 5% albumin and is relatively inexpensive.202 In postoperative pulmonary function studies of extravascular lung water accumulation and alveolar-arterial differences in oxygen tension, there was no significant difference seen when either 6% HES or 25% albumin was used in the pump prime.203 Platelet counts tend to be lower when hetastarch (the high-molecular-weight form of HES) is used in CPB primes, but this reduction has questionable clinical significance.92 When HES is infused in cardiac patients in the immediate post-CPB period, significant derangements in hemostasis have been reported, despite not being present when the solution was given to patients on entry to the intensive care unit.204 The authors hypothesized that the infusion of HES in the immediate post-CPB period, when fibrinolytic stimulation is at a maximum and platelet count is at a nadir, could be responsible. The low-molecular-weight HES compound pentastarch may offer future promise as a more potent volume expander than hetastarch by causing fewer alterations in hemostasis.99 One study failed to show a positive correlation between extravascular lung water and plasma colloid oncotic pressure/pulmonary capillary wedge pressure gradient after CPB when bubble oxygenators were used.205 The use of hypertonic saline solutions (7.2% NaCl) in combination with hetastarch was recently shown to result in better patient hemodynamics with lower fluid requirements during CPB.206 There also was evidence that this combination technique may result in better postoperative pulmonary function compared with hetastarch alone.
The addition of glucose to prime solutions remains an area of controversy because of the relation between high glucose concentrations on CPB and neurologic dysfunction.207,208 Metz and Keats209 reported that when glucose was included in prime solutions of 107 patients undergoing CPB, there was a lower fluid balance as evidenced by significant reductions in crystalloid administration and there was no increased neurologic dysfunction. Although there is a paucity of prospective studies on the neurologic outcome of cardiac patients after perioperative glucose administration, there is evidence that glucose administration is associated with greater morbidity after cerebral ischemia.210,211 The hypothesis for this pathophysiologic phenomenon is related to a shift in glycolysis from aerobic to anaerobic pathways during ischemia, which results in a metabolic end-product accumulation of lactate and decline in intracellular pH.212 Until further work is done in which tightly controlled preoperative and postoperative neurologic examinations are performed, it may be advantageous to manage glucose conservatively, restricting glucose-containing solutions in cardiac patients.213
Marelli and colleagues studied perioperative fluid balance in 100 adult patients divided into two groups who either did or did not receive albumin (50 g) in the bypass prime.214 They were unable to show any improvement in more than 40 clinical parameters affecting patient outcome when albumin was included in the prime. It is known that, within the first few seconds of CPB, a proteinaceous film is deposited on the surface of all extracorporeal circuit surfaces.215 Priming the pump circuitry with albumin is thought to decrease the initial adsorption of protein components, which would increase biocompatibility. Bonser et al216 examined complement activation in 36 patients who received priming solutions of either crystalloid, crystalloid plus albumin, or crystalloid plus the plasma expander polygeline. They measured products of both the alternate and common complement pathways and found a significantly greater level of activation in both the crystalloid and crystalloid plus albumin groups when compared with the polygeline patients. A similar study examined both plasma and dextran 70 in priming solutions and their effects on complement activation.217 When plasma was added to the prime, a significant increase in the plasma concentration of C3 activation products (C3c and C3dg) was observed, which was not present in the dextran 70 group. A study of the effects of postbypass hypoalbuminemia demonstrated that this reduction was well tolerated except in patients with poor left ventricular function.97
Further important considerations in choosing a priming solution for CPB circuits include alterations induced by changes in electrolyte activity. Balanced electrolyte solutions are the first-choice base solutions of most prime solution “cocktails” used by perfusionists. Lactated Ringer’s solution, Normosol-A, and Plasmalyte are used frequently because of their electrolyte compositions and isotonicity. One potential concern with the latter solutions focuses on the absence of calcium and potential for hypocalcemia. Calcium concentration varies depending on the type of prime constituents, as well as the presence of citrate in allogeneic blood products. Hysing et al218 reported substantial differences in the calcium concentrations among five different prime solutions throughout the bypass period. They reported an initial decline in ionized calcium with the initiation of CPB followed by a normalization over the first 30 minutes of ECC and emphasized the importance of frequent monitoring of this cation. In pediatric ECC and in certain adult patients who have preoperative deficiencies in either hemoglobin or coagulation proteins, it may be necessary to prime the heart-lung machine with allogeneic blood products. When calcium-containing prime solutions, such as lactated Ringer’s, are used, additional anticoagulation is necessary to prevent circuit thrombus from forming before the initiation of CPB. The most frequently used ratio of heparinization for CPB is 2500 IU heparin for each liter of prime solution, which would ensure adequate anticoagulation for both sanguineous and asanguineous prime solutions.
Computers in perfusion
Microprocessors are ubiquitous, and their incorporation into the CPB circuit is profound and encompassing.219 The acceptance of computers in clinical practice has been a gradual process among perfusionists,220 which may reflect an overall tendency to resist the unknown coupled with reluctance to confront the anticipated complexity of advancing technologies. Microprocessors can be manipulated easily by user requirements to mimic logic and related functions. They are able to function without interruption through all processes involved in cardiac surgery, with minimal downtime ensured with proper utilization and maintenance. Microprocessors have proved to effectively decrease communication delays by processing vast quantities of information quickly, satisfying the demand of immediate output to facilitate decision processes. Their utility in organizing and analyzing large datasets aids in producing trending information, as well as identifying aberrant situations.
The practice of ECC is represented by a multitude of physiologic and mechanical events, which are closely interrelated and constantly changing. The continuous generation of information during these events provides a perfect situation for data capture and processing, optimizing the conduct of perfusion.221–223 Most heart-lung machine manufacturers market data processing systems that, through a microprocessor, interface with various operating room equipment including bedside monitors and anesthesia machines. Peripheral components of the ECC, such as online blood gas analyzers, coagulation monitoring devices, heater/cooler units, and autotransfusion devices are all microprocessor controlled and easily can be integrated into a single operating system. This organized feedback acts as an information source that centralizes data handling. The information obtained during ECC is formidable and is best manipulated by a database management system. The database management system controls how the computer stores and retrieves data and is nothing more than a tool enabling multiple complex calculations to be performed quickly.
Some of the data types obtained and analyzed during ECC include patient anthropomorphic information and perfusion and/or oxygen requirements calculated from body surface area and anticipated metabolic demands. The determinations of prime constituents and medication dosing are routinely performed via simple computer programs, together with predicting postdilutional hematocrits.224 The conduct of CPB is reflected by terminals that display information on pump dynamics, perfusion flow, SVR, ventilatory needs, and cardiac index. In-line arterial blood gas monitors can process changes in both gas exchange and pH as often as every second. Arterial and venous samples can be measured continuously, and arterial-venous oxygen differences, oxygen delivery and consumption, and oxygen extraction ratios calculated. Input and output ratios can be determined quickly by loading values for volume input (crystalloid, colloid, blood products) and volume output (urine, aspirated waste, ultrafiltration [UF], and autotransfusion). Graphic interpretation of data is facilitated by trending summaries over the CPB period, which could incorporate “flags” as markers of events that deviated from standard treatment. This information could then be tabulated as norms and indices that could alter patient management strategies and be used in quality management assessment. A case summary then can be generated that could be used for review and interpretation, or as an official record of the case.225
Some of the additional benefits of computers in perfusion include their utilization as educational and training aids,226 for assessment of the patient’s physiologic status227 and optimization of metabolite delivery.228 Perhaps no area is more critical in the application of microprocessors in perfusion than that of safety. No matter how integrated a circuit becomes, or how technologically enhanced are the CPB components that evolve, without the conduct of safe, uneventful bypass, the advances are meaningless. Advances in bubble detection devices that incorporate ultrasonic systems have greatly reduced the risk for gross air embolism. One shortcoming in safety devices remains the absence of reliable level-detection systems that monitor venous reservoir volumes. Arterial pressure monitoring with feedback loops that will interrupt pump operation in the event of high pressure, or gas emboli detection, has greatly enhanced the quality of CPB.
The future of computers in ECC is probably as much a function of education as technologic advance. The most advanced systems, with the highest price tags, are of absolutely no value without the fundamental knowledge of how to operate them. Nevertheless, some of the future goals of microprocessor-enhanced perfusion include inexpensive, menu-driven software programs that would reduce the time spent learning terminology and functions. A reduction in system complexity with succinct troubleshooting guides may decrease technical support required for system maintenance. Finally, computers will serve as valuable training tools to assess skills and competencies before actual clinical situations.229–231 Indeed, several perfusion education programs incorporate clinical computer simulation as a means of assessing student proficiency in didactic areas before operating the pump in the operating room.
Perioperative methods of red blood cell conservation
Homologous blood is a precious resource, the transfusion of which confers both benefits and risks. The practice of transfusion began in the 1930s with the Nobel Prize–winning work of Landsteiner.232 Transfusion medicine expanded through experience gained in battlefield medicine and the development of cardiac surgery, vascular surgery, and oncology. Of the 29 million transfusions administered each year, it is estimated that one third to half are not administered in accordance with evidence-based indications.233 Cardiac surgery programs are one of the leading consumers of blood and blood products. More than 80% of the blood used in cardiac surgery is transfused in 15% to 20% of the patients undergoing surgery.234 In the late 1970s and early 1980s, there was concern about transfusion-related hepatitis B and C, HIV, and bacterial infections. With modern blood bank processes and screening, these risks have become extremely low; however, other associated risks including transfusion-related lung injury, leukocyte-related target organ injury, transfusion errors, and bacterial infections are comparatively common. Furthermore, there is a growing confirmation of the relation between transfusion and reduced short-term survival, long-term survival, and HF in cardiac surgery patients.235–241 Transfusion of stored blood and blood products is related to a host of adverse effects including release of bioactive compounds that cause inflammation, reduced oxygen availability to tissues, and other immunomodulatory effects, all of which contribute to increased morbidity and mortality242 (see Chapters 30 and 31).
Transfusion practice varies widely, and this variation is based largely on individual physician practice.243 The administration of allogeneic blood products during cardiac surgery continues to be a major concern for both patients and clinicians. The changing population of patients undergoing cardiac surgery has presented new transfusion-related challenges that are being addressed through both pharmacologic and mechanical means. A combination of increasing age of patients undergoing cardiac surgery and a greater percentage of patients undergoing resternotomy procedures has increased the challenge of bloodless cardiac surgery. Although the safety of receiving allogeneic blood has increased dramatically, risks remain and need to be understood when considering patient transfusion. These risks include both hemolytic and nonhemolytic reactions, disease transmission, graft-versus-host disease, recipient alloimmunization, and hypervolemia.244 Results generated when meticulous attention and adherence to conventional blood conservation techniques have been followed are promising.245,246 However, the diversity in surgical practices, anesthesia management, and postoperative care all represent a multifactorial process rendering reproducibility difficult from center to center.
Isovolumic hemodilution combined with hypotensive anesthesia is an effective strategy for limiting allogeneic transfusions. Intraoperative phlebotomy before ECC is performed easily with volume replacement consisting of either colloid or crystalloid solutions ranging, respectively, from 1 to 3 mL for every milliliter of phlebotomized blood. Relative contraindications to performing intraoperative donation may be left main stenosis, unstable angina, critical aortic stenosis, hemodynamic instability, and a history of cerebrovascular disease.132 The increased risks associated with allogeneic blood are well-known. Although the dangers of receiving contaminated blood vary among geographic regions, it is accepted that the incidence of post-transfusion hepatitis C has decreased markedly,247 whereas the risk for HIV transmission has been reported to be as high as 1 in 100,000 transfusions. The emphasis on reducing the risks associated with blood exposure has long been evident within hospitals. Standing blood utilization committees are charged with the responsibility of reviewing transfusion practices within hospitals. Some states have enacted laws that protect the rights of patients undergoing elective surgical procedures in regard to blood transfusions. Legislative actions in California have established specific mandates for physician involvement in ensuring that patients at risk for transfusion are informed of the availability of alternate techniques for reducing the risk for allogeneic blood exposure. Clearly, the impetus directing specific blood replacement practices associated with cardiovascular surgery will come under intense scrutiny from both internal and external sources of review.

Preoperative Donation
Predonation before surgery would seem to be a plausible means of obtaining blood and avoiding homologous transfusion, particularly in this era of heightened awareness of the dangers associated with receiving allogeneic blood products. The use of autologous blood not only is nonimmunogenic but reduces the hospital’s dependence on blood banks. However, this technique has had limited success in treating the cardiac surgery patient.248 From a blood bank perspective, predonation of blood is a logistic nightmare. Furthermore, there are many contraindications to autologous blood collection in cardiac patients, including aortic stenosis, left main coronary artery disease, idiopathic hypertrophic subaortic stenosis, unstable angina, cardiac failure, recent myocardial infarction, ventricular arrhythmia, symptoms on the day of donation, and the emergency need for surgery. Only 10% of transfusion recipients may be eligible for self-donation249; therefore, the majority of surgical candidates require alternative measures to reduce exposure to the general blood supply.

Plasmapheresis
Plasmapheresis is the separation of whole blood into plasma (which may be platelet poor or platelet rich), platelets, and RBCs. The first clinical utilization of plasmapheresis in thoracic surgery was reported by Ferrari and colleagues250 in 1987. The benefits of plasmapheresis in the cardiac surgical patient are derived from the production of autologous blood products that, because of their separation into isolated components, can be administered to treat specific deficiencies related to the patient’s hemostatic needs. One of the most critical advantages involves the treatment of patients who would otherwise not be candidates for predonation of blood. The logistic difficulties are easily overcome when this method is used in the operating room with the patient under the direct care of the anesthesiologist.
In the operating room, removal of whole blood should begin as soon as central venous access is established. The most common site is the internal jugular vein, although external jugular, saphenous, and antecubital veins all have been used. The anticoagulant used is sodium citrate, which should be delivered at a rate of 1 mL/12 mL whole blood. The blood is drawn into a collection bag and then transferred to a processing bowl in the autotransfusion device via a peristaltic pump. The calculated plasma volume to be removed has generally been set as 20% of the patient’s circulating plasma volume,251 which ranges from 10 to 12 mL/kg.252 Approximately 200 mL whole blood can be processed in 12 to 14 minutes.251,252 The number of cycles necessary to complete the calculated draw volume is dependent on the patient’s RBC mass and plasma volume. Volume replacement should be carried out both before and during the sequestration process. Replacement therapy varies among institutions, but the solutions most often reported include high-molecular-weight HES (6% HES),252 crystalloid solutions, and 5% albumin.253 After the plasma has been removed, the RBCs can either be reinfused to the patient via the return mode of the machine or saved and returned after CPB. The product yield is dependent on the patient’s predraw platelet level and plasma volume but generally is between 1.0 and 2.5 × 1011 platelets/600 mL product.251–253
The results of plasmapheresis in thoracic surgery have been encouraging, although questions have been raised concerning efficacy.254,255 When the use of autotransfusion alone has been compared with plasmapheresis, the patients treated with PRP have had lower positive fluid balances than patients who had cell washing used as a method of hemoconcentration.252 Several clinical trials have shown a decreased usage of allogeneic blood products, including plasma and platelets, during the hospitalization of patients treated with PRP.252,256–258 After the reinfusion of autologous PRP, patients have had greater operative platelet counts,139 decreased postoperative bleeding,250,259 and greater fibrinogen and antithrombin III (AT III) concentrations.260 Giordano et al reported that the concomitant use of autotransfusion and PRP reduced transfusions from 13.67 to 6.32 allogeneic blood exposures per patient.253
Stammers et al261 have described additional benefits of plasmapheresis in the immediate postinfusion period and can be related to an overall reduction in fibrinolytic tendency. It also has been shown that, through reductions in blood transfusion, PRP administration to patients in a hyperfibrinolytic state ameliorates the effect of fibrinolytic substances. Although specific factors in the plasma product have not been identified, the rapid reduction in fibrinolysis suggests the presence of endogenous antifibrinolytic substances.
The effects of platelet-affecting drugs on both platelet-poor plasma and PRP are germane because a substantial number of cardiac patients are exposed to these medications before surgery. Giordano and colleagues256 studied patients who received warfarin (Coumadin), heparin, or nonsteroidal anti-inflammatory agents up to the day before surgery. When these patients were treated with PRP, there was no effect on postoperative bleeding after reinfusion of PRP. The contraindications to plasmapheresis are based mainly on the patient’s inability to withstand low hemoglobin concentrations before the initiation of CPB. Patients with hemoglobin concentrations of less than 10 g/dL generally have their RBCs returned in between cycles.

Autologous Priming Techniques
Rosengart et al’s262 prospective trial led the way for other investigators. Their study, conducted on 60 first-time CABG patients, established that AP limits hemodilution and reduces the number of patients needing RBC transfusions. Since their report, other randomized and observational trials have reported similar benefits.263–267 However, Murphy et al268 concluded that AP does not offer a clinical benefit as a blood conservation technique. Their trial was limited by design (retrospective cohort, single surgeon), and the limited reporting of AP techniques and volume management strategies prevents replication. More recently, Trowbridge et al269 designed a prospective study aimed at identifying optimal characteristics of the AP process and found that when used effectively, defined as removal of at least 1300 mL or when less than 10% of AP volume was returned to the patient, greater hematocrit values were obtained and fewer patients received transfusions. Furthermore, they reported that the amount of removed prime returned to the patient was related to the patient’s urinary output and the amount of blood loss during the procedure.269

Perioperative Salvage and Autotransfusion
Cardiotomy Suction
Shed blood from the surgical field and blood vented from the LA, left ventricle, pulmonary artery, or the aorta is collected and reinfused into the CPB circuit through the cardiotomy suction system. This system is composed of tubing usually 1/4-inch internal diameter directed through an RP into a filtered reservoir. Cardiotomy suction blood contains fat, bone, lipids, and other debris from the surgical field. This blood is also exposed to air, shear forces, and artificial surfaces that cause exacerbation of the systemic inflammatory response and result in microcirculatory dysfunction. These substances may traverse the CPB circuit, enter into the arterial line, and ultimately obstruct the microcapillary circulation of the patient. Brown et al270 identified thousands of embolic lesions in the brains of patients who died within 3 weeks of cardiac surgery and reported an association between embolic lesions and duration of CPB. For each 1-hour increase in the duration of CPB, the embolic load increased by 90.5%. Cardiotomy suction blood has been identified as a major source of lipid emboli in several studies.271–273 For this reason, some have advocated eliminating the use of cardiotomy suction, which is returned directly to the ECC. Several clinical studies have examined the effects of eliminating cardiotomy suction. In a randomized trial enrolling CABG patients, use of cardiotomy suction resulted in significant increases in thrombin generation, neutrophil and platelet activation, as well as the release of neuron-specific enolase.274 Nuttall et al,275 in a study of patients in whom an open venous reservoir was used, compared the return of cardiotomy suction directly to the ECC, versus sequestration and processing of cardiotomy blood to a cell saver. A battery of blood tests were performed to evaluate platelet function, and no significant difference in any of the tests or in blood transfusion requirements was observed.

Cell Salvaging through Centrifugation and Washing Techniques
The major components of any automated or manual device used for cell processing in autotransfusion are listed in Table 29-1. The process begins with the aspiration of blood from the surgical site together with an anticoagulant via a double-lumen line. The blood, together with other operative contaminants including bone chips and adipose tissue, is then collected in a cardiotomy reservoir, functioning as the first filtration, with depth and screen filters ranging in size from 40 to 120 μm. A peristaltic pump then transfers the contents from the cardiotomy reservoir into a centrifuge bowl that has been specifically designed to separate blood according to specific particulate density. Centrifugation necessary for this separation process generally is between 4800 and 5600 rpm (Figure 29-25). The volume of the bowl is an important characteristic of these devices because the volume of the bowl has a role in the minimum amount of shed blood required to obtain an acceptable hematocrit in the returned product. Some of the systems come with 125-mL bowls for use with small patients or when smaller amounts of shed blood are anticipated. The heavier RBCs are packed farthest from the axis of rotation, whereas the lighter plasma and crystalloid fractions remain closest to the center of the bowl. A wash mode is initiated when the centrifuge bowl has reached its optimal packed RBC level, with sterile physiologic saline pumped through the RBC layer, removing plasma-free hemoglobin, clotting factors, anticoagulant, and nonautogenous particles.
TABLE 29-1 Basic Components of a Typical Autotransfusion Device
Centrifuge | |
Centrifugal bowl | |
Aspiration set | |
Anticoagulant | Cardiotomy reservoir |
Wash fluid | |
Waste bag | |
Reinfusion bag |
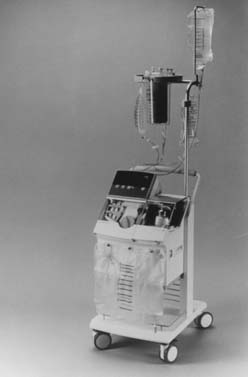
Figure 29-25 Autotransfusion cell processing device using a peristaltic pump and centrifuge for processing of shed blood.
(Courtesy of Haemonetics Corporation, Braintree, MA.)
One new cell-saver design, the Continuous Autotransfusion System (CATS; Terumo Cardiovascular, Tustin, CA), does not have a Latham bowl and functions in a continuous manner such that the packed RBC product is harvested during centrifugation (Figures 29-26 and 29-27). This approach has several advantages: a lower minimum amount of shed blood is required before packed RBCs may be harvested, a greater RBC concentration of resultant product is obtained, and, most importantly, the separated lipid layer remains suspended during the process. With the traditional Latham bowl systems in which the centrifugation is stopped between cycles, lipids may remix with the final RBC product and be returned to the patient. Several recent studies have shown that the use of a continuous processing system is superior to the Latham bowl-type systems in terms of lipid removal and neurocognitive outcomes.276,277 Kincaid et al278 studied effects of blood processing technique on production of lipid emboli in the brain (Small Capillary Arterial Dilations) in a canine model. Two recent randomized trials were designed to determine whether use of a cell saver reduced neurocognitive dysfunction after CPB. In Rubens et al’s study,279 266 patients undergoing CABG surgery were randomized to two groups: an unprocessed cardiotomy suction blood group (control) and that processed by centrifugal cell washing (treatment group). Greater blood product administration and blood loss were observed in the treatment group. No differences in microemboli generation, neurocognitive dysfunction, or other adverse events were demonstrated between groups. In another study by Djaiani et al,276 patients randomized to cell processing with a continuous autotransfusion system had reduced transfusion requirements and improved neurocognitive function. The latter study used a continuous cell processing system that has been shown to reduce blood lipid content. The former study used a Latham bowl intermittent system, which previously has been shown to be ineffective at lipid removal. Further studies are necessary to define the impact of cardiotomy suction on clinical outcomes.
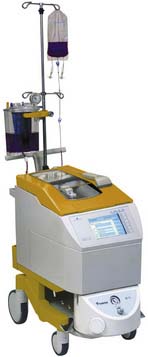
Figure 29-26 Continuous Autotransfusion System (CATS).
(Courtesy of Terumo Cardiovascular Systems, Ann Arbor, MI.)
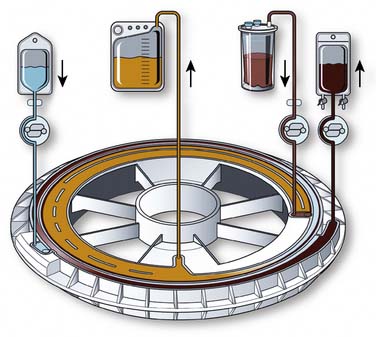
Figure 29-27 Continuous Autotransfusion System Cell processing cassette.
(Courtesy of Terumo Cardiovascular Systems, Ann Arbor, MI.)
Autotransfusion as a routine practice in cardiac surgery has received mixed acceptance. Cost-effectiveness always has been a concern, with the prevailing belief that the utilization of autotransfusion should be considered only when anticipated blood loss would result in a reinfusion of 1 to 2 units of processed RBCs.280 However, the active interest in minimizing patient exposure, combined with the use of smaller-volume centrifuge bowls, have prompted increased use of autotransfusion during cardiac surgery. Young et al281 reported a reduction in allogeneic RBC transfusion from 4.2 to 1.5 units/patient when autotransfusion was used in cardiac patients. The quality of RBCs processed via autotransfusion during cardiac surgery also has been compared with fresh autologous blood, with RBCs collected from the operative field having an in vivo survival comparable with that of phlebotomized blood.282 Schwieger et al’s283 study examined autotransfusion-collected blood for risk for infection and found that patients who had blood salvaged and processed by cell-washing equipment had no higher rate of infection than patients who had no autotransfusion but received banked blood.
In addition to aspirating shed blood from cardiac patients, the autotransfusion device can be used to concentrate the pump perfusate at the termination of CPB. Although this process is known to reduce the protein concentration of the perfusate when compared with reinfusion of the unprocessed pump contents, this method significantly reduced allogeneic banked blood exposure.284 Many centers will infuse the blood contained in the CPB circuit at the termination of bypass. The blood in the CPB circuit is displaced with a balanced electrolyte solution so that the pump remains primed should it be necessary to return to bypass. Sometimes vasodilators are administered to the patient to increase capacitance and allow this blood to be reinfused. It is common practice in neonate and pediatric cardiac surgery to use a technique referred to as “modified ultrafiltration” (MUF). With this technique, UF is conducted to remove plasma water, while at the same time the blood remaining in the CPB circuit is slowly infused into the patient. Similarly, this technique may be performed using a device called a hemobag, where the contents of the CPB circuit are infused into a collection bag and the contents of the bag are then ultrafiltered and returned to the patient.285,286 The product returned to the patient has a lower water content and greater concentration of RBCs, platelets, white blood cells, and plasma proteins.
The contraindications to the use of autotransfusion are relative and are evaluated on a per-case basis. Therefore, the relative contraindications include contaminated wound sites and/or septic procedures, malignancy, aspiration during caesarean sections, and concurrent use when microfibular collagen agents are present. The risks assumed with using cell salvaging and reinfusion techniques in these patients must be weighed against the inherent benefits of autologous versus allogeneic transfusion. Hemoglobinemia resulting from RBC destruction caused by exposure of blood to disposable autotransfusion circuitry and trauma caused by aspiration and mechanical treatment has been reported.287 The reinfusion of packed RBCs may also lead to pulmonary insufficiency if inadequate filtering of the product results in microaggregate embolization in the pulmonary vasculature. The risk for air embolism also is increased whenever extracorporeal devices are used; therefore, proper precautions with operator vigilance are paramount in assuring patient safety.

Postoperative Shed Mediastinal Blood Collection
The collection and reinfusion of postoperative mediastinal blood after cardiac surgery have been described as postoperative autotransfusion (PAT)288,289 and have been used in cardiac surgery since 1978.290 This process consists of connecting either a dedicated collection device or a cardiotomy reservoir from the extracorporeal circuit directly to mediastinal chest tubes and a negative pressure source. Blood flows from the mediastinal tubes into the collection reservoir, where it undergoes gross filtration (40 to 120 μm). The collected product then is reinfused back to the patient via an infusion pump and through an additional 20-μm filter. The volume collected after the operation varies from center to center and according to procedure but may range from 400 to 1200 mL over the first 24 hours. Shed mediastinal blood is defibrinogenated; therefore, levels of fibrin(ogen) split products are increased after reinfusion.291
Morris and Tan292 commented on the use of PAT in 155 consecutive cardiac patients. These authors found a substantial and significant reduction of approximately 30% in the use of allogeneic blood products in patients undergoing cardiac surgery with PAT. Other authors have found that when the postoperative blood loss was less than 500 mL, PAT conferred no benefit in reducing banked blood requirements.293,294 In a prospective, randomized study of cardiac patients undergoing CABG surgery, Bouboulis et al295 found no benefit to the use of PAT, and patients receiving autotransfusion had greater incidences of febrile reactions. These authors and others have advocated the concurrent use of a cell-washing device to process the PAT product before reinfusion.296 A portable cell-washing device, the CardioPAT, is now available for processing shed blood from thoracic and pleural drainage. This device functions similarly to the centrifugal cell-washing systems. The major differences are a smaller footprint and a slower rate of processing than the traditional cell-saver systems. The processing of this blood removes activated white blood cells and fibrinolytic mediators, which may be associated with hemolytic reactions found when unwashed blood is reinfused. Schmidt et al297 have shown that the reinfusion of PAT blood in CABG patients caused an increase in levels of cardiac enzymes including creatine kinase-MB activity. These increases may result in a compromised assessment of myocardial injury in patients undergoing cardiac surgery with PAT.

Ultrafiltration
UF is a process in which plasma water is filtered from whole blood via a semipermeable membrane (see Figure. 29-34). Although primarily a method of removing plasma water, it is also an effective means of blood conservation in that it indirectly increases the volume of RBCs, platelets, and coagulation factors. The technology used in UF was initially developed as a treatment for dialysis patients who became volume overloaded.298 UF is used synonymously with hemofiltration and diafiltration and uses similar devices and principles to those seen in continuous arteriovenous hemofiltration and slow continuous UF. Continuous arteriovenous hemodiafiltration uses a dialysate that flows countercurrent to the direction of blood flow around the fibers, removing plasma solutes and electrolytes by diffusion. When UF is used exclusively to remove excessive fluid from CPB circuits, it has been referred to as hemoconcentration.
Cardiac patients are particularly susceptible to volume overloading via crystalloid administration for hemodynamic maintenance and prime solution of the heart-lung machine. Priming of the extracorporeal circuit with nonhemic solutions results in hemodilution that ranges from 33% to 200% of the patient’s volume. After cardiac surgery, the amount of extravascular fluid load may increase by greater than one third of the adult patient’s prebypass blood volume,299 whereas in pediatric perfusion, the total volume of hemodilution may far exceed the patient’s preoperative blood volume.300 It is well-known that the body responds to hemodilution by increasing cardiac index as a result of reduced SVR.301 In sick or compromised hearts, however, there is less myocardial reserve, which may result in inadequate oxygen delivery and hypoperfusion because of decreased output. UF not only reduces the risk for volume overload in these patients, but it can be used to correct electrolyte and acid-base imbalances.
UF is a hemoconcentration technique by which plasma water and certain low-molecular-weight plasma solutes are separated from circulating whole blood by free convective transport. A semipermeable hollow-fiber membrane operates on the principle of a hydrostatic pressure differential generated across the membrane to separate an ultrafiltrate from blood (Figure 29-28).302 The composition of the ultrafiltrate is similar to that of glomerular filtrate.303 Uremic toxins also are selectively removed from the circulating perfusate,304 which may decrease the incidence of acute tubular necrosis as a result of CPB. Sieving coefficients that are based on the molecular weight of plasma solutes and the porosity of the UF device have been established by the various manufacturers. These are determined by dividing the concentration of the solute in the filtrate by the concentration in the plasma. Generally, solutes greater than 50,000 Daltons do not pass through the membrane pores (albumin has a molecular mass of 65,000 Daltons).
The advantages of UF in the cardiac patient are:
The UF rate is the rate at which plasma volume is removed from the blood passing through the ultrafiltrator and is dependent on TMP and the surface area of the hemoconcentrator.307
There are few contraindications to hemoconcentration with an ultrafiltrator. As with any nonendothelialized material, biocompatibility becomes an important issue. Leukopenia and complement activation have both been reported when blood is exposed to cellulosic membrane material.308,309 Excessive TMP may lead to increased RBC trauma and release of plasma-free hemoglobin. When concentrating the pump contents, care must be taken during reinfusion because of the retention of heparin in the hemoconcentrated product. The heterogenous molecular size of heparin varies the amount of heparin retained in the hemoconcentrate. Strict cost analysis during routine cardiac surgery is difficult to quantify because patients are known to tolerate positive fluid balances of up to 4 L without adverse pathologic effects.173 Therefore, the benefits of UF in these patients have yet to be established.

Modified Ultrafiltration
In 1991, Naik et al310 described a modification to the technique of UF that has since been extensively applied to pediatric patients undergoing cardiac surgery. Nearly 75% of all pediatric centers in North America routinely use MUF for neonate, infant, and pediatric cardiac surgery.311 Pediatric patients are thought to be more susceptible to the injurious effects of fluid overloading and may benefit from this technique because of the greater reoperation rate and use of profound and deep hypothermia seen in pediatric cardiac surgery. The technique is applied after CPB and allows the UF of both the circuit contents and the patient. The timing of MUF is critical and permits a rapid increase in patient hematocrit by the removal of plasma water.312 The results associated with MUF have been very encouraging and have included reductions in postoperative morbidity,313 reduced blood loss and blood utilization,310,314 reduced inflammatory mediators,315,316 and improvements in myocardial function317 and cerebral oxygenation.314
MUF generally is performed shortly after termination of CPB and is completed with a typical ultrafiltrator. In pediatric operations, these devices are routinely set up and primed together with the entire ECC. The ultrafiltrator is placed in-line at a point distal to the membrane oxygenator with the inlet of the device connected to the arterial line and the outlet connected to the venous return line (Figure 29-29). An RP controls the flow rate through the MUF circuit and is located in a parallel circuit between the arterial and venous cannulae. Such a configuration allows conventional UF to take place during the CPB procedure. On separation from CPB, the patient becomes the source for blood for MUF by clamping both the arterial and venous lines proximal to the MUF circuit, draining from the arterial cannula, and reinfusing directly into the venous cannula. The remaining pump contents also are concentrated during MUF and serve as a volume replacement for the removed plasma water. Blood flows during MUF vary with patient size and hemodynamic stability.
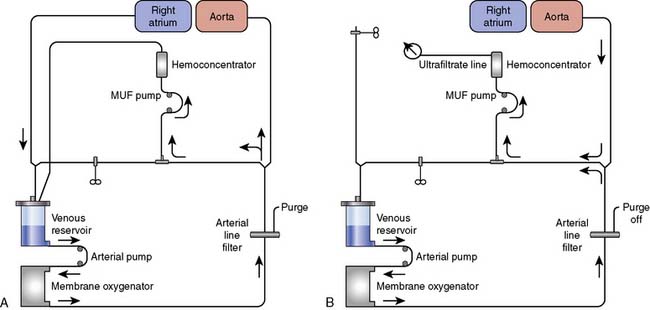
Figure 29-29 Modified ultrafiltration (MUF) circuit used for pediatric cardiac surgery. A, Conventional ultrafiltration. B, MUF.
(From Darling EM, Shearer IR, Nanry C, et al: Modified ultrafiltration in pediatric cardiopulmonary bypass. J Extra Corpor Technol 26:205, 1994.)
During MUF, there is an increase in mean arterial pressure (MAP) that may be related to changes in SVR associated with increasing blood viscosity and via the removal of vasoactive substances.314,318 The obvious benefit of MUF is the removal of plasma water and the concentration of cellular and acellular elements of blood. However, a number of investigators have shown that UF reduces the production of other potential harmful substances including cytokines and endogenous pyrogens.316,319 This reduction in number is independent of the effects of hemoconcentration and is instead related to a reduction in the whole-body inflammatory response associated with CPB.316 The benefits of MUF are accentuated when aggressive UF is utilized during the rewarming period of CPB. This is more than likely a result of the removal of the activated complement fragment C3a, which is easily sieved in the ultrafiltrate.316 Patients treated with MUF have been shown to have a significantly faster rate of pulmonary function recovery than nonultrafiltrated patients, which may be because of a leukocyte stability and a reduced degranulation of polymorphonuclear neutrophils in the pulmonary capillaries.316,319